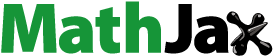
Abstract
N-[(2-Hydroxyl)-propyl-3-trimethyl ammonium] chitosan chloride (HTCC), a hydrosoluble chitosan derivative, has been extensively investigated as a class of drug delivery vehicles because of its unique features. However the studies on HTCC for pulmonary delivery systems have been rarely conducted. This study aimed to design porous microspheres (MS) containing cyclosporine A (CsA) using HTCC as the carrier. The physicochemical properties and biocompatibility of the MS were evaluated. The in vivo efficacy of MS was evaluated in an asthmatic rat model after pulmonary administration. The results showed that porous MS suitable for inhalation could be readily produced by spray drying method. Optimized porous MS in this study exhibited to be biocompatible and safe to use in the lung, and they were effective in suppression of inflammation in the asthmatic rat model. Above all, our results suggested that HTCC porous MS are promising drug carriers for pulmonary drug delivery.
Graphical Abstract
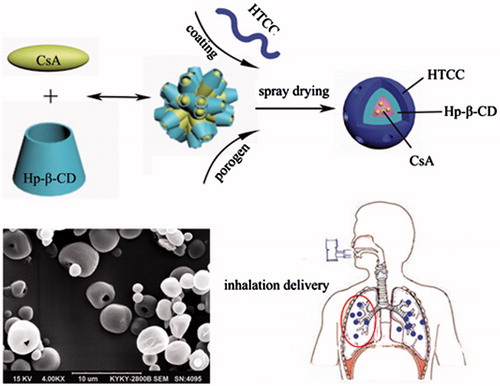
Introduction
Chitosan (CTS) is a cationic natural biomaterial with distinct advantages, such as biodegradability, low toxicity, and high biocompatibility [Citation1,Citation2]. Meanwhile, CTS can improve drug absorption by opening the intercellular tight junctions in the lung epithelium [Citation3]. Therefore, CTS has used to prepare the sustained release form of pulmonary delivery. Nevertheless, its applications are limited in terms of solubility because CTS loses its cationic nature above pH 6.5. Hence, the water solubility of CTS should be improved to broaden its applications. N-[(2-Hydroxyl)-propyl-3-trimethyl ammonium] chitosan chloride (HTCC) is a hydrosoluble CTS derivative. HTCC was obtained via the reaction between hydroxypropyl trimethyl ammonium chloride (EPTAC) and CTS [Citation4]. HTCC has also been extensively investigated as a class of drug delivery vehicles because of its unique features, including biocompatible material, antibacterial property, good water solubility in a wide range of pH, high drug-loading capacity, and the varying release rate by modulating the degree of substitution (DS) of HTCC [Citation5]. Moreover, HTCC is able to increase the paracellular permeability and/or absorption of peptide drugs across mucosal epithelia at neutral pH values as the positive surface charge of HTCC can bind to the negative-charged epithelial cell membranes by electrostatic interactions [Citation6]. Therefore, HTCC is a promising polymer as a basic material for mucosal drug delivery systems, such as pulmonary delivery.
Drug delivery by the pulmonary provides applicable features including local targeting, circumvention of first-pass hepatic metabolism and rapid onset of action. Based on these characteristics, numerous efforts have been made to design drug delivery by the pulmonary route for local or systemic effect [Citation7–9]. However, commercially available inhalable products are mainly rapid release formulations that require the patient to inhale several times every day, thus reducing patient compliance [Citation10]. For the existing problems, sustained release microspheres (MS) have been proposed for pulmonary delivery to reduce systemic toxicity and enhance therapeutic effectiveness in recent decades [Citation11–13]. The physical properties of particles are essential for an effective pulmonary drug delivery. An optimal aerodynamic diameter ranges from 1 to 5 μm [Citation14]. MS with an aerodynamic diameter larger than 5 μm are likely deposited in the upper airways. By contrast, those smaller than 1 μm are exhaled [Citation14]. Edwards studied innovatively that porous MS exhibit a higher respirable fraction than nonporous particles do, although these MS are characterized by identical physical diameters [Citation15]. Characterized by the larger surface-to-volume ratio, porous MS have been potentially applied as pulmonary drug delivery systems [Citation16,Citation17].
But at present, the research on HTCC as a drug delivery vehicle has focused mainly on films [Citation18], hydrogels [Citation19], and nanoparticles [Citation20], the studies on HTCC for pulmonary delivery systems have been rarely conducted. To address this challenge, in our study, HTCC was used as the basic excipient/carrier to prepare porous MS as pulmonary drug delivery systems with ammonium bicarbonate (ABC) as an effervescent porogen. Hydroxypropyl-β-cyclodextrin (HP-β-CD) could solubilize hydrophobic drugs such as cyclosporine A (CsA) [Citation21], so it was incorporated with HTCC. Techniques such as emulsion solvent evaporation, supercritical fluid technology and spray drying have been used to produce drug-loaded micro- and nanoparticles [Citation22–24]. Spray drying offers the advantage of encapsulation and drying in one continuous operation, which is significantly less time consuming than other methods. The hypothesis was that porous HTCC MS have physicochemical properties which were suitable for pulmonary drug delivery, and good biocompatibility and in vivo efficacy.
Materials and methods
Materials
CsA was supplied by Minsheng Pharmaceutical Factory (Hangzhou, China). HTCC was synthesized and characterized in accordance with a previously described process [Citation4,Citation5]. The molecular weights of HTCC were 1360 kDa, 450 kDa, and 170 cps with DS of 88.74, 83.60, and 73.95%, respectively. All other chemicals and reagents used in this study were of analytical grade, and purchased from Shanghai Chemical Reagent Company (Sigma Co., St. Louis, MO).
Animals
Male Sprague-Dawley rats weighing 280 ± 50 g were purchased from Jinan Lukang, Jinan, China. Toads (30–40 g, male and female) were provided by Weifang Medical University, Shandong, China. The animal protocol was approved by Shandong Medical Laboratorial Animal Administration Committee (Approval No. WFMU-IACUC-C2016-10-13-205). The rats were housed in a room with controlled temperature and humidity. All animal studies were carried out in accordance with the National Institutes of Health Guide for the Care and Use of Laboratory Animals (NIH publication no. 8023) revised in 1978.
Methods
Preparation of CsA/HTCC/Hp-β-CD porous MS
Predetermined amounts of Hp-β-CD and CsA were dissolved in 800 ml of 1% aqueous solution according to various formulations (). Then the solution was mixed well with certain amounts of HTCC and ABC, followed by filtering through a 0.45 μm micropore film. Then, the MS were prepared employing a spray drier (Buchi Mini Spray Dryer, B-290, Flawil, Switzerland) with operating parameters of inlet temperature as in , feed rate 4–6 mL/min, and the air-flow rate 600 L/h. The spray-dried MS were collected and stored in a desiccator at room temperature prior to analysis.
Table 1. Composition of formulations used for aqueous solution to be spray dried.
Physicochemical property
Microparticle morphology: The surface morphology of MS was examined by scanning electron microscopy (SEM) (KYKY 2800B, KYKY Technology Development Co., Ltd, Beijing, China).
Size of microparticles: The mean volume diameters (MVDs) of the microparticles were measured via dynamic light scattering (DLS) (Malvern MasterSizer, model MS 2000; Malvern Instruments, Ltd., Malvern, UK). Each sample was measured at least three times.
Fourier-transformation infrared spectroscopy (FT-IR) analysis: FT-IR analysis was carried out for pure CsA, HTCC, Hp-β-CD, blank microsphere MS D, MS A, MS B, MS C and MS I in the range of 4000–500 cm−1 using Avater-360 FT-IR spectroscope (Nicolet, Madison, WI).
Differential scanning calorimetry (DSC) analysis: The DSC of pure CsA, HTCC, Hp-β-CD, blank microsphere MS D, MS A, MS B, MS C, and MS I was characterized using DSC7020 differential thermal scanning analyser (Hitachi High-tech Science Corporation, Tokyo, Japan).
X-ray diffraction analysis: The X-ray diffraction (XRD) of pure CsA, HTCC, Hp-β-CD, blank microsphere MS D, MS A, MS B, MS C and MS I was characterized using Ultima IV (Rigaku Corporation, Tokyo, Japan) at 44 mA and 40 kV.
High-performance liquid chromatography (HPLC) method validation: The CsA concentration was determined using Agilent 1260 HPLC (Agilent Technologies Inc., Palo Alto, CA). The quantitative analysis of CsA was performed using C18 chromatographic column (250 × 4.6 mm, 5 μm) (Elite Company, Dalian, Liaoning Province, China) at 70 °C. The mobile phase consisted of acetonitrile, water in the ratio of 74:26, and the flow rate was 1 mL/min. The detection wavelength was 210 nm. The intra- and inter-day precision and accuracy were examined by analyzing CsA (5.8, 23.2 and 46.4 μg/mL) with five replicates on five separate days, and the precision are presented as the relative standard deviations (RSD).
Entrapment efficiency (EE) and drug-loading efficiency (DL): The CsA concentration was determined using the validated HPLC method, and EE and DL were calculated using EquationEquations (1)(1)
(1) and Equation(2)
(2)
(2) as below:
(1)
(1)
(2)
(2)
In vitro aerosol dispersion performance by the next-generation impactor (NGI): As previously reported [Citation25], the aerosol dispersion properties of the dry powder particles as dry powder inhalers (DPIs) were tested using the NGI with a stainless steel induction port (US Pharmacopeia throat) attachment (NGI model 170; MSP Corporation, Shoreview, MN) coupled with specialized stainless steel NGI gravimetric insert cups (MSP Corporation). The NGI was run at a controlled flow rate of Q = 60 L/min. At this rate the cutoff diameters for each impactor stage from one to seven were sequentially 8.06, 4.46, 2.82, 1.66, 0.94, 0.55 and 0.34 µm as calibrated and stated by the manufacturer. The mass median aerodynamic diameter (MMAD) and geometric standard deviation (GSD) were determined using a mathematic programme written by Dr Jay Holt [Citation26]. All experiments were triplicated (n = 3). The fine particle fraction (FPF), respirable fraction (RF), and emitted dose (ED) were calculated as follows in EquationEquations (3–5):
(3)
(3)
(4)
(4)
(5)
(5)
Biocompatibility
Assay for lactate dehydrogenase (LDH) and protein on the acute lung toxicity: Male Sprague-Dawley rats weighing 280 ± 50 g were divided into five groups (four rats in each group): normal group, phosphate-buffered solution (PBS) group (100 μL, pH = 7.4), positive control group (100 μL, 0.25% Triton X-100), MS group (20 mg), and HTCC group (10 mg). Dry powder of MS and HTCC was administered by an inhaler, and normal group did not be disposed. The activity of LDH in the supernate was measured using Full Automatic Biochemical Analyser 7080 (Hitachi Instrument Ltd., Tokyo, Japan). The protein content was determined using an assay kit of Coomasie Briliant Blue protein (Nanjing Jiancheng Bioengineering Institute, Nanjing, China). A sodium dodecylsulphate–polyacrylamide gel electrophoresis (SDS–PAGE) also operated to testify the protein content with operating parameters of 8% stack, 25 μL protein samples, and 200 V constant voltage.
Ciliotoxicity study: Ciliotoxicity studies were carried out using an in situ toad palate model. The upper palates of the toads (n = 6) were exposed and treated with HTCC (10 mg), CsA (0.4 mg), MS (20 mg) and 0.5 ml of normal saline, respectively. Thereafter, the test formulations were removed by washing the palate with saline. Approximately 3 × 3 mm of the palate was dissected and the mucocilia was examined instantly with an inverted microscope (Olympus CK2, Tokyo, Japan) at an enlargement of 40×, the lasting time of cilia movement was recorded every 1 h in the first 10 h, then every 20 min. The mucosa sections were kept in saline before the next observation. And 1 mm3 of mucocilia of each group was tested with transmission electron microscope. Both the morphology of mucocilia and the lasting time of cilia movement were considered as evaluation criteria of ciliotoxicity of formulations.
In vivo efficacy evaluation
Immunization and challenge: The rats were divided into four groups. Each group consisted of five rats. They were immunized on 1, 8 and 15 days by intraperitoneal injection of 80 μg chicken ovalbumin (OVA) emulsified in 1 mg aluminium hydroxide adjuvant in a total volume of 0.2 ml of saline. From 22 days, the rats were atomizing inhalation administered by 1% OVA in a total volume of 10 mL of saline two times a day and 7 days in a row. Budesonide (BUD) and MS I were dissolved in saline. Rats were atomization inhalation administered 10mg/kg/day (in 1 ml) of BUD and 120 mg/kg/day (in 1 ml) of MS I, respectively, each day from Days 22 to 29 consecutively. The control and OVA groups were administered saline.
Bronchoalveolar lavage fluid (BALF) collection and leukocyte count: Each rat was anaesthetized and the trachea was cannulated. BALF was collected by flushing 1 ml of PBS into the lung via the trachea immediately after sacrifice. Approximately 0.8 ml of BALF was recovered after five lavages. The BALF was centrifuged (1500 rpm/min, 5 °C, 10 min) and the supernatant was stored at −70 °C until measurement of cytokines. Cells from the BALF were washed five times with PBS and the pellet was resuspended in 1 ml of PBS. Total cell number was counted by cytospin and a differential cell count was performed after staining with Giemsa solution and each 200 cells were counted in each of four random locations.
Enzyme-linked immunosorbent assay (ELISA): The levels of cytokines in BALF or serum, interleukin-4 (IL-4) and tumor necrosis factor-α (TNF-α) were measured by sandwich ELISA from R&D Systems (Minneapolis, MN) according to the manufacturer’s instructions. The level of total protein and LDH in BALF were measured by total protein quantitative assay kit and Cytotoxicity Assay Kit from Shanghai Biotechnology Systems (Beyotime, Shanghai, China), respectively. Each concentration was calculated using a linear-regression equation obtained from the standard absorbance values.
Histopathological studies
Lung tissue was fixed with 10% (v/v) formaldehyde, then were dehydrated in various concentrations of ethanol and embedded in paraffin. For histopathological examination, 4 μm sections of fixed embedded tissues were cut on a microtome (Leica RM 2135 Rotary Microtome, Wetzlar, Germany), and placed on glass slides and stained with HE for general morphology. Pulmonary histopathological changes were assessed on five point scores by two independent examiners. The scores were: 0, no inflammatory cells; 1, minimalaccumulation of inflammatory cells; 2, moderate accumulation of inflammatorycells; 3, severe accumulation of inflammatory cells; and 4, extreme accumulation of inflammatory cells.
Statistical analysis
The results were reported as mean ± standard deviations. Statistical analysis was carried out using SPSS19.0 (Armonk, NY) with a two-tailed paired t-test and Duncan’s multiple range tests. And differences were considered to be significant at a level of p < .05.
Results and discussion
Physicochemical property
Morphological analysis
The morphological characteristics of MS prepared according to various formulations () were shown in . Three levels of CsA/HTCC/Hp-β-CD inclusion complexes without porogens (MS A, MS B, and MS C) were prepared. Blank microparticles were labelled MS D (). ABC [Citation17], poly(propylene glycol)-block-poly(ethylene glycol)-block-poly(propylene glycol) (F68) [Citation27], polyethylene glycol (PEG, Mn = 20,000) [Citation28], chloroform (Chl) [Citation29], and polystyrene (PS) MS [Citation30] were selected as porogens in this study. ABC was utilized as an effervescent porogen in the formulations of MS E, MS F, MS G, MS H, MS I, MS J, MS K, MS L, and MS M. The morphological characteristics of MS evidently differed when the formulations were varied. External porousness was observed on the surface of MS E with adding ABC, indicating ABC may be suitable as a porogen. When the ABC content was varied, particle diameter and porosity were changed significantly, as demonstrated by the different morphologies of MS F, MS G, and MS J. MS G showed compact and aggregated spherical particles and a slightly wrinkled surface. A similar morphology was observed in MS H, suggesting CsA slightly affected the morphological structure of the spray-dried MS. MS I was spherical with a smooth surface and porous structures. The porous structure may facilitate pulmonary delivery of CsA administered with fast adsorption and desorption because of their greater surface area than nonporous MS. MS J presented an irregular or sheet structure with compact aggregation. And this morphology was different from that of MS E whose formulation was the same as MS J, except for the molecular weight of HTCC, showing that varying molecular weight would result in different morphologies. When 170 cps HTCC was employed, MS K, MS L, and MS M showed spherical morphologies with smooth surface. No visible difference was observed on the surface of MS L and MS M, which indicated the content of HTCC could not exert an effect on the porosity within a certain range. MS K displayed smooth surface and pore structure with a spherical morphology, but the porosity was lower than that of MS I.
When F68 was used as a porogen as shown in , (N,O), HTCC/Hp-β-CD and CsA/HTCC/Hp-β-CD MS became regular spherical particulate without porousness. With the increase of F68, there was still no porousness in the MS P, of which the diameter became dramatically small when comparing with MS N and MS O. When PEG served as porogen, MS Q became compact and aggregated, and porousness was not observed at the surface of the microsphere aggregate. When Chl acted as porogen, MS R became an irregular spherical material with small pores, which was not appropriate for pulmonary drug delivery. Additionally, when PS MS served as porogen, MS S displayed irregular spherical with wrinkle-like features. With the removal of PS MS, no difference was observed in the appearance of MS T and MS S.
According to the morphology of all MS, MS I, which possessed a spherical morphology, a smooth surface and pore structures, was selected as the optimal formulation for the following investigation.
Characterization of particle size
The geometric diameter should be higher than 4 μm to prevent phagocyte clearance [Citation31]. As shown in , the MVDs of MS A, MS B, MS C and MS I measured using DLS were 2.95, 2.38, 2.17 and 3.54 μm, respectively. The MVD of porous MS I was significantly larger compared with MS B or MS C (p < .05). The geometric diameter of MS I was in size range of 1–7 μm in . The geometric diameter of fractional MS I was greater than 4 μm, indicating that could resist phagocytosis through macrophages.
Figure 2. The geometric diameter determined via dynamic light scattering. (A) The MVDs of different MS. Bars represent standard errors of three replications. Different letters indicate significant differences (p < .05) according to Duncan’s multiple range tests; (B) the size distribution by intensity of MS I.
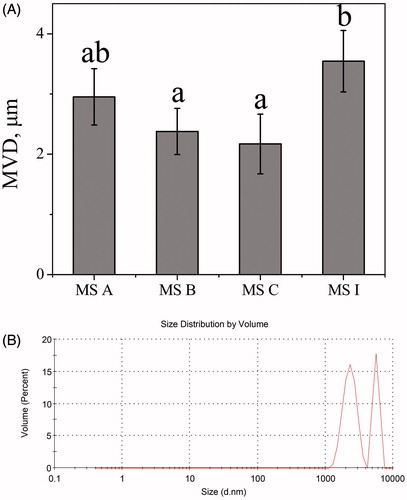
FT-IR analysis
The FT-IR spectra were shown in . The adsorption peak at 1595 cm−1 corresponding to the N-H deformation vibration of amino groups was absent in the HTCC spectrum, hence indicating that the primary amine has been changed to a quaternary ammonium group [Citation4]. The peak at 1480 cm−1 for HTCC spectrum could be assigned to the C–H bending of − N(CH3)3+. When comparing with the peaks of stretching vibration of the amide of HTCC and hydroxyl bond of Hp-β-CD, the peak observed at 3373 cm−1 in the spectrogram of MS D was shifted to a lower frequency by about 37 and 20 cm−1 respectively, which resulted from the formation of intermolecular hydrogen bonds between the stretching vibration of the amide of HTCC and hydroxyl bond of Hp-β-CD. However, the peak of MS B was shifted to 3386 cm−1 with the addition of CsA, which demonstrated that CsA weakened the hydrogen bonds between the two molecules. This change was in agreement with the result that in the CsA spectrogram, the high-intensity stretching vibration appearing at 1628 cm−1 was the characteristic absorption peak of the stretching vibration of one carbonyl, after complex formation, the absorption shifted to 1632, 1636, 1632, and 1639 cm−1 in the FT-IR spectra of MS A, MS B, MS C, and MS I, respectively, which indicated the existence of strong interactions between the C=O group of CsA and the hydrogen bond donor of MS D (blank MS). This could be explained by the previous studies that the CDs and CsA were able to self-assemble in aqueous solutions to form nano-sized aggregates and micellar-like structures [Citation32], and this force weakened the hydrogen bonds between Hp-β-CD and HTCC. The FT-IR spectra of MS A, MS B, and MS C showed that the hydrogen bonds were strengthened with the increasing proportion of HTCC, which demonstrating that different formulations should be considered on the ability to form hydrogen bonds. Comparing MS I with the above-mentioned drug-loading MS without porousness, the molecules and intermolecules forces between the three molecules were under the influence of ABC, which was manifested by the blue shifts of the peaks of the stretching vibration of one carbonyl and the intermolecular hydrogen bonds between the stretching vibration of the amide of HTCC and hydroxyl bond of Hp-β-CD.
DSC analysis
The DSC thermograms are shown in . Raw CsA was characterized by a glass transition temperature (Tg) at 136 °C, which had not melting point even upon heating to 200 °C. This result indicated the amorphous nature of the drug, which was in good agreement with previous reports on CsA [Citation33,Citation34]. Nevertheless, the Tg was absent in CsA-loaded MS, thereby indicating that the amorphous CsA was dispersed into the polymer matrix. One endothermic peak was observed for HTCC at 168 °C. A small endothermic peak was observed at 162 °C for Hp-β-CD. Only one small endothermic peak at 165 °C was observed in MS D, which suggested that chemical bond existed between the two material in line with FT-IR analysis. At 165 °C, the peak was again observed with the addition of CsA to HTCC/Hp-β-CD in MS B, showing that the encapsulation of ∼0.89% CsA did not render the thermal stability of MS in spite of the influence on hydrogen bond between HTCC and Hp-β-CD. This might be explained by the protective effect of the important adjuvant, HTCC, on the interior structure, which could be further demonstrated by the result that with the increase of HTCC content, the peaks of MS A and MS C were strengthened in intensity and shifted to the right in different extent comparing with MS B. The endothermic peak at 165 °C was also observed for MS I, and was approximate in intensity with MS A and MS C, which indicating that ABC did not affect the thermal stability of final MS, that was, the thermal stability of MS I was not lower than those of MS A and MS C.
XRD analysis
As shown in , the XRD pattern for CsA powder did not display the representative peaks of crystalline CsA as in the previous study [Citation35,Citation36], indicating that the CsA used in our study was predominantly in amorphous state. Compared with the CTS, which showed two diffraction peaks at 2θ = 11° and 2θ = 20° corresponding to the crystal forms I and II [Citation4], the only diffraction peak at 2θ = 20° of HTCC became lower and broader because of the introduced EPTAC, which hindered the reconstitution of inter or intramolecular hydrogen bonds of HTCC, indicating the decrease of crystalline [Citation37]. HP-β-CD showed an amorphous structure lacking crystalline peaks, but extremely small peaks at 2θ = 11°, 18° were observed. In the XRD pattern of MS D, only one diffraction peak at 2θ = 19° was broader and lower compared with the diffraction peaks at 2θ = 20° of HTCC and 2θ = 18° of HP-β-CD, which corresponded to the low crystalline degree based on the knowledge that the width of the XRD peak is related to the degree of crystallinity [Citation4]. The lower crystallite suggested that the intermolecular interaction between HP-β-CD and HTCC macromolecular chains destroyed the original crystalline structures. In the XRD pattern of MS A, MS B, MS C, and MS I, with the addition of CsA to HTCC/Hp-β-CD, the diffraction peak was still observed at 2θ = 19 ± 1° without any characteristic CsA peaks, which showed that CsA was entrapped. Moreover, ABC didn’t make a difference to the crystalline structure, which was consistent with the result of DSC analysis that thermostability of MS I was not altered during ABC addition.
HPLC validation
A calibration curve (R2 = 0.99997) was obtained with CsA standard solutions ranging from 5.8 to 46.4 μg/mL with a retention time of 5.266 min. As shown in , the RSD of intra-day precision were 1.49, 0.42 and 0.51% at the three different levels, respectively, and the RSD of their inter-day precision were 1.42, 0.36, and 0.48% respectively. The precision were all below 5%. The intra-day and inter-day accuracy ranged from 100.39 to 103.10% and 99.27 to 103.66%, respectively. Therefore, these data confirmed that the HPLC method was sufficiently sensitive and reproducible for detecting CsA.
Table 2. Accuracy and precision of the analyses of CsA (n = 5).
EE and DL analyses
EE and DL are important factors of the MS. As shown in , the values of EE of MS A, MS B, MS C and MS I were 6.22, 5.77, 5.28, and 7.28%, respectively. The results of EE showed no significant differences among MS A, MS B, and MS C (p > .05), indicating that EE did not increase with an increase on the ratio of drug/polymer. However, the EE of porous MS I was improved significantly with ABC addition, which could be explained by the large surface area of interior duo to the porous support [Citation38]. In , the DL of MS A, MS B, MS C and MS I was 0.89, 1.15, 0.59, and 1.85%, respectively. Additionally, the DL of MS I was higher than any of the three nonporous MS (p < .05) duo to the large surface area of porous particles [Citation38]. The lower entrapment and drug-loading efficiencies of all MS could be explained by aspiration of the smallest and lightest particles formed by the non-encapsulated free drug and the corresponding excipients during the spray-drying process [Citation39]. Besides, the relatively low values of EE and DL could be because of low volumes of feed solution in each batch [Citation40]. Although the values of EE and DL were very low, the formulation endowed with porousness carried more drug than these nonporous MS because of its porosity and specific surface area [Citation41].
Aerodynamic properties
The DPIs aerosol deposition profiles for MS A, MS B, MS C, and MS I were investigated using NGI. As seen in , the differences in deposition on stages mimicked middle lung and deep lung delivery were remarkable among all MS. For MS A, MS B and MS C, these aerosol peak modes were on stage one with 41.28, 36.32 and 30.10%, respectively. For MS I, the aerosol peak mode was on stage three with 26.38%. Meanwhile, 23.46 and 23.85% of the aerosol powders were on stage two and four, respectively. illustrates that MS I with porousness increased aerosol deposition on lower NGI stages modelled for middle lung and deep lung delivery compared with the nonporous MS of MS A, MS B, and MS C. This result was consistent with previous researches that showed porous MS are more likely to target middle lung and deep lung delivery because of the smaller surface-to-volume ratio [Citation16,Citation17].
Figure 5. Aerosol dispersion performance properties containing deposition on stages (A), ED (B), FPF (C), RF (D), GSD (E) and MMAD (F). Bars represent standard errors of three replications. Different letters indicate significant differences (p < .05) according to Duncan’s multiple range tests.
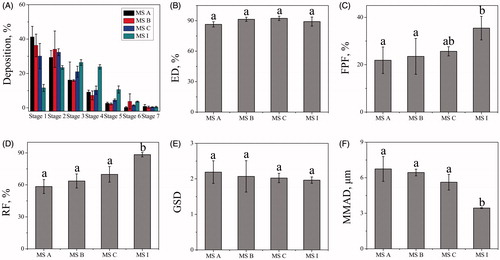
The aerosol performance parameters of the nonporous and porous MS were also shown in . For all MS, the ED values were in the range of ∼85–98% (), which represented considerably high efficiency in aerosolization. And, the ED values had no significant differences among these MS (p > .05). However, the FPF value for MS I was remarkably higher than that of MS A and MS B in (p < .05), which showing that the porous MS have a higher probability of penetrating into the deep lung than that of nonporous MS. The RF values were in the range of ∼55–88% for all MS in . For MS I, the value was higher than any of nonporous MS (p < .05), thereby suggesting that the presence of porousness favourably enhanced the aerosolization efficiency process. The GSD value is used to indicate the polydispersity of the particle size distribution. As shown in , this value showed no significant difference between the porous and nonporous MS (p > .05). MS I, with a GSD of 1.96 ± 0.09, was appropriate for pulmonary drug delivery according to previous researches [Citation25]. Representing the comprehensive characteristics of particle size, morphology and characteristics, the MMAD is a vital parameter for controlling the deposition of aerosol in the lungs since it can reflect the real and complex movement of particles in the respiratory system. As shown in , the MMAD value of MS I was 3.53 ± 0.20 µm which is in an inhalable range of 1–5 µm [Citation14]. On the contrary, other MS with a MMAD of more than 5 µm will more likely deposit in mouth or throat.
Biocompatibility
Assay for LDH and protein
LDH, an intracellular enzyme, is an indicator of membrane irritation and often regarded as a sensitive indicator of an inflammatory response when it is leaked into the BALF [Citation42]. To estimate the biocompatibility of the material, the total proteins and LDH activities were measured as shown in . The LDH activity in BALF administered with the dry powder was analysed by comparing with those examined after administration of PBS, Triton X-100 and the normal group, respectively. The LDH activities of HTCC and MS I administered after 24 h were increased but without statistical difference compared with the PBS and normal groups (p > .05). For the protein content, the result of Triton X-100 administered after 24 h was significantly increased compared with the MS I, HTCC, PBS and normal groups (p < .05). And there was no significant difference between the HTCC and normal groups, which was in agreement with previous study that HTCC is biocompatible [Citation6]. MS I also had no significant difference compared with the normal group, which was the same tendency compared with that observed after HTCC administration. Therefore, these results demonstrated that MS I could be applied as a potential safe sustained release pulmonary drug carrier.
Figure 6. In vivo biocompatibility including assay for protein (A) and LDH (B) in BALF on acute lung toxicity, and the microscopic structure of mucosa cells (C) and lasting time of ciliary movement (D) on ciliotoxicity study of in situ toad palate model. For acute lung toxicity, bars represent standard errors of four replications, and “*” compared with Triton X-100 group (p < .05) was observed according to a two-tailed paired t-test. For ciliotoxicity study, bars represent standard errors of six replications, and “Δ” compared with NS group (p < .05) was observed according to a two-tailed paired t-test.
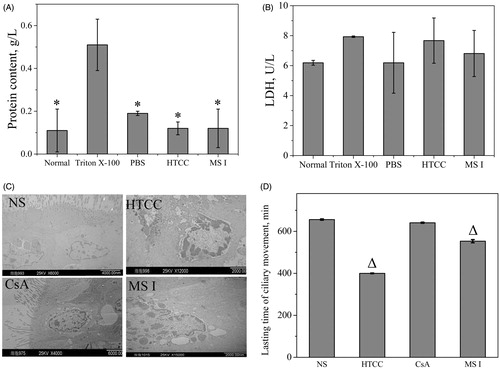
Ciliotoxicity study
The effects of the formulations on ciliary movement should be analysed, since the mucocilia movement as the body’s primary nonspecific defense mechanism is necessary to protect the respiratory system [Citation43]. The in situ toad palate model has been widely used in evaluating the ciliotoxicity of respiratory tract. The microscopic structure of mucosa cells and lasting time of the ciliary movement of each group were presented in . As shown in the , normal saline did not significantly affect the mucosal cell morphology. The mucosal cell morphology in HTCC group displayed significant typical apoptosis characteristics, including nuclear chromatin condensation and marginalization, thus suggesting that HTCC could suppress cell growth and promote apoptosis in mucosa cells, which demonstrated by the fact that the lasting time of HTCC samples after administration was more significantly decreased at a ratio of 60.98% than the normal saline in . This damage was maybe caused by the positive charge of HTCC which could have noxious stimuli on mucosa cells, and could be tailored by varying the DQ based on the previous research about the effect of quaternization degree on physiochemical and biological activities of CTS [Citation37]. However, the microscopic structure of mucosal cells in CsA group was normal with a prominent nucleolus and scattered chromatin granules, which was in consistent with the finding that no differences exist in the lasting time of ciliary movement with a ratio higher than 90%. MS I showed ciliotoxicity, which was obtained from the microscopic structure of mucosal cells with irregular nucleus and a significant decrease in the lasting time of ciliary movement compared with the normal saline group. But it was noticed that the ratio of 84% was higher than 80%, indicating that MS I had good safety profile and could be applied as pulmonary drug carrier [Citation43].
In vivo efficacy evaluation
Effects of MS I on OVA-induced total and differential leukocytes in BALF
OVA-induced asthma is a disease that results from chronic airway inflammation characteristically associated with the infiltration of total cells (TC), lymphocytes (LY), and eosinophils (EOS) into the bronchial lumen. The OVA-induced airway inflammation rat model of asthma was summarized, and the change of total and differential leukocytes in the BALF was examined. As shown in , in the BALF, the numbers of TC, EOS and LY were increased by 6.2-, 7.9- and 13.1-fold, respectively, compared with those in the control group. The numbers of OVA-induced TC, EOS, LY and monocytes were decreased by MS I and BUD administration. Compared with the MS I group, there was no significant difference in the BUD group. These results suggested that MS I inhibited the OVA-induced inflammatory response in a rat model of asthma.
Figure 7. In vivo efficacy in the asthmatic rat model including effects of MS I on OVA-induced total and differential leukocytes in BALF (A), effects of MS I on OVA-induced levels of IL-4 and TNF-α (B), effects of MS I on OVA-induced levels of total protein (C) and LDH (D) in BALF, and effects of lung histopathological changes. Bars represent standard errors of five replications. “a” compared with the control group (p < .05) was observed according to a two-tailed paired t-test. “b” compared with the OVA-treated group (p < .05) was observed according to a two-tailed paired t-test.
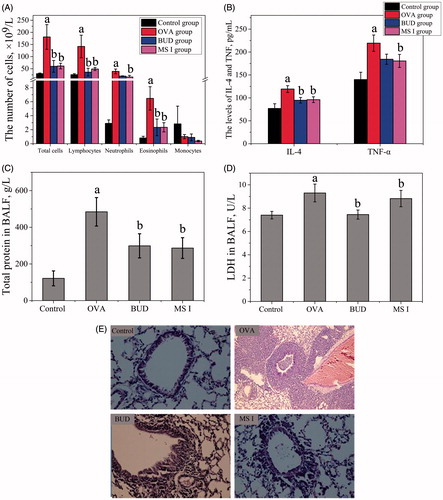
EOS are considered the key effector cells in the pathogenesis of asthma through destroying epithelial cells, participating in the release of some inflammatory cytokines (IL-4 and IL-5), and inducing bronchial hyperresponsiveness. The inflammatory cells and inflammatory factors have an interaction, and the inflammatory process is enlarged in each step after inflammatory cells have increased the expression of inflammatory cytokines and inflammatory cytokines, thereby increasing the survival of inflammatory cells [Citation44]. CsA aerosol inhalation can inhibit allergic airway inflammation in mice [Citation45]. In our study, the TC and MS I groups in the BALF of rats were significantly reduced compared with the model set, and the effect on EOS was not significantly different compared with the BUD inhalation group. This observation showed that the anti-inflammatory effect of MS I in our experiment not only resembles BUD, but also its required dose was less than that in previous experiments [Citation46].
Effects of MS I on OVA-induced levels of IL-4 and TNF-α inflammatory cytokines in BALF
In the present study, MS I could significantly reduce the characteristics of airway inflammation, and infiltration, as well as the production of inflammatory cytokines. At the inflammation sites, multiple inflammatory cells, including EOS and NE, can generate reactive oxygen species (ROS), which can participate in the development of allergic asthma. ROS promote the activities of pro-inflammatory redox-sensitive nuclear factors, including NF-kB, which can induce various inflammatory genes, including IL-4 and TNF-α, and increase the occurrence of allergic inflammation in asthmatic patients [Citation47]. The OVA-induced levels of inflammatory cytokines were increased compared with the control levels, according to the levels of IL-4 and TNF-α cytokines in BALF analysed using ELISA (). The levels of TNF-α and IL-4 were slightly increased by 1.59-, and 1.57-fold, respectively, compared with those in the control group. MS I and BUD considerably inhibited the level of TNF-α and IL-4 compared with that in the OVA-induced group. The level of TNF-α was more weakly inhibited by CK administration compared with that in the OVA-induced control group. The BUD group was slightly higher than that of MS I group, but the difference was not statistically significant. The result showed that MS I group significantly reduced the TNF-α content in BALF, suggesting that MS I inhibited airway inflammation and improved symptoms resembling BUD, which may be achieved through reduction cytokines release.
Effects of MS I on OVA-induced levels of total protein and LDH in BALF
LDH is a kind of glucolytic enzyme existing in all kinds of organizations and cells in human body. LDH level would increase when the human body tissue is injured [Citation48]. Therefore, LDH activities and total protein were also investigated to evaluate the membrane-irritating potential of the MS accurately. The levels of total protein and LDH in the BALF were measured through ELISA in , and the results revealed that their levels increased by 4.1- and 1.2-fold, respectively, compared with the control group. The total protein was significantly inhibited by MS I and BUD administration. The BUD group was slightly higher than that of MS I group, but the difference was not statistically significant. These results suggested that MS I inhibited the total protein and LDH level in the rat model of asthma.
Effects of lung histopathological changes
OVA induced the infiltration of inflammatory cells into the lung tissue compared with that in the control group in . MS I and BUD attenuated the OVA-induced infiltration of inflammatory cells into the lung tissue. MS I also inhibited the OVA-induced mucus hypersecretion compared with those in the OVA-inhaled group. The BUD weakened the increased inflammation and mucus score, which represented EOS-rich leukocyte infiltration and mucus secretion. These results suggested that MS I inhibited the OVA-induced inflammatory infiltration and mucus hypersecretion in an asthma rat. CsA, as an immune inhibitor and similar to glucocorticoid, can inhibit the invasion of inflammatory cells in asthma and reduce the leakage of micro blood vessels in the lung [Citation49]. In the present study, the white blood cell (WBC) and neutrophil (NE) contents in BALF at the asthma rats were reduced by MS I treatment, and the inflammatory reaction was reduced compared with the asthma model as showed by the pathological results. Therefore, the MS I had therapeutic effects on OVA-induced asthma rats.
Conclusion
In this study, porous MS containing CsA for pulmonary delivery were developed. MS I, which possessed a spherical morphology with smooth surface and pore structure, was the optimal formulation with regard to morphology. Characterized by the smaller surface-to-volume ratio, MS I exhibited larger MVD, but smaller MMAD compared with nonporous particles, which indicated that MS I has high lung deposition. This observation could be further confirmed by their excellent aerodynamic properties. The EE and DL of porous MS I were also higher compared with those of other nonporous MS. In addition, the biocompatibility of MS I, including the acute lung toxicity and ciliotoxicity estimated in vivo, demonstrated that MS I was safe as inhalation dry powders. We also demonstrated that MS I markedly decreased the number of the TP, LDH, inflammatory cells, IL-4 and TNF-α cytokines compared with those in the OVA-induced group, and reduced the leukocyte classification levels in BALF. Based on lung histopathological studies, inflammatory cell infiltration was inhibited by MS I administration compared with that in the OVA-induced group. Taken together, the porous HTCC MS are a promising class of drug carrier for local sustained inhalation therapy of pulmonary diseases.
Disclosure statement
No potential conflict of interest was reported by the authors.
Additional information
Funding
References
- Ahmadi Nasab N, Hassani Kumleh H, Beygzadeh M, et al. Delivery of curcumin by a pH-responsive chitosan mesoporous silica nanoparticles for cancer treatment. Artif Cells Nanomed Biotechnol. 2018;46:75–81.
- Wang F, Wang Y, Ma Q, et al. Development and characterization of folic acid-conjugated chitosan nanoparticles for targeted and controlled delivery of gemcitabinein lung cancer therapeutics. Artif Cells Nanomed Biotechnol. 2017;45:1530–1538.
- Yamamoto H, Kuno Y, Sugimoto S, et al. Surface-modified PLGA nanosphere with chitosan improved pulmonary delivery of calcitonin by mucoadhesion and opening of the intercellular tight junctions. J Control Release. 2005;102:373–381.
- Yang X, Zhang C, Qiao C, et al. A simple and convenient method to synthesize N-[(2-hydroxyl)-propyl-3-trimethylammonium chitosan chloride in an ionic liquid. Carbohydr Polym. 2015;130:325–332.
- Li SD, Li PW, Yang ZM, et al. Synthesis and characterization of chitosan quaternary ammonium salt and its application as drug carrier for ribavirin. Drug Deliv. 2014;21:548–552.
- Soares PI, Sousa AI, Ferreira IM, et al. Towards the development of multifunctional chitosan-based iron oxide nanoparticles: optimization and modelling of doxorubicin release. Carbohydr Polym. 2016;153:212–221.
- Kaur R, Garg T, Das Gupta U, et al. Preparation and characterization of spray-dried inhalable powders containing nanoaggregates for pulmonary delivery of anti-tubercular drugs. Artif Cells Nanomed Biotechnol. 2016;44:182–187.
- Zhang Y, Zhang J. Preparation of budesonide nanosuspensions for pulmonary delivery: characterization, in vitro release and in vivo lung distribution studies. Artif Cells Nanomed Biotechnol. 2016;44:285–289.
- Bhardwaj A, Mehta S, Yadav S, et al. Pulmonary delivery of antitubercular drugs using spray-dried lipid-polymer hybrid nanoparticles. Artif Cells Nanomed Biotechnol. 2016;44:1544–1555.
- Liang Z, Ni R, Zhou J, et al. Recent advances in controlled pulmonary drug delivery. Drug Discov Today. 2015;20:380–389.
- Feng R, Zhang Z, Li Z, et al. Preparation and in vitro evaluation of etoposide-loaded PLGA microspheres for pulmonary drug delivery. Drug Deliv. 2014;23:1962–1971.
- Wang Y, Zhu LH, Chen AZ, et al. One-step method to prepare PLLA porous microspheres in a high-voltage electrostatic anti-solvent process. Materials. 2016;9:368.
- Chen AZ, Zhao C, Wang SB, et al. Generation of porous poly-L-lactide microspheres by emulsion-combined precipitation with a compressed CO2 antisolvent process. J Mater Chem B. 2013;1:2967–2975.
- Patton JS, Byron PR. Inhaling medicines: delivering drugs to the body through the lungs. Nat Rev Drug Discov. 2007;6:67–74.
- Edwards DA, Hanes J, Caponetti G, et al. Large porous particles for pulmonary drug delivery. Science. 1997;276:1868–1871.
- Hirst PH, Pitcairn GR, Weers JG, et al. In vivo lung deposition of hollow porous particles from a pressurized metered dose inhaler. Pharm Res. 2002;19:258–264.
- Kim TK, Yoon JJ, Lee DS, et al. Gas foamed open porous biodegradable polymeric microspheres. Biomaterials. 2006;27:152–159.
- Vitali L, Della Betta F, Cost AC, et al. New multilayer coating using quaternary ammonium chitosan and κ-carrageenan in capillary electrophoresis: application in fast analysis. Talanta. 2014;123:45–53.
- Wu Y, Wu S, Hou L, et al. Novel thermal-sensitive hydrogel enhances both humoral and cell-mediated immune responses by intranasal vaccine delivery. Eur J Pharm Biopharm. 2012;81:486–497.
- Wei W, Lv PP, Chen XM, et al. Codelivery of mTERT siRNA and paclitaxel by chitosan-based nanoparticles promoted synergistic tumor suppression. Biomaterials. 2013;34:3912–3923.
- Brewster ME, Loftsson T. Cyclodextrins as pharmaceutical solubilizers. Adv Drug Deliv Rev. 2007;59:645–666.
- Kanokpanont S, Yamdech R, Aramwit P. Stability enhancement of mulberry-extracted anthocyanin using alginate/chitosan microencapsulation for food supplement application. Artif Cells Nanomed Biotechnol. 2018;46:773–782.
- Balmayor ER, Tuzlakoglu K, Azevedo HS, et al. Preparation and characterization of starch-poly-epsilon-caprolactone microparticles incorporating bioactive agents for drug delivery and tissue engineering applications. Acta Biomater. 2009;5:1035–1045.
- Kankala RK, Zhang YS, Wang SB, et al. Supercritical fluid technology: an emphasis on drug delivery and related biomedical applications. Adv Healthcare Mater. 2017;6:1–31.
- Wu X, Zhang WF, Hayes D Jr, et al. Physicochemical characterization and aerosol dispersion performance of organic solution advanced spray-dried cyclosporine A multifunctional particles for dry powder inhalation aerosol delivery. Int J Nanomedicine. 2013;8:1269–1283.
- Holt J. The MMAD Calculator. 2009. Available from http://www.mmadcalculator.com/
- Chamieh J, Zimmermann Y, Boos A, et al. Preparation and characterisation of silica monoliths using a triblock copolymer (F68) as porogen. J Colloid Interface Sci. 2009;340:225–229.
- Ma B, Li X, Qin A, et al. A comparative study on the chitosan membranes prepared from glycine hydrochloride and acetic acid. Carbohydr Polym. 2013;91:477–482.
- Zuo HG, Zhu JX, Zhan CR, et al. Preparation of malathion MIP-SPE and its application in environmental analysis. Environ Monit Assess. 2015;187:1144–1153.
- Li J, Zhang Y. Porous polymer films with size-tunable surface pores. Chem Mater. 2007;19:2581–2584.
- Meenach SA, Kim YJ, Kauffman KJ, et al. Synthesis, optimization, and characterization of camptothecin-loaded acetalated dextran porous microparticles for pulmonary delivery. Mol Pharmaceutics. 2012;9:290–298.
- Jóhannsdóttir S, Jansook P, Stefánsson E, et al. Development of a cyclodextrin-based aqueous cyclosporin A eye drop Formulations. Int J Pharm. 2015;493:86–95.
- Moffat JG, Qi S, Craig DQ. Spatial characterization of hot melt extruded dispersion systems using thermal atomic force microscopy methods: the effects of processing parameters on phase separation. Pharm Res. 2014;31:1744–1752.
- Yamasaki K, Kwok PC, Fukushige K, et al. Enhanced dissolution of inhalable cyclosporine nano-matrix particles with mannitol as matrix former. Int J Pharm. 2011;420:34–42.
- Tam JM, McConville JT, Williams RO, 3rd, et al. Amorphous cyclosporin nanodispersions for enhanced pulmonary deposition and dissolution. J Pharm Sci. 2008;97:4915–4933.
- Chen X, Young TJ, Sarkari M, et al. Preparation of cyclosporine A nanoparticles by evaporative precipitation into aqueous solution. Int J Pharm. 2002;242:3–14.
- Huang J, Cheng ZH, Xie HH, et al. Effect of quaternization degree on physiochemical and biological activities of chitosan from squid pens. Int J Biol Macromol. 2014;70:545–550.
- Volodkin DV, Larionova NI, Sukhorukov GB. Protein encapsulation via porous CaCO3 microparticles templating. Biomacromolecules. 2004;5:1962–1972.
- Sarisözen C, Arica B, Orman MN, et al. Optimization of prednisolone acetate-loaded chitosan microspheres using a 2(3) factorial design for preventing restenosis. Drug Deliv. 2010;17:178–186.
- Gungor S, Okyar A, Erturk-Toker S, et al. Ondansetron-loaded chitosan microspheres for nasal antiemetic drug delivery: an alternative approach to oral and parenteral routes. Drug Dev Ind Pharm. 2010;36:806–813.
- Cai Y, Chen Y, Hong X, et al. Porous microsphere and its applications. Int J Nanomedicine. 2013;8:1111–1120.
- Henderson RF. Use of bronchoalveolar lavage to detect lung damage. Environ Health Perspect. 1984;56:115–129.
- Na L, Wang J, Wang L, et al. A novel permeation enhancer: N-succinyl chitosan on the intranasal absorption of isosorbide dinitrate in rats. Eur J Pharm Sci. 2013;48:301–306.
- Wong WS, Koh DS. Advances in immunopharmacology of asthma. Biochem Pharmacol. 2000;59:1323–1335.
- Balsley MA, Malesevic M, Stemmy EJ, et al. A cell-impermeable cyclosporine A derivative reduces pathology in a mouse model of allergic lung inflammation. J Immunol. 2010;185:7663–7670.
- Aalbers R, Vogelmeier C, Kuna P. Achieving asthma control with ICS/LABA: a review of strategies for asthma management and prevention. Respir Med. 2015;111:1–7.
- Ricciardolo FL, Petecchia L, Sorbello V, et al. Bradykinin B2 receptor expression in the bronchial mucosa of allergic asthmatics: the role of NF-kB. Clin Exp Allergy. 2016;46:428–438.
- Pritchard KA Jr, Feroah TR, Nandedkar SD, et al. Effects of experimental asthma on inflammation and lung mechanics in sickle cell mice. Am J Respir Cell Mol Biol. 2012;46:389–396.
- Xie QM, Chen JQ, Shen WH, et al. Effects of cyclosporin A by aerosol on airway hyperresponsiveness and inflammation in guinea pigs. Acta Pharmacol Sin. 2002;23:243–247.