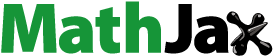
Abstract
Chemotherapy is ineffective for treating malignant glioma (MG) because of the low therapeutic levels of pharmaceuticals in tumour tissues and the well-known tumour resistance. The resistance to alkylators is modulated by the DNA repair protein O6-alkylguanine-DNA alkyltransferase (AGT). O6-benzylguanine (O6-BG) can irreversibly inactivate AGT by competing with O6-methylguanine and has been confirmed to increase the therapeutic activity of alkylators. We developed hybrid-structured poly[(d,l)-lactide-co-glycolide] nanofibrous membranes (HSNMs) that enable the sequential and sustained release of O6-BG and two alkylators (carmustine and temozolomide [TMZ]). HSNMs were surgically instilled into the cerebral cavity of pathogen-free rats and F98 glioma-bearing rats. The release behaviours of loaded drugs were quantified by using high-performance liquid chromatography. The treatment results were compared with the rats treated with intraperitoneal injection of O6-BG combined with surgical implantation of carmustine wafer and oral TMZ. The HSNMs revealed a sequential drug release behaviour with the elution of high drug concentrations of O6-BG in the early phase, followed by high levels of two alkylators. All drug concentrations remained high for over 14 weeks. Tumour growth was slower and the mean survival time was significantly prolonged in the HSNM-treated group. Biodegradable HSNMs can enhance therapeutic efficacy and prevent toxic systemic effects.
Introduction
Malignant glioma (MG) is one of the most common and treatment-resistant human tumours, with a median overall survival of 12–14 months [Citation1,Citation2]. Chemotherapy, radiotherapy and surgical excision are core components of the management of MG. The current standard treatment for patients with MG aged younger than 70 years is maximal surgical debulking followed by standard radiotherapy with concomitant and adjuvant chemotherapy with temozolomide (TMZ) [Citation3,Citation4]. The only two chemotherapeutics approved by the US Food and Drug Administration (FDA) for the treatment of MG, TMZ and carmustine (BCNU), both exert their therapeutic effect through alkylation [Citation5]. Unfortunately, the majority of patients with MG treated with alkylators demonstrate de novo or acquired resistance with subsequent tumour progression [Citation5]. The major cause of resistance to alkylating agents, such as BCNU and TMZ is O6-alkylguanine-DNA alkyltransferase (AGT), which is a DNA repair protein that removes and repairs O6-alkylguanine lesions initiated by alkylating agents [Citation6]. O6-benzylguanine (O6-BG) is an AGT substrate that inactivates AGT and enhances the cytotoxicity of alkylators both in vitro and in vivo [Citation6,Citation7].
Belonging to the class of nitrosoureas, BCNU alkylates the O6-guanine position in the DNA and cross-links the DNA, thereby inhibiting cancer growth [Citation8,Citation9]. Unfortunately, the efficacy of BCNU is limited by its associated side effects, including myelosuppression, hepatic toxicity and pulmonary fibrosis [Citation10,Citation11]. BCNU wafers (Gliadel® wafer; MGI Pharma, Inc., Bloomington, MN) are biodegradable polymers loaded with BCNU (polifeprosan 20 with BCNU) that are approved for treating newly diagnosed and recurrent MG. After a glioblastoma is surgically removed, the wafers are implanted in its cavity. As the polymer is degraded gradually, the wafers facilitate the delivery of high concentrations of BCNU to the surrounding tissues, with BCNU deposited up to 12 mm away from the implantation site in animal models [Citation12–14]. The safety and convenience of Gliadel wafers have been addressed for patients undergoing surgical resection of both recurrent and newly diagnosed MGs. Patients receiving Gliadel wafer treatment have reportedly survived approximately 2 months longer those who did not receive the treatment [Citation12,Citation15].
TMZ is an alkylating agent that induces DNA methylation of guanine at the O6 position. O6-methylguanine incorrectly pairs with thymine and triggers the mismatched system, leading to a double-strand break and the induction of apoptosis [Citation16,Citation17]. DNA alkylating agents are cytotoxic to cancer and normal cells, which leads to dose-limiting toxic effects, including myelosuppression [Citation18]. TMZ is an oral DNA alkylating agent with good penetration through the blood–brain barrier (BBB) and distribution in the central nervous system. Several studies have demonstrated the efficacy of TMZ in the treatment of high-grade gliomas, particularly in combination with radiotherapy [Citation3,Citation19].
Reportedly, approximately 50% of MGs overexpress AGT, as indicated by the hypomethylation of the promotor controlling AGT expression [Citation20,Citation21]. High-expression AGT tumour cells are resistant to TMZ owing to the ability of the catalytic AGT protein to repair TMZ-induced cytotoxic DNA damage [Citation2,Citation11,Citation22]. For patients with newly diagnosed MG, the median increase in survival for treatment with TMZ and radiotherapy is only 2.5 months compared with radiotherapy only. Current studies suggest that 60–75% of patients with MG derive no benefit from treatment with TMZ [Citation1,Citation20]. For recurrent MG, TMZ treatment failed in more than half of patients, with tumour recurrence within 6 months, indicating that TMZ is merely a less effective chemotherapy [Citation20,Citation23].
In this study, we embedded O6-BG into core–sheath-structured 50:50 poly[(d,l)-lactide-co-glycolide] (PLGA) nanofibrous membranes by employing coaxial electrospinning techniques and loaded the two different alkylators (BCNU and TMZ) into a blended 75:25 PLGA nanofibrous membrane through regular electrospinning. Hybrid-structured nanofibrous membranes (HSNMs) were thus obtained. The in vitro release profiles of the biopharmaceuticals were assessed using an elution method and a high-performance liquid chromatography (HPLC) assay. The HSNMs were surgically implanted into the brain parenchyma of pathogen-free rats after craniectomy. The in vivo release characteristics of O6-BG, TMZ and BCNU from the HSNMs were explored. Thereafter, the HSNMs were surgically implanted into the brain surface of tumour-bearing rats. The survival time and tumour volumes were compared with the tumour-bearing rats that treated with intraperitoneal injection of O6-BG combined with surgical implantation of gliadel and oral TMZ.
Methods and materials
Nanofibrous membrane fabrication
The commercial poly(lactide-co-glycolide) polymers Resomer® RG503 (lactide:glycolide, 50:50, 33,000 Da) and RG 756 (lactide:glycolide, 75:25, 70,000 Da) were obtained from Boehringer Ingelheim (Ingelheim am Rhein, Germany). O6-BG, BCNU and TMZ were purchased from Sigma-Aldrich (Steinheim, Germany). Gliadel wafer was purchased from MGI Pharma, Inc. (Bloomington, MN).
Two different structured nanofibres were prepared: core-sheath O6-BG/50:50 PLGA nanofibres and blended alkylating agents (TMZ and BCNU)/75:25 PLGA nanofibres. Core-sheath nanofibres were fabricated using a coaxial spinning device that transports two solutions simultaneously [Citation24]. Preset percentages of 50:50 PLGA (240 mg) were dissolved in 1 mL of hexafluoroisopropanol (HFIP; Sigma-Aldrich, St. Louis, MO) and 10 mg of O6-BG was dissolved in 1 mL of methanol. The 50:50 PLGA and O6-BG solutions were then instilled into two individual syringes for coaxial electrospinning. During spinning, the solutions were conveyed by two individually controlled pumps with flow rates of 0.3 and 0.9 mL/h for the core O6-BG solution and the sheath PLGA solution, respectively. The solutions in the syringes were applied with a positive voltage of 15 kV, and the travel distance between the needle tip and the collector, an aluminium sheet, was 15 cm.
Thereafter, the 75:25 PLGA (250 mg) and alkylators (20 mg TMZ and 20 mg BCNU) were dissolved in 1 mL of HFIP. The solution was then delivered and electrospun at room temperature by using a syringe pump at a volumetric flow rate of 1.8 mL/h. The electrospun nanofibres were collected in a nonwoven form on the same aluminium sheet. Hybrid bi-layered nanofibrous membranes with two different structures, namely core-sheath and blend nanofibres, were obtained. The thickness of the spun HSNMs was 0.12 mm, with each layer (core-sheath structured or blended matrix) of approximately 0.06 mm thick.
Microscopy examination
The electrospun morphology of nanofibrous membranes was analysed using a JEOL Model JSM-7500 F field emission scanning microscope (SEM; JEOL, Tokyo, Japan). The average diameter was acquired by calculating the diameters of 50 randomly selected fibres on the SEM photomicrographs. The structure of electrospun core-sheath nanofibrous membranes was inspected using a JEM-2000EXII field emission transmission electron microscope (TEM; JEOL). To confirm the existence of protein in the spun nanofibres, core–sheath-structured nanofibrous membranes were coaxially electrospun with 50:50 PLGA as the sheath layer and recombinant enhanced green fluorescent protein (reGFP) at the core. Spun fibres were then observed using a Leica TCS SP8X laser-scanning confocal microscope (LSCM; Leica Microsystems, Wetzlar, Germany) with an excitation wavelength of 487 nm. The porosity (%) of the nanofibrous membranes was computed employing the expression:
where ρmembrane and ρpolymer represent the densities of the nanofibrous membrane and the polymer, respectively.
In vitro elution characteristics of O6-BG and chemotherapeutic agents
The release features of O6-BG, BCNU and TMZ from the HSNMs were quantified using an in vitro elution method. Samples (1 × 1 cm2) cut from the multidrug-loaded membranes were deposited in test tubes (n = 3) with 1 mL of phosphate-buffered saline (PBS). After incubation in an oven at 37 °C for 24 h, the solution was gathered and substituted with 1 mL of new PBS for the next 24-h period. The process was replicated daily for 8 weeks. Drug levels in the gathered solutions were assessed using a Hitachi L-2200 HPLC assay (Multisolvent Delivery System, Tokyo, Japan).
To quantify the levels of eluted pharmaceuticals, a Discovery Bio Wide Pore C18–5 4.6 cm ×150 mm, 5-µm HPLC column (Supelco Analytical, Bellefonte, PA) was used to segregate the chemotherapeutic compounds. For BCNU, purified water and methanol (85/15 vol/vol; Sigma-Aldrich, Steinheim, Germany) were used in the mobile phase, and a monitoring absorbance of 230 nm and a flow rate of 1.0 mL/min were adopted. To assess O6-BG, the mobile phase included methanol and 0.25 M (pH 7.0) in a volume ratio of 50:50. The monitoring absorbance was maintained at 289 nm, whereas the volumetric flow rate was 1.0 mL/min. To characterize TMZ, the mobile phase included 80% methanol and 0.5% acetic acid (80/20 vol/vol). The monitoring absorbance was maintained at 316 nm and the volumetric flow rate was set at 1.0 mL/min. All experiments were performed in triplicate.
In vivo elution characteristics of O6-BG and chemotherapeutic agents
Sixty pathogen-free male Fischer 344 rats weighing between 250 and 300 g were purchased from BioLASCO Taiwan Co., Ltd. (Taipei, Taiwan). All rats were quarantined in the centre for 7 d before use. All animal-related procedures were approved by the Taipei Medical University Institutional Animal Care and Use Committee (LAC-2015–0157) and care of the animals followed the guidelines of the Department of Health and Welfare, Taiwan.
Each rat was anesthetized with a combination of intraperitoneal injection of ketamine hydrochloride (60 mg/kg; Parke-Davis, Morris Plains, NJ) and halothane inhalation. The depth of anaesthesia was monitored with both tail pinch and corneal reflex tests. The rats were randomly divided into 10 groups (3 d and 1, 2, 3, 4, 6, 8, 10, 12 and 14 weeks) containing five to six rats each. An approximately, 1.0-cm scalp incision was performed after shaving and sterilizing the postorbital region. The scalp fascia and muscle of the rat were dissected with a scalpel, and a craniectomy (approximately 10 × 10 mm2) was performed using an electric burr (). After achieving adequate haemostasis, the HSNM embedded with O6-BG, BCNU and TMZ was surgically implanted onto the surface of the brain parenchyma (). The 10 × 10 mm2 HSNM contained approximately 0.125 mg of O6-BG, 0.384 mg of BCNU and 0.384 mg of Temozolomide. The incision wound was then closed with 3–0 nylon sutures. After recovery from anaesthesia, the animals were returned to the housing facility. Rats with intraoperative brain injury or infection were excluded from the study. On day 3 and at weeks 1, 2, 3, 4, 6, 8, 10 and 12, overdosed ketamine hydrochloride (over 120 mg/kg) was administered to the rats intraperitoneally. Blood samples (approximately 0.5 mL) were gathered using syringes through cardiac puncture. The ipsilateral brain tissue beneath the HSNM was surgically extracted. Brain tissue samples with dimensions of 8 × 8 mm2 and thicknesses of 8–10 mm were sliced into four layers (i.e. layers 1–4 from the surface beneath the membrane towards the centre of the brain, with a thickness of approximately 1.5–2 mm for each layer) by using a rodent brain slicer (Zivic Instruments, Pittsburgh, PA). Brain tissue samples of about 50 mg in weight were gathered from each sliced layer. All specimens (brain and different brain tissue layers) were collected at each time-point. The tissue samples were extracted through sonication for 20 s and then centrifuged. Moreover, plasma was gathered and maintained at −80 °C. Drug levels in the samples were evaluated using HPLC analysis. The brain tissue (or at least one rat) at each time point was extirpated for histological analysis. Samples were stained with haematoxylin and eosin (H&E) and glial fibrillary acidic protein (GFAP) and then examined using light microscopy.
Figure 1. Surgical procedure and gross wound appearance. (A) A craniectomy was performed, and adequate hemostasis was attained. (B) Hybrid-structured nanofibrous membranes were placed onto the brain cortex. (C1-4) The number at the lower left corner of each image indicates the number of weeks after the implantation of the poly[(D,L)-lactide-co-glycolide] (PLGA) nanofibrous membrane. The scalp wound and brain tissue were quite clear, and no infection (exudate, pus, or granulation) was observed. The biodegradable PLGA nanofibrous membrane degraded with time, with only a small amount of the residual PLGA nanofibers (indicated by the black arrow) by the end of the study (week 14).
![Figure 1. Surgical procedure and gross wound appearance. (A) A craniectomy was performed, and adequate hemostasis was attained. (B) Hybrid-structured nanofibrous membranes were placed onto the brain cortex. (C1-4) The number at the lower left corner of each image indicates the number of weeks after the implantation of the poly[(D,L)-lactide-co-glycolide] (PLGA) nanofibrous membrane. The scalp wound and brain tissue were quite clear, and no infection (exudate, pus, or granulation) was observed. The biodegradable PLGA nanofibrous membrane degraded with time, with only a small amount of the residual PLGA nanofibers (indicated by the black arrow) by the end of the study (week 14).](/cms/asset/3d83ca9e-af55-420b-9ad2-ad40e67d256a/ianb_a_1460374_f0001_c.jpg)
Tumour inoculation
The rat malignant glioma cell F98 (ATCC-CRL-2948) was purchased from American Type Culture Collection and cultured in Dulbecco’s Modified Essential Medium (DMEM; Wako, Osaka, Japan) supplement with 10% FBS. A craniectomy (about 10 × 10 mm) was performed using an electric burr after deep anaesthesia. The rat was secured in a stereotactic frame (Model SAS 64612; ASI Instruments, Warren, MI). The total forty rats were implanted with F98 MG cells. A culture medium of 10 μL containing 2–3 × 105 9 L or F98 cell was injected over 3 min via a 26-gauge needle inserted to a depth of 5 mm at the centre of the craniectomy. After tumour inoculation, the animals were allowed to recover from anaesthesia and were given free access to water and food.
MRI examination and therapeutic regimens
The gross wound appearance was observed daily and survival time was recorded. All brain MRI scans (coronal view) were acquired using 7-Tesla Biospec (Bruker, Ettlingen, Germany). Before the treatment implementation (approximately 14–16 d after F98 MG cell implantation), T1- and T2-weighted MRI images were acquired to confirm that the glioma models were successfully established and excluded epidural, subdural, and intracranial haemorrhage or abscess (infection). After the rat MG models were successfully created and then tumour-bearing rats were randomly subdivided into two groups. In the gliadel group, the O6-BG was intraperitoneally administrated in dosage 50 mg/kg 1–2 h before surgical implantation of Gliadel wafer. In gliadel group, the one-twenty gliadel wafer (0.384 mg) was implanted onto to brain surface in each rat. One week later, oral TMZ (Temodal® 100 mg) was administered orally at a dose of 200 mg/BSA (m2) once daily for the first 5 days followed by a 23-day treatment interruption (cycle 1). If the tumour-bearing rats survival more than 1 month, start cycle 2. In the HSNM group, the biodegradable HSNM (10 × 10 mm) covering the brain surface was implanted. T2 weighted images were obtained as a reference to define the tumour district 2, 4, 6, 10 and 14 weeks after treatment. The tumour volume was reconstructed and calculated using the open-source, FDA-approved Digital Imaging and Communication in Medicine image Osirix software (Pixmeo SARL, Bernex, Switzerland). The therapeutic efficacy was evaluated using the tumours’ response to the treatment as a complete response, partial response or poor response on the basis of the confirmatory MRIs performed after four or more weeks. A complete response was defined as no tumour progression (tumour volume always decreased with time); a partial response was defined as a temporary decrease followed by an increase in the tumour volume; and poor response was defined as a time-dependent increase in the tumour volume.
Statistical analysis
Experimental data were collected from the samples and recorded as mean ± standard deviation. Statistically significant differences were analysed through paired samples t tests using the Stata SE version 12.0 software (StataCorp, College Station, TX). Survival data were analysed employing the Kaplan–Meier method, with statistical significance determined to exploit the post-hoc log-rank test. A repeated measures mixed model was adopted for evaluating the effect of different treatments on the implanted tumour growth.
Results
Characterization of drug-eluting nanofibres
Core-sheath and blended HSNMs were successfully manufactured using the versatile coaxial electrospinning process. The typical SEM photomicrographs of electrospun core-sheath nanofibres and blended nanofibres under 8000 × magnification are illustrated in , respectively. The average diameters of core-sheath and blended drug-eluting nanofibres were measured and calculated to be 529 ± 177 and 676 ± 203 nm, respectively, on the basis of data derived from 50 randomly selected fibres on the photomicrographs. The porosities of both core-sheath and blended nanofibres were high (78.1 ± 3.2 and 76.0 ± 4.1%, respectively). Moreover, the TEM photomicrograph is shown in demonstrates the core-sheath morphology of spun nanofibres. Based on the TEM images, the thickness of the HSNMs shells was estimated to be 36 ± 12 nm. An LSCM was also employed to assess the protein distribution. The coaxially electrospun core-sheath structured fibres showed the string-like green signals of reGFP (), demonstrating a relatively uniform protein distribution within the core-sheath structured nanofibres and high protein activity after electrospinning.
Figure 2. Characteristics of nanofibrous membrane. The typical examples of the SEM photomicrographs demonstrate the successful electrospinning of core-sheath nanofibres (A) and blended nanofibres (B). (C) Transmission electron microscopy photomicrograph reveals the core-sheath structure of coaxial electrospun nanofibres. (D) Laser-scanning confocal microscopic image demonstrated the bioactivity of recombinant enhanced green fluorescent protein in core-sheath nanofibres. (Magnification, 20×).
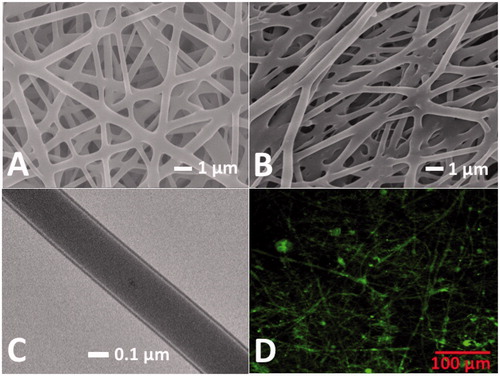
In vitro elution behaviours of biopharmaceutical agents
The in vitro elution curves of O6-BG, BCNU and TMZ from the HSNMs are shown in . O6-BG released greater drug levels during the first 4 weeks, after which the concentrations slowly decreased. The BCNU and TMZ release profile consisted of an initial slow-release lag phase in first 3 weeks, followed by a peak release phase from weeks 3 to 5 and a moderate-release phase starting in the fifth week. The accumulated elution curves of the HSNM are presented in . O6-BG was loaded onto core-sheath structured 50:50 PLGA nanofibres, leading to the sustained release of the biopharmaceuticals for 60 d, nearly half of which occurred during the first 3 weeks. BCNU and TMZ were loaded onto blended 75:25 PLGA nanofibres, yielding a slow degradation rate. The BCNU and TMZ released slowly initially, followed by accelerated release from days 21 to 35 and a moderate-release phase from days 35 to 60.
In vivo release characteristics
In vivo biopharmaceutical levels were assessed for 14 weeks using HPLC analysis. displays the drug elution profile of O6-BG in different layers of the brain and in plasma. The mean O6-BG level in the brain reached its maximal level (297.80 ± 51.85 μg/mL) on day 3 postsurgery and ranged from 52.52 ± 7.53 to 181.41 ± 67.58 μg/mL over the 14 weeks. The average O6-BG level value in plasma on day 3 postsurgery was 16.78 ± 3.03 μg/mL (maximum plasma concentration) and this had decreased gradually to 7.83 ± 1.37 μg/mL by the end of the study.
Figure 4. In vivo release. In vivo release of O6-benzylguanine (O6-BG) and alkylators from biodegradable hybrid-structured nanofibrous membranes (log10 scale). (A) O6-BG. (B) 1,3-Bis(2-chloroethyl)-1-nitrosourea (BCNU or carmustine). (C) Temozolomide. (Error bars represent standard deviations.) (D) The brain/blood concentration ratio of O6-BG, BCNU and temozolomide.
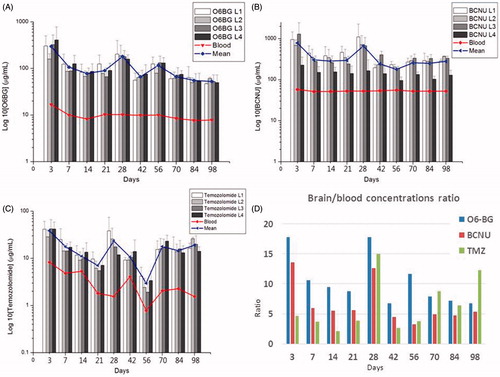
shows the in vivo release characteristics of BCNU. After the implantation of drug-eluting nanofibres, the BCNU concentration in the brain tissue swiftly reached its peak (785.90 ± 113.68 μg/mL) on day 3 postsurgery and remained at approximately 300 μg/mL during the first 3 weeks. The concentration then reached a second peak (662.52 ± 74.87 μg/mL) on day 28 postsurgery, followed by a slow decrease to 279.05 ± 31.73 μg/mL at 14 weeks. Drug concentrations in the plasma were relatively low (51.33 ± 1.14 to 57.72 ± 1.36 μg/mL). displays the release patterns of TMZ in the brain and plasma. The TMZ concentrations in the brain tissue (38.43 ± 11.34 μg/mL) peaked on day 3 postsurgery and ranged from 2.97 ± 0.96 to 23.28 ± 1.97 μg/mL for the 14-weeks study period. Again, the TMZ levels were markedly lower in the plasma (0.78 ± 0.13 to 8.27 ± 1.54 μg/mL). BCNU and TMZ were embedded onto 75:25 PLGA nanofibrous membranes, which feature a slower degradation rate than 50:50 PLGA nanofibres. The drug levels grew from week 3 and reached a peak during week 4. All drug levels (including O6-BG, BCNU and TMZ) were significantly higher in the brain (local) than in the plasma (systemic). The drug concentrations of biochemotherapeutic agents varied substantially between the brain and plasma at all-time points (p < .01). displays the brain/blood drug concentration ratios of O6-BG, BCNU and TMZ. The mean brain/blood drug concentration ratios were 10.46 (range, 3.22–13.61), 6.60 (range, 6.71–17.77) and 6.36 (range, 2.06–15.02) for O6-BG, BCNU and TMZ, respectively.
Histological examination of brain tissue
depicts the gross wound appearance at different time points. The operative wound and brain surface remained clear and no adhesion was observed. Furthermore, no gross exudate, pus or granulation was noticed. The biodegradable HSNM gradually degraded after implantation and very few remaining nanofibres were noted by week 14.
shows H&E-stained images of the brain tissues for weeks 2–14. No leukocyte migration to the brain tissue, necrotized tissue, thickening of the dura mater or other inflammatory response was noted. The cellularity of the brain decreased progressively (brain atrophy) at subsequent experimental stages. The estimated cell count was 434.80 ± 74.44 mm2 in week 2, which progressively had decreased to 196.80 ± 29.67 mm2 by the end of the study (week 14). The results of GFAP immunocytochemical examinations of the brain tissue are shown in . An increasing number of disrupted/destroyed glial cells and progressively decreased cellularity of the glial cells were noted.
Figure 5. Pathologic examination of brain tissue samples at different time points. (A1–A4) The number at the lower right corner of each image indicates the estimated number of cells. No inflammation (achieved through the increased movement of plasma and leukocytes, especially granulocytes, from the blood to the injured tissues), tissue necrosis or dura mater thickening was observed in the haematoxylin and eosin histological examination. Progressive brain atrophy (decreased cellularity) was noted in serial histological examinations (magnification, 40×). (B1–B4) Glial fibrillary acidic protein Immunocytochemical examination revealed progressive decrease in the cellularity and destruction of the glial cells at subsequent experimental stages (magnification, 100×). (A1, B1: 2 weeks; A2, B2: 6 weeks; A3, B3: 10 weeks; A4, B4: 14 weeks after the implantation of the hybrid-structured poly[(d,l)-lactide-co-glycolide] nanofibrous membranes).
![Figure 5. Pathologic examination of brain tissue samples at different time points. (A1–A4) The number at the lower right corner of each image indicates the estimated number of cells. No inflammation (achieved through the increased movement of plasma and leukocytes, especially granulocytes, from the blood to the injured tissues), tissue necrosis or dura mater thickening was observed in the haematoxylin and eosin histological examination. Progressive brain atrophy (decreased cellularity) was noted in serial histological examinations (magnification, 40×). (B1–B4) Glial fibrillary acidic protein Immunocytochemical examination revealed progressive decrease in the cellularity and destruction of the glial cells at subsequent experimental stages (magnification, 100×). (A1, B1: 2 weeks; A2, B2: 6 weeks; A3, B3: 10 weeks; A4, B4: 14 weeks after the implantation of the hybrid-structured poly[(d,l)-lactide-co-glycolide] nanofibrous membranes).](/cms/asset/1ba0b270-9d50-43c5-a057-4e967500616d/ianb_a_1460374_f0005_c.jpg)
Survival analysis
The rats that died during the perioperative period developed wound infection or failed to develop glioma were excluded. A total of 35 MG models were successfully created, 17 and 18 rats were enrolled in the gliadel and HSNM groups, respectively. The median survival time was 61.41 ± 22.62 d in the gliadel group, whereas the median survival time was 87.16 ± 17.00 d in the HSNM group. The Kaplan–Meier survival curves in demonstrated that the survival period was significantly shorter in the gliadel group than that in the HSNM group (p < .01).
Figure 6. Survival analysis and tumour volumes. (A) The survival rates for the gliadel and HSNM groups were analysed using Kaplan–Meier method. The overall survival rate was significantly higher in the HSNM group, p < .001. (B) The tumour volume change in the two groups was evaluated by adopting a repeated measured mixed model. The tumour volumes in the gliadel group rapidly increased and were significantly higher than those in the HSNM group (p < .01).
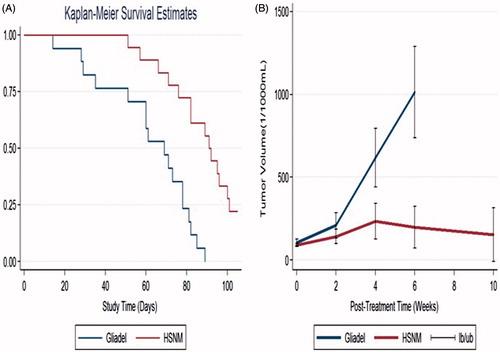
MRI and tumour volumes
The mean tumour volumes before treatment (approximately 14–16 d after tumour cell inoculation) were 105.92 ± 36.94 × 10−3 and 90.86 ± 17.08 × 10−3 mL, respectively. The initial mean tumour volumes were slightly lower in the HSNM group; however, the difference was not statistically significant (p = .18). The tumour volumes rapidly increased in the gliadel group, with tumour volumes of 209.68 ± 133.47 × 10−3, 617.02 ± 247.59 × 10−3 and 1014.44 ± 221.60 × 10−3 mL at 2, 4 and 6 weeks, respectively. The tumour volumes raised slower in the HSNM group, with a mean tumour volume 142.75 ± 84.89 × 10−3, 233.95 ± 195.43 ×10−3, 199.45 ± 198.60 × 10−3 and 153.89 ± 211.38 × 10−3 mL at 2, 4, 6 and 10 weeks, respectively. The repeated measures mixed model analysis of tumour volumes in the gliadel and HSNM groups is presented in . The tumour volumes rapidly increased in the gliadel group, whereas the volumes gradually increased, the difference of mean tumour volumes at 2nd and 4th week achieved statistical significance with p values .031, .002 and .005 at 2, 4 and 6 weeks. In the gliadel group, only one rat survival at post-treatment 10 weeks, thus the MRI data was not enrolled to compare with that in HSNM group. A high percentage (81.25%) of rats in the gliadel group manifested poor response, and only 18.75% manifested partial response, whereas no rat manifested complete response. Furthermore, approximately one-third rats (29.41%) in HSNM group expressed complete response, 23.53% expressed partial response, whereas less than half (47.06%) rats displayed poor response ().
Table 1. Responses of the rats to various treatments.
illustrates the serial brain MRI images in gliadel group; the implanted tumour rapidly grew and result in an intense mass effect, eventually causing the ray to die. displays the serial brain MRI scans of the F98 MG tumour-bearing rats in HSNM group. The initial tumour volume was similar to gliadel group, the tumour slowly grew and achieved the maximum tumour volume in week 4 and thereafter decreased steadily and no regrowth of tumour was observed.
Figure 7. Serial MRI images. The number in the upper right corner of each image indicated the number of weeks after the treatment. (A) The implanted glioma in most rats in the gliadel group rapidly increased and resulted in a severe mass effect and death. (B) The rat in the HSNM group expressed a good response to HSNM treatment. The tumour volumes clearly decreased with time, and nearly disappeared at end of the study.
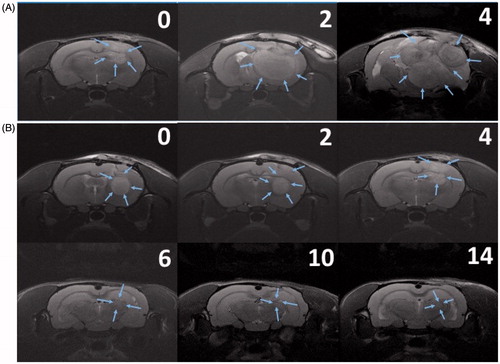
Discussion
MG arises from glial cells, which offer physical and chemical support for neurons and constitute approximately 90% of all brain cells. Glioma cells invasively infiltrate healthy brain tissues and cause great disarray in surrounding brain tissues, requiring tumour resection through surgical excision and invariably leading to death [Citation25,Citation26]. The BBB, the protective environment of the CNS, also restricts the transport of chemotherapeutic agents inside the brain tumour. Even with systemic levels in the toxic range, the drug concentration in the brain remains below the therapeutic level. To achieve effective local drug delivery with minimal side effects, different novel methods have been introduced [Citation27,Citation28], including the administration of biopharmaceuticals via intracranially implanted catheters and convection-enhanced drug delivery, as well as administration through controlled-release polymers. The only one of these new agents that has been approved by the FDA to treat MG is the 1,3-bis(2-chloroethyl)-1-nitrosourea (BCNU or carmustine) implant marketed as the Gliadel® wafer. Preclinical results suggest that the interstitial delivery of BCNU results in superior survival compared with systemic administration in gliosarcoma models [Citation29–31]. Although the BBB limits the intracerebral delivery of chemotherapies, resistance to chemotherapy is the major challenge leading to the failure to cure patients with a diverse spectrum of malignancies [Citation5,Citation23]. Previous preclinical trials conducted predominantly but not exclusively for non-CNS tumours have demonstrated that resistance to TMZ is mediated partly through the DNA repair protein AGT [Citation6,Citation7]. AGT removes chloromethylation or methylation damage from the O6 position of DNA guanines before cell injury and cell death. Certainly, AGT is not the only mechanism of resistance to the alkylators in MGs, but it is definitely a highly important one [Citation5,Citation32].
In vitro and in vivo studies using tumour cell lines and subcutaneous brain tumour xenografts have demonstrated that O6-BG enhances the therapeutic efficacy of alkylating agents [Citation7,Citation33–35]. The results from phase I and II trials have demonstrated that O6-BG, when added to TMZ [Citation5,Citation6] or BCNU (Gliadel wafer) [Citation22,Citation36–38], may circumvent tumour cell resistance and restore TMZ or BCNU sensitivity. Several strategies are currently being pursued to improve the therapeutic efficacy of alkylators by inhibiting AGT to reduce chemoresistance and improve overall survival. AGT may be a promising target for the treatment of alkylator-resistant gliomas [Citation2,Citation22,Citation37,Citation38].
Although the systemic administration of O6-BG alone is safe in animals and humans, it can cause a significant increase in toxicity and adverse effects when provided as a pretreatment before systemic BCNU or TMZ therapy [Citation11]. When both O6-BG and BCNU are administered systemically, O6-BG not only promotes the activity of BCNU but also enhances the hematopoietic toxicity of BCNU [Citation5]. Early-phase studies have revealed that O6-BG/TMZ administration causes severe off-target myelosuppression. In particular, patients administered TMZ at the maximum tolerated dose (472 mg/m2) in combination with O6-BG exhibited a 46% incidence of grade 4 neutropenia [Citation6,Citation39]. A key consideration is that the maximum effective alkylator dose used in combination with O6-BG is limited by systemic toxicities. The strategy of avoiding systemic toxicity by using a local delivery system was studied with promising results. To attenuate the increased systemic toxicity caused by O6-methylguanine-DNA methyltransferase inhibitors, Koch et al. [Citation40] locally administrated O6-BG through an Ommaya reservoir in combination with concomitant oral TMZ treatment. Although evidence that local O6-BG administration causes AGT to diminish in a residual tumour remains lacking, the trial conducted by Koch et al. demonstrated that intracerebral O6-BG treatment used concomitantly with TMZ (and presumably also other alkylating agents) might be a safe strategy for improving glioma therapy [Citation40]. In a study of nitrosourea-resistant intracranial human glioma xenografts in athymic rats, Kurpad et al. [Citation35] delivered O6-BG intra-arterially, yielding rapid AGT depletion and selective uptake into intracranial tumours and offering significant potential for the regional chemomodulation of AGT-mediated nitrosourea resistance in malignant human gliomas with concomitant reduction of systemic toxicity. In a phase I trial, Weingart et al. [Citation37] showed that systemic O6-BG can be administered concurrently with the intracranial implantation of BCNU wafers without added toxicity. In a phase II trial, Quinn et al. [Citation22] showed that the efficacy of implanted Gliadel wafers may be improved with the addition of O6-BG. Rhines et al. [Citation11] also concluded on the basis of their clinical trial that O6-BG can potentiate the effects of interstitially delivered BCNU and, for tumours expressing significant AGT, may be necessary for the BCNU to provide a meaningful therapeutic benefit.
Polymeric nanoparticles have been shown to be promising carriers for CNS drug delivery owing to their potential both in encapsulating drugs, hence protecting them from excretion and metabolism and in delivering active agents across the BBB without interfering with the normal function of the brain [Citation41–44]. Recent studies have suggested that the size of the delivery vehicle is one of the pivotal aspects determining performance because it influences biodistribution, cellular uptake and circulatory half-life. Smaller-sized fibres, particularly those sized approximately 100 nm, are likely to be advantageous because they are taken up by cells at rates 15–250 times faster than those of microsized fibres [Citation45,Citation46].
For a water-soluble drug in a hydrophobic polymeric matrix, the release mechanisms are controlled by channel diffusion, osmotic pressure and polymer degradation [Citation47]. First, most of the pharmaceuticals were dispersed in the bulk of the PLGA matrix following the electrospinning process. However, some drug might be located on the surfaces of the nanofibres, leading to the initial burst of BCNU and TMZ by channel diffusion. Second, if the polymer matrix surrounding the isolated drugs remains intact during the release, the pharmaceuticals will not be released from the nanofibres. However, water will be taken up by a water-soluble drug with high osmotic pressure through the polymer, causing swelling of the fibres. The polymer matrix may break under this swelling to form openings for drug release. Meanwhile, varying the ratio of lactic acid to glycolic acid monomers results in PLGA polymers with varying delivery rates [Citation48,Citation49]. The methyl side group in PLA makes it more hydrophobic than PGA; lactide-rich PLGA copolymers thus degrade more slowly. The only exception is 50:50 PLGA, which exhibits the fastest hydrolysis rate [Citation48,Citation50]. Since 50:50 PLGA possesses a less molecular weight, nanofibres made of 50:50 PLGA are expected to degrade faster and release the drugs at a higher rate than those of 75:25 PLGA. This might explain why O6-BG (encapsulated by 50:50 PLGA) was released at greater drug levels during the first 10 d. On the other hand, BCNU was released faster than TMZ. This can be attributable to the fact that BCNU is more hydrophilic than TMZ, thus resulting in higher elution rate. Finally, when polymer molecular weight decreases sufficiently, polymer loss begins. The drugs will then be released along with this polymer loss, leading to the gradual diminish of the drugs after 8 weeks.
O6-BG is a synthetic derivative of guanine and can easily be denatured by the application of a stress or compound, such as heat, organic solvent, strong acid or base or concentrated inorganic salt. In this study, we loaded O6-BG with 50:50 PLGA core-sheath structured nanofibres through coaxial electrospinning techniques. The LSCM image of reGFP demonstrated O6-BG retention at the core of electrospun core-sheath nanofibres with good bioactivity. The in vitro and in vivo studies revealed that O6-BG was quickly released from the hybrid-structured nanofibrous membrane, whereas TMZ and BCNU are quickly released after 3 weeks. The release of O6-BG prior to alkylators can provide advantages by suppressing tumour AGT activity, restoring tumour sensitivity and maximizing potential therapeutic benefit.
Theoretically, higher drug concentrations possess more efficient therapeutic effects and prevent tumour resistance. In vitro studies have shown that BCNU-resistant human glioma cells are effectively killed at a BCNU concentration of 250 μM (53.75 μg/mL) [Citation51]. The HSNMs developed in this study released concentrations of BCNU higher than 200 μg/mL for more than 14 weeks. Furthermore, the in vivo mean brain/blood drug concentration ratios were 10.46 (range, 3.22–13.61), 6.60 (range, 6.71–17.77) and 6.36 (range, 2.06–15.02) for O6-BG, BCNU and TMZ, respectively. The high brain/blood drug concentration ratios demonstrated minimum systemic side effects caused by chemotherapy, particularly in the combined administration of multiple biopharmaceuticals. Finally, the gross appearance showed clear wounds without granulation, scar tissue formation or abnormal discharge. No inflammation or infection reaction was noted in the H&E staining. The HSNM releases high concentration of O6-BG in the first 2 weeks followed by the high concentration of BCNU and TMZ for more than 14 weeks. While the HSNM was implanted onto brain surface of F98 tumour-bearing rat, the therapeutic efficacy (retarded tumour growth and longer survival time) was better than the rats treated with intraperitoneal injection of O6-BG combined with surgical implantation of gliadel wafer followed by oral TMZ regimen. The sequentially and continuously release O6-BG, BCNU and TMZ enhance the therapeutic efficacy of alkylators-based chemotherapy. Electrospun biodegradable PLGA HSNMs, after being applied to F98 tumour-bearing rats, demonstrated their ability to serve as an ideal vehicle for the local delivery of protein and chemical compounds to the brain; they can potentially enhance the therapeutic efficacy of MG while minimizing the toxic effects of systemic administration.
Acknowledgements
We thank the staff at the 7 T animal MRI Core Lab of Neurobiology and Cognitive Science Center, National Taiwan University for their technical and facility support.
Disclosure statement
The authors declare no conflict of interest.
Additional information
Funding
References
- Tanaka S, Louis DN, Curry WT, et al. Diagnostic and therapeutic avenues for glioblastoma: no longer a dead end? Nat Rev Clin Oncol. 2013;10:14–26.
- Adair JE, Johnston SK, Mrugala MM, et al. Gene therapy enhances chemotherapy tolerance and efficacy in glioblastoma patients. J Clin Invest. 2014;124:4082–4092.
- Stupp R, Mason WP, van den Bent MJ, et al. Radiotherapy plus concomitant and adjuvant temozolomide for glioblastoma. N Engl J Med. 2005;352:987–996.
- Stupp R, Hegi ME, Gilbert MR, et al. Chemoradiotherapy in malignant glioma: standard of care and future directions. J Clin Oncol. 2007;25:4127–4136.
- Quinn JA, Desjardins A, Weingart J, et al. Phase I trial of temozolomide plus O6-benzylguanine for patients with recurrent or progressive malignant glioma. J Clin Oncol. 2005;23:7178–7187.
- Quinn JA, Jiang SX, Reardon DA, et al. Phase II trial of temozolomide plus O6-benzylguanine in adults with recurrent, temozolomide-resistant malignant glioma. J Clin Oncol. 2009;27:1262–1267.
- Dolan ME, Mitchell RB, Mummert C, et al. Effect of O6-benzylguanine analogues on sensitivity of human tumor cells to the cytotoxic effects of alkylating agents. Cancer Res. 1991;51:3367–3372.
- Bacolod MD, Johnson SP, Ali-Osman F, et al. Mechanisms of resistance to 1,3-bis(2-chloroethyl)-1-nitrosourea in human medulloblastoma and rhabdomyosarcoma. Mol Cancer Ther. 2002;1:727–736.
- Brandes AA, Tosoni A, Basso U, et al. Second-line chemotherapy with irinotecan plus carmustine in glioblastoma recurrent or progressive after first-line temozolomide chemotherapy: a phase II study of the Gruppo Italiano Cooperativo di Neuro-Oncologia (GICNO). J Clin Oncol. 2004;22:4779–4786.
- Kornblith PL, Walker M. Chemotherapy for malignant gliomas. J Neurosurg. 1988;68:1–17.
- Rhines LD, Sampath P, Dolan ME, et al. O6-benzylguanine potentiates the antitumor effect of locally delivered carmustine against an intracranial rat glioma. Cancer Res. 2000;60:6307–6310.
- Brem H, Piantadosi S, Burger PC, et al. Placebo-controlled trial of safety and efficacy of intraoperative controlled delivery by biodegradable polymers of chemotherapy for recurrent gliomas. The Polymer-brain Tumor Treatment Group. Lancet. 1995;345:1008–1012.
- Tseng YY, Wang YC, Su CH, et al. Concurrent delivery of carmustine, irinotecan, and cisplatin to the cerebral cavity using biodegradable nanofibers: in vitro and in vivo studies. Colloids Surf B Biointerfaces. 2015;134:254–261.
- Grossman SA, Reinhard C, Colvin OM, et al. The intracerebral distribution of BCNU delivered by surgically implanted biodegradable polymers. J Neurosurg. 1992;76:640–647.
- Westphal M, Hilt DC, Bortey E, et al. A phase 3 trial of local chemotherapy with biodegradable carmustine (BCNU) wafers (Gliadel wafers) in patients with primary malignant glioma. Neuro Oncol. 2003;5:79–88.
- Trivedi RN, Almeida KH, Fornsaglio JL, et al. The role of base excision repair in the sensitivity and resistance to temozolomide-mediated cell death. Cancer Res. 2005;65:6394–6400.
- Kohsaka S, Wang L, Yachi K, et al. STAT3 inhibition overcomes temozolomide resistance in glioblastoma by downregulating MGMT expression. Mol Cancer Ther. 2012;11:1289–1299.
- Cai S, Xu Y, Cooper RJ, et al. Mitochondrial targeting of human O6-methylguanine DNA methyltransferase protects against cell killing by chemotherapeutic alkylating agents. Cancer Res. 2005;65:3319–3327.
- Vredenburgh JJ, Desjardins A, Reardon DA, et al. Experience with irinotecan for the treatment of malignant glioma. Neuro-oncology. 2009;11:80–91.
- Chamberlain MC. Temozolomide: therapeutic limitations in the treatment of adult high-grade gliomas. Expert Rev Neurother. 2010;10:1537–1544.
- Kitange GJ, Carlson BL, Schroeder MA, et al. Induction of MGMT expression is associated with temozolomide resistance in glioblastoma xenografts. Neuro Oncol. 2009;11:281–291.
- Quinn JA, Jiang SX, Carter J, et al. Phase II trial of Gliadel plus O6-benzylguanine in adults with recurrent glioblastoma multiforme. Clin Cancer Res. 2009;15:1064–1068.
- Affronti ML, Heery CR, Herndon JE, 2nd, et al. Overall survival of newly diagnosed glioblastoma patients receiving carmustine wafers followed by radiation and concurrent temozolomide plus rotational multiagent chemotherapy. Cancer. 2009;115:3501–3511.
- Hsu YH, Lin CT, Yu YH, et al. Dual delivery of active antibactericidal agents and bone morphogenetic protein at sustainable high concentrations using biodegradable sheath-core-structured drug-eluting nanofibers. Int J Nanomedicine. 2016;11:3927–3937.
- Wick W, Wick A, Weiler M, et al. Patterns of progression in malignant glioma following anti-VEGF therapy: perceptions and evidence. Curr Neurol Neurosci Rep. 2011;11:305–312.
- Lopez KA, Tannenbaum AM, Assanah MC, et al. Convection-enhanced delivery of topotecan into a PDGF-driven model of glioblastoma prolongs survival and ablates both tumor-initiating cells and recruited glial progenitors. Cancer Res. 2011;71:3963–3971.
- Tseng YY, Su CH, Yang ST, et al. Advanced interstitial chemotherapy combined with targeted treatment of malignant glioma in rats by using drug-loaded nanofibrous membranes. Oncotarget. 2016;7:59902–59916.
- Aberoumandi SM, Mohammadhosseini M, Abasi E, et al. An update on applications of nanostructured drug delivery systems in cancer therapy: a review. Artif Cells Nanomed Biotechnol. 2017;45:1–11.
- Tamargo RJ, Myseros JS, Epstein JI, et al. Interstitial chemotherapy of the 9L gliosarcoma: controlled release polymers for drug delivery in the brain. Cancer Res. 1993;53:329–333.
- Bota DA, Desjardins A, Quinn JA, et al. Interstitial chemotherapy with biodegradable BCNU (Gliadel) wafers in the treatment of malignant gliomas. Ther Clin Risk Manag. 2007;3:707–715.
- Fakhoury M. Drug delivery approaches for the treatment of glioblastoma multiforme. Artif Cells Nanomed Biotechnol. 2016;44:1365–1373.
- Bocangel DB, Finkelstein S, Schold SC, et al. Multifaceted resistance of gliomas to temozolomide. Clin Cancer Res. 2002;8:2725–2734.
- Bobola MS, Tseng SH, Blank A, et al. Role of O6-methylguanine-DNA methyltransferase in resistance of human brain tumor cell lines to the clinically relevant methylating agents temozolomide and streptozotocin. Clin Cancer Res. 1996;2:735–741.
- Friedman HS, Dolan ME, Pegg AE, et al. Activity of temozolomide in the treatment of central nervous system tumor xenografts. Cancer Res. 1995;55:2853–2857.
- Kurpad SN, Dolan ME, McLendon RE, et al. Intraarterial O6-benzylguanine enables the specific therapy of nitrosourea-resistant intracranial human glioma xenografts in athymic rats with 1,3-bis(2-chloroethyl)-1-nitrosourea. Cancer Chemother Pharmacol. 1997;39:307–316.
- Friedman HS, Pluda J, Quinn JA, et al. Phase I trial of carmustine plus O6-benzylguanine for patients with recurrent or progressive malignant glioma. J Clin Oncol. 2000;18:3522–3528.
- Weingart J, Grossman SA, Carson KA, et al. Phase I trial of polifeprosan 20 with carmustine implant plus continuous infusion of intravenous O6-benzylguanine in adults with recurrent malignant glioma: new approaches to brain tumor therapy CNS consortium trial. J Clin Oncol. 2007;25:399–404.
- Quinn JA, Pluda J, Dolan ME, et al. Phase II trial of carmustine plus O(6)-benzylguanine for patients with nitrosourea-resistant recurrent or progressive malignant glioma. J Clin Oncol. 2002;20:2277–2283.
- Quinn JA, Jiang SX, Reardon DA, et al. Phase I trial of temozolomide plus O6-benzylguanine 5-day regimen with recurrent malignant glioma. Neuro Oncol. 2009;11:556–561.
- Koch D, Hundsberger T, Boor S, et al. Local intracerebral administration of O(6)-benzylguanine combined with systemic chemotherapy with temozolomide of a patient suffering from a recurrent glioblastoma. J Neurooncol. 2007;82:85–89.
- Tosi G, Costantino L, Ruozi B, et al. Polymeric nanoparticles for the drug delivery to the central nervous system. Expert Opin Drug Deliv. 2008;5:155–174.
- Barbu E, Molnar E, Tsibouklis J, et al. The potential for nanoparticle-based drug delivery to the brain: overcoming the blood-brain barrier. Expert Opin Drug Deliv. 2009;6:553–565.
- Chen H, Nan W, Wei X, et al. Toxicity, pharmacokinetics, and in vivo efficacy of biotinylated chitosan surface-modified PLGA nanoparticles for tumor therapy. Artif Cells Nanomed Biotechnol. 2017;45:1–8.
- Afsharzadeh M, Hashemi M, Mokhtarzadeh A, et al. Recent advances in co-delivery systems based on polymeric nanoparticle for cancer treatment. Artif Cells Nanomed Biotechnol. 2017 [cited 2017 Sep 28]; [16 p.]. DOI:10.1080/21691401.2017.1376675
- Decuzzi P, Pasqualini R, Arap W, et al. Intravascular delivery of particulate systems: does geometry really matter? Pharm Res. 2009;26:235–243.
- Tseng YY, Liao JY, Chen WA, et al. Sustainable release of carmustine from biodegradable poly[((D,L))-lactide-co-glycolide] nanofibrous membranes in the cerebral cavity: in vitro and in vivo studies. Expert Opin Drug Deliv. 2013;10:879–888.
- Yu M, Yao Q, Zhang Y, et al. Core/shell PLGA microspheres with controllable in vivo release profile via rational core phase design. Artif Cells Nanomed Biotechnol. 2018 [cited 2018 Feb 27]; [10 p.]. DOI:10.1080/21691401.2018.1443940
- Makadia HK, Siegel SJ. Poly Lactic-co-Glycolic Acid (PLGA) as biodegradable controlled drug delivery carrier. Polymers (Basel). 2011;3:1377–1397.
- Park TG. Degradation of poly(lactic-co-glycolic acid) microspheres: effect of copolymer composition. Biomaterials. 1995;16:1123–1130.
- Tseng YY, Yang TC, Wang YC, et al. Targeted concurrent and sequential delivery of chemotherapeutic and antiangiogenic agents to the brain by using drug-loaded nanofibrous membranes. Int J Nanomedicine. 2017;12:1265–76.
- Tseng YY, Huang YC, Yang TC, et al. Concurrent chemotherapy of malignant glioma in rats by using multidrug-loaded biodegradable nanofibrous membranes. Sci Rep. 2016;6:30630.