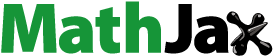
Abstract
Magnetic ferrite nanoparticles (MNPs) coated with biocompatible polymers capable of drug loading and release are fascinating nanostructures for delivering anti-cancer drugs. Herein, we report the synthesis and characterization of a novel β-cyclodextrin-folate-tethered dextran polymer. Nickel–zinc ferrite nanoparticles are prepared and coated with the polymer to form a biocompatible hybrid magnetic nanocarrier. To establish the significance of folate unit of the polymer in anticancer activity, a similar derivatized polymer, i.e. β-cyclodextrin-dextran conjugate without folate tether is used for comparison. The size of the hybrid MNPs is ∼20 nm, which is a size suitable for cancer drug targeting. The polymer-coated magnetic nanocarriers are soft ferromagnets as suggested by their narrow magnetic hysteresis loops. The anticancer drug camptothecin (CPT) is loaded on the magnetic nanocarriers. The drug loading efficiency is observed to be above 92%. The nanocarriers show sustained in vitro drug release for above 45 h. The in vitro cytotoxicity studies reveal that the loaded CPT retains its potency in the nanocarrier and the folate-tethered nanocarrier shows better anticancer activity than the one which does not carry a folate unit. The magnetic nanocarrier is suitable for magnetic field-guided drug transport, enhanced drug loading and release and folate receptor-mediated endocytotic uptake of drugs by cancer cells.
Introduction
Magnetic drug targeting has acquired attention as an innovative approach by which magnetic nanoparticles (MNPs) loaded with drug are guided to specific tissues of the body by the application of an external magnetic field [Citation1,Citation2]. Ferrite nanomaterials of various chemical compositions have been used in magnetic drug targeting [Citation3–6]. Improvement of magnetic properties and toxicity are some of the parameters sought through the endeavour of testing new MNPs [Citation7]. Another challenge is the development of coating polymers, which combine biocompatibility and ability to escape macrophage uptake [Citation8–10]. In addition, the overall size of the polymer-coated MNPs should be of a desirable range (>20 nm and <100 nm) as MNPs larger than 100 nm are rapidly cleared by the liver and spleen [Citation11] and MNPs of size below 10 nm can be cleared by renal filtration [Citation12]. Particles in the desirable range of size have the longest blood circulation time [Citation7].
Spinel ferrites substituted with elements like Mn2+, Co2+, Ni2+ and Zn2+ provide opportunity to fine-tune the magnetic characteristics of the MNP core depending on the kind of divalent ion [Citation7]. Coating of MNPs with biocompatible carbohydrate polymers like dextran mitigates the toxicity of the MNPs. The induction of reactive oxygen species (ROS) depends on the firmness of the coating agent, its inclination to produce ROS and concentration inside the cells [Citation13–16]. A few dextran-coated MNP formulations have been approved as MRI contrast agents by the FDA and EMA [Citation7]. A raise to a more desirable or better quality of recourse of tumour specific hitting, water-soluble folate conjugates are designed and synthesized as the folate conjugation facilitates the uptake by folate-receptor overexpressed cells [Citation17].
Covalent linking of anticancer drugs has been a strategy to aim at cancer cells [Citation17–19]. However, such strong bonds between the polymer and the drug need to be cleaved so that the prodrug action becomes tangible. Drug loading on the polymer shell by physical loading, on the other hand, relies on hydrophobic/hydrophilic interactions as a method simple to realize [Citation20,Citation21]. Drug leakage through the polymer layer is undesirable but possible in such a kind of drug loading. A novel method which overcomes the above-mentioned shortcomings is the functionalization of polymers with a cavity-containing molecule such as β-cyclodextrin (β-CD) [Citation22,Citation23]. β-CD is a non-toxic cyclic oligosaccharide consisting of seven α-d-glucopyranose units in the form of a ring [Citation24]. The structure of β-CD is intriguing due to its hydrophilic exterior and the hydrophobic inner cavity capable of forming host–guest association with drug molecules of appropriate size and shape [Citation25]. Hence, tethering β-CD with dextran offers a possible unique mode of encapsulation of drugs which can effectuate enhanced drug loading and release. Moreover, folate tagging of the β-CD-dextran conjugate could assist or expedite precise targeting.
Nickel–zinc ferrite nanoparticles have been reported active against breast cancer MCF7, colon cancer HT29 and liver cancer HepG2 cells as cytotoxic agents [Citation26]. Apoptosis was induced in liver cancer than in breast and colon cancer cells through a capase-9-dependent pathway. Because of high-magnetic dipole interactions between particles agglomeration was observed and the diameter of the NPs was in the range of 10–30 nm [Citation26]. On the basis of the above line of rumination, we have carried out the present work with a design of a novel carrier system through the synthesis of nickel–zinc ferrite magnetic nanoparticles in a preferred size range and a folate- and β-CD-tagged dextran as the tumour-specific biocompatible polymer and combining those for sharp-shooting cancer cells.
Materials and methods
Materials
The AnalaR reagents (p-toluenesulfonic anhydride, ethylene diamine and epichlorohydrin) used for the synthesis of the β-CD-derivative of dextran were products of Aldrich (Bangalore, India). β-CD was supplied by Hi Media (Bangalore, India), and dextran and folic acid by Aldrich. All the solvents used for the synthesis (in AR grade) and for UV-visible spectral measurements (in spectral grade) were purchased from Aldrich. The reagents used for the preparation of ferrite NPs, namely zinc nitrate, ferric nitrate, ammonium hydroxide and tetraethyl orthosilicate were received from Merck (Bangalore, India). The purity of the chemicals was above 98%. The dialysis membranes used for the drug release study were the products of Hi Media (diameter 14.33 mm, LA387–1MT). 3–(4,5-Dimethylthiazol-2-yl)-2,5-diphenyltetrazolium bromide (MTT) was procured from Hi Media and the human cervical cancer cells (HeLa) from National Centre for Cell Science (Pune, India).
In vitro cytotoxicity
MTT assay was performed to evaluate the cytotoxic effects of various nano-formulations (CPT, CD-Dx, Dx-CD-FA, DNPs, FDNPs, CPT@DNPs, CPT@FDNPs) on HeLa (cervical cancer) cells. In brief, the HeLa cells were sub-cultured by using Dulbecco’s modified Eagles media (DMEM) supplemented with 5% foetal calf serum (FCS). The cultured HeLa cells were seeded in 96-well cell culture plates at a density of 5 × 104 cells/well and incubated for 24 h at 37 °C, 5% CO2. After 24 h, fresh DMEM medium with nano-formulations of different concentration ranging 0.5, 1.0, 5.0, 10 and 20 µg/ml was added and then incubated for 24 h. Untreated cells were used as a control. After incubation, the medium was removed and washed with PBS. 100 µl of fresh DMEM medium containing 10 µl of MTT reagent (5 mg/ml in PBS) was added to each well and incubated for 5 h for the formation of purple formazan crystals and the absorbance was determined by using an ELISA plate reader at 540 nm.
Preparation of nickel–zinc ferrite MNPs
A certain amount of Ni (NO3)2⋅6H2O (0.5 mole), Zn(NO3)2⋅6H2O (0.5 mole) and Fe (NO3)3⋅9H2O (1 mole) were dissolved in distilled water and stirred till transparent solution was obtained. To the latter was added Si (OC2H5)4 (2 moles) and a few drops of concentrated nitric acid and the mixture was stirred in a magnetic stirrer for a half hour. The solution so obtained was cast in a Petri dish. The gelation of the solution took place in 24 h. The acquired substance was then grinded into a fine powder and then annealed for 3 h at 600 °C [Citation27].
Synthesis of 6-O-tosyl-β-cyclodextrin (Ts-CD)
6-O-Tosyl-β-cyclodextrin was prepared using the procedure reported by Raoov et al. [Citation28]. In a typical procedure, β-CD (11.5 g, 10 mmol) and p-toluenesulfonic anhydride (4.9 g, 15 mmol) were mixed in 250 ml of water. The suspension was stirred at room temperature for 2 h. 50 ml of 10% NaOH solution was added to the above solution, with stirring. The mixture was filtered, brought to pH 8.0 by adding ammonium chloride (13.4 g) and then the precipitate product of Ts-CD was collected by cooling at 4 °C overnight.
Synthesis of 6-deoxy-6-aminoethylamino-β-cyclodextrin (CD-en)
6-Deoxy-6-aminoethylamino-β-cyclodextrin was prepared using the procedure reported by Izawa et al. [Citation29]. Under nitrogen atmosphere, Ts-CD (1.50 g, 1.17 mmol) and ethylene diamine (5 ml) were mixed and stirred at 60 °C for 12 h. After cooling it to room temperature, the mixture was poured into ethanol. The precipitated product was filtered, washed with ethanol and dried.
Synthesis of β-CD-dextran conjugate (CD-Dx)
Epichlorohydrin (80% by weight) was added to an aqueous solution of dextran containing 2.8 M NaOH (20% w/v) and stirred at 40 °C using a magnetic stirrer bar for 1 h (procedure reported by Meybodi et al.) [Citation30]. To the cross-linked dextran containing epoxide groups (1.0 g, 1 eq.), CD-en (0.05 g, 1 eq.) was added at an alkaline condition (mentioned in the previous step) at 65 °C, and stirred magnetically for a period of 2 h. The product obtained was washed with alcohol and dried. The synthesized compound was characterized by spectroscopic measurements.
Synthesis of β-CD-folate conjugate (CD-FA)
Folic acid (1 mmol), dicyclohexylcarbodiimide, (1.1 mmol) and N-hydroxysuccinimide (1.1 mmol) were dissolved in 40 ml of DMSO and stirred overnight in a dark room. The white precipitate (reaction by-product of dicyclohexyl urea) formed was removed by vacuum nitration. 6-Deoxy-6-aminoethylamino-β-CD (1 mmol) was dissolved in 2 ml of DMSO and added to the now activated folic acid (1 mmol) and stirred for 6 h, yelding a yellow solid when dried in vacuum.
Synthesis of dextran-β-CD-folate conjugate (Dx-CD-FA)
Epichlorohydrin (80% by weight) was added to an aqueous solution of dextran containing 2.8 M NaOH (20% w/v) and stirred at 40 °C using a magnetic stirrer bar for 1 h (procedure reported by Meybodi et al.) [Citation30]. To the cross-linked dextran-containing epoxide groups (1 eq.), CD-FA (1 eq.) was added at an alkaline condition (mentioned in the previous step) at 65 °C, and stirred magnetically for a period of 2 h. The product obtained was washed with alcohol and dried. The synthesis of the β-CD-folate conjugate is illustrated in Scheme 1.
Polymer coating of MNPs
The MNPs were coated with either the CD-Dx/Dx-CD-FA conjugate by adding 10 mg of each of the polymer with 10 mg of MNPs in 0.5 M NaOH solution and then sonicating (from now on, the CD-Dx and the Dx-CD-FA coated MNPs will be referred to as DNPs and FDNPs, respectively).
Drug-encapsulation efficiency
Pre-calculated quantities of the freeze-dried CPT@DNPs and CPT@FDNPs were weighed and dispersed in PBS (pH 7.4). The concentration of CPT was measured by recording UV–visible spectra at 368 nm. The encapsulation efficiency (EE) was calculated using the following relation [Citation31]:
(1)
(1)
In vitro drug release
By suspending the CPT@DNPs and CPT@FDNPs in dialysis bags, the in vitro drug release was studied in dialysis bags. The dialysis bags used for each sample were immersed in PBS solution of two different pH values (7.4 and 6.0) and kept on a shaking bath. The solutions outside the bags were withdrawn at pre-planned time intervals to determine the concentration of the released drug [Citation32]. The volume in the dialysis system was kept constant by adding fresh buffer solutions. The concentration of CPT was determined using UV–visible spectral measurement. Three independent experiments were done and the average values were calculated.
Results and discussion
Preparation and characterization of the polymer-coated MNPs
DNPs and FDNPs were fabricated by incorporating the MNPs into the corresponding polymer, namely CD-Dx and Dx-CD-FA, respectively. The X-ray diffraction patterns shown in indicate that the sol–gel method used in the synthesis of MNPs has resulted in the formation of nickel–zinc ferrite in inverse spinel form as suggested by the reflections corresponding to the indices (2 2 0), (3 1 1), (4 4 0) and (5 1 1). The prominent peaks are visible in the CD-Dx and Dx-CD-FA coated forms also. An average grain size of ∼10 nm is determined using the Scherrer equation [Citation33], which employs the peak broadening data. In addition, it is observed that the MNPs belong to the cubic crystal system. The EDX spectrum () enables a qualitative analysis of the as-synthesized magnetic nanoparticles, which clearly reveals the presence of the elements Fe, Ni, Zn and O. Furthermore, the exact elemental composition of the NPs and their stoichiometry was quantitatively determined by ICP-AES and the composition is Ni0.45Zn0.47Fe2.08O4. The size distribution of the CD-Dx- and Dx-CD-FA-coated MNPs are shown in , respectively. The dynamic light scattering measurements result in the size distribution profiles with the average diameter at 20 and 20.6 nm, respectively, of the CD-Dx and Dx-CD-FA shrouded MNPs (). This size range of the NPs is visibly recognized from the TEM images of MNPs shown in .
Figure 1. (A) X-ray diffraction patterns of as-prepared MNPs, FDNPs and DNPs. (B) Energy-dispersive X-ray spectrum of as-prepared MNPs. (C) Particle size distribution of DNPs observed from dynamic light scattering. (D) Particle size distribution of FDNPs observed from dynamic light scattering. (E) Transmission electron microscopic image of DNPs. (F) Transmission electron microscopic image of FDNPs.
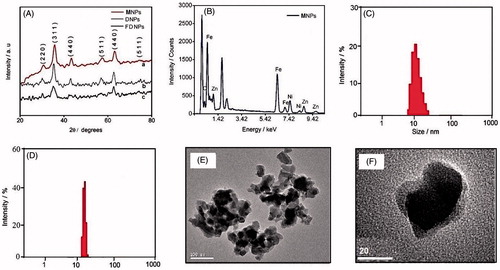
To further confirm the presence of various elements and to determine their electronic states, X-ray photoelectron spectrum of the FDNPs was recorded (). XPS remains a better reliable technique than EDX and it is noteworthy that the 2p and 3p electron peaks are seen separately for Fe, Ni and Zn.
Figure 2. (A) X-ray photoelectron spectra of MNPs. (B) Thermogravimetric curves of DNPs and FDNPs. (C) Magnetization curves of DNPs, MNPs and FDNPs.
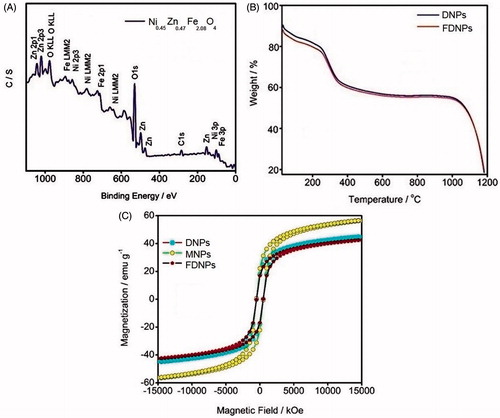
shows the thermogravimetric profile of the DNPs and FDNPs. Both the samples of MNPs coated with different polymers show similar effects on the incremental changes of temperature. Water present in the form of moisture and hydration of the polymer matrix leaves off up to 170 °C and the polymers start melting and decomposing up to 260 °C. Phase transition of the oxide material is observed above 1000 °C. The decreased weight percentage (∼40%) corresponds to the polymer coverage of the MNPs. It is hence inferred that the polymers cover-up the MNPs and remain intact [Citation34].
Magnetic property of the MNPs
Samples of MNPs were prepared each in a few milligrams in order that all the samples are aggregated equally before studying their magnetic properties. VSM of the MNPs in free and polymer-coated forms were recorded to observe the hysteresis loops. Narrow hysteresis loops are observed in all the three cases, namely as-synthesized MNPs, DNPs and FDNPs, showing that the particles are of soft ferromagnetic behaviour. The saturation magnetization (MS) value of the as-prepared MNPs is 64 emu g−1, which is larger than that of the polymer-shrouded ones, namely DNPs (44 emu g−1) and FDNPs (42 emu g−1). The remanence and coercivity of the different samples of MNPs are similar. The response of the MNPs to the applied field is strong and removal of the field does not lead to a complete magnetic relaxation. However, the coercivity and remanence are not high, which means that the MNPs are suitable for drug transport [Citation35].
Synthesis and characterization of the FA-Dx polymer
We have already reported the synthesis and characterization of the CD-functionalized dextran (CD-Dx) [Citation16]. The synthesis of Dx-CD-FA is discussed in the experimental section. The IR and 1H NMR spectra of the Dx-CD-FA polymer are shown in . The IR spectrum shows prominent bands at 3320.23, 2924.75, 1698.07, 1645.44, 1512.98 and 1023.36 cm−1. The first three bands concord with the O–H, aliphatic C–H and C=O stretching vibrations, respectively. The 1645.44, 1572.98 and 1028.36 cm−1 bands correspond to the C–N stretching, N–H bending and C–O stretching, respectively. The N–H stretching has merged with the broad OH stretching band.
The 1H NMR spectrum of Dx-CD-FA is shown in . The H-5 and H-6 protons of the glucopyranose rings of dextran are observed at 3.71 ppm. The H-3 proton and the H-2, H-4 protons resonate at 3.45 and 3.13 ppm, respectively. The peak at 4.80 ppm is due to the H-1 of dextran. The H-5 proton signal of β-CD is observed at 3.62 ppm. The H-3 proton signals are seen merged with those of the H-2, H-4 protons of dextran. The peak at 4.53 ppm corresponds to the primary hydroxyls of β-CD. The aromatic proton of the phenyl ring of the folate unit is seen resonating between 7.0 and 7.6 ppm. The pteridine group protons resonate between 8.3 and 8.7 ppm. The methyl proton signals of the folate unit are observed at 2.33 ppm. Protons of the dextran and the β-CD moieties are indicated with numbers in (β-CD is shown as a bucket). The IR and the NMR spectra unambiguously confirm the formation of the Dx-CD-FA derivative.
Drug loading and drug release
The encapsulation efficiency of CPT in DNPs and FDNPs was calculated using Equation (1). The calculated encapsulation efficiencies are 93.2 ± 3.4 and 92.1 ± 3.8, respectively, for DNPs and FDNPs. This means that an excellent loading of drugs on the polymer-coated MNPs is achieved. This is enabled by the tethering of β-CD to the polymers, which can form host–guest complexes with CPT [Citation36]. The in vitro release profiles of CPT from the carriers were measured using dialysis membranes as discussed in the experimental section. The loaded drug is released from the carriers well over a period of 45 h, both under the pH conditions of 7.4 and 6.0 at 35 °C. The two pH values are chosen as the physiological pH is 7.4 and in the tumour microenvironment the pH is decreased due to acidic metabolites caused by anaerobic glycolysis in hypoxia [Citation37]. The cumulative release of CPT is 65% at pH 7.4 and 38% at pH 6.0 in the case of CPT@DNPs, whereas 73% and 47% at pH 7.4 and 6.0, respectively, in the case of CPT@FDNPs. The sustained release means that the therapeutic efficiency will be maintained and will aid in the prevention of the development of drug resistance by the cancer cells. Hence, the polymer-coated MNPs are promising candidates for in vivo cancer therapy by magnetic field guiding.
The MTT assay was done to assess the cytotoxic effects of the formulated nanoconjugated drugs against cervical cancer cells. HeLa cells were treated with different concentrations of CPT, CD-Dx, Dx-CD-FA, DNPs, FDNPs, CPT@DNPs and CPT@FDNPs for 24 h and cell survival was determined by MTT assay (). It is observed that the cytotoxic effects occur in a dose-dependent manner. In HeLa cancer cells, the cytotoxic activity of the nanoconjugates follows the order (CPT@FDNPs) > (CPT) > (CPT@DNPs) > (FDNPs) > (DNPs) > (Dx-CD-FA) > (Dx-CD). The toxicity is reduced when FDNPs are used as a carrier. Among the complexes, FDNPs–CPT is found effective on the HeLa cells with an LD50 value of 1.5 µg/ml at a dose of 20 µg/ml, whereas the LD50 values of other complexes are 3.9 µg/ml for DNPs–CPT, 4.1 µg/ml for FDNPs, 9.19 µg/ml for DNPs and 17.13 µg/ml for Dx-CD-FA, respectively. The MNPs were loaded with Dxt, β-CD and CPT and evaluated for their toxicity against HeLa cells. To improve the effectiveness, folate unit is coupled with Dx-CD. The folate receptors are over-expressed on the surface of numerous cancer cells, whereas it expresses little on the surface of normal cells [Citation38,Citation39]. While comparing with CPT@DNPs and CPT@FDNPs on HeLa cells, the latter is more effective than the former. The CPT@FDNPs is less toxic (almost 10 times by magnitude) suggesting that the drug is not freely available in the cell culture media but is gradually released and targets in a manner that can help in sustained release of the drug and reducing toxicity but maintaining potency. The CPT@FDNPs exhibit significant cytotoxic effect against HeLa (cervical cancer) cells with low LD50 values. It indicates their efficiency in killing cancer cells even at low concentrations. Furthermore, from the results obtained, it is apparent that the folate-tethered nanoformulation has a significant cytotoxic activity against cervical cancer compared with other nanocarrier tested above and it aids in the sustained release of the drug for the treatment of cervical cancer. A summary of the entire work done is shown in Scheme 2.
Conclusions
We demonstrate a novel, simple and efficient method for tethering of β-CD and folate with dextran and coating it on magnetic Ni0.45Zn0.47Fe2.08O4 nanoparticles. The colloidal magnetic nanocarriers show augmented drug loading and sustained release of the popular cancer drug, CPT. The drug loading is above 92% and the release of drugs occurs over a period of 45 h. The MNPs behave as soft ferrites showing ferromagnetic behaviour as studied by vibrating sample magnetometry. Being in the size of ∼20 nm that falls in the range of size suitable for drug delivery, the magnetic nanocarrier is a promising structure for carrying drugs, i.e. it will not enter either the fenestrations of the glomerular endothelium or red pulp cords of the spleen. The systemic toxicity will be reduced on using the ferrite nanocarrier as the percentage of each of the cations (Ni2+, Zn2+ and Fe3+) in the crystal of the nickel–zinc ferrite are lesser than the larger Fe3+ percentage in the popular magnetic nanomaterial, Fe3O4. The folate unit is observed necessary for the uptake by the folate receptor over-expressed cancer cells. Unlike, the most common mode of release of drugs from the polymer-coated nanomaterials that require surface erosion of the polymer, the presently reported nanocarrier can withhold the drug through molecular host–guest association. The conjugation of folate, cyclodextrin and dextran to form the biocompatible polymeric carrier opens up varied types of drugs of appropriate size and shape (which get encapsulated through non-covalent interaction) as hybrid nanocarriers.
Disclosure statement
No potential conflict of interest was reported by the authors.
Additional information
Funding
References
- Peng N, Wu B, Wang L, et al. High drug loading and pH-responsive targeted nanocarriers from alginate-modified SPIONs for anti-tumor chemotherapy. Biomater Sci. 2016;4:1802–1813.
- Sehrig FZ, Majidi S, Nikzamir N, et al. Magnetic nanoparticles as potential candidates for biomedical and biological applications. Artif Cells Nanomed Biotechnol. 2016;44:918–927.
- Colombo M, Carregal-Romero S, Casula MF, et al. Biological applications of magnetic nanoparticles. Chem Soc Rev. 2012;41:4306–4334.
- Tziveleka L-A, Bilalis P, Chatzipavlidis A, et al. Development of multiple stimuli responsive magnetic polymer nanocontainers as efficient drug delivery systems. Macromol Biosci. 2014;14:131–141.
- Papaphilippou P, Loizou L, Popa NC, et al. Superparamagnetic hybrid micelles, based on iron oxide nanoparticles and well-defined diblock copolymers possessing β-ketoester functionalities. Biomacromolecules. 2009;10:2662–2671.
- Choi HS, Liu W, Liu F, et al. Design considerations for tumour-targeted nanoparticles. Nature Nanotech. 2010;5:42–47.
- Arbab AS, Bashaw LA, Miller BR, et al. Characterization of biophysical and metabolic properties of cells labeled with superparamagnetic iron oxide nanoparticles and transfection agent for cellular MR imaging. Radiology. 2003;229:838–846.
- Luong D, Sau S, Kesharwani P, et al. Polyvalent folate-dendrimer-coated iron oxide theranostic nanoparticles for simultaneous magnetic resonance imaging and precise cancer cell targeting. Biomacromolecules. 2017;18:1197–1209.
- Nagvi S, Samim M, Abdin MZ, et al. Concentration-dependent toxicity of iron oxide nanoparticles mediated by increased oxidative stress. Int. J. Nanomed. 2010;5:983–989.
- Liu Q, Zhu H, Qin J, et al. Theranostic vesicles based on bovine serum albumin and poly(ethylene glycol)-block-poly(l-lactic-co-glycolic acid) for magnetic resonance imaging and anticancer drug delivery. Biomacromolecules. 2014;15:1586–1592.
- Sakhteman A, Parhizkar G, Omidi M, et al. Synthesis and in vitro assessment of novel water‐soluble dextran‐docetaxel conjugates as potential pH sensitive system for tumor‐targeted delivery. J Appl Polym Sci. 2017;134:45457.
- Zu Y, Wang D, Zhao X, et al. A novel preparation method for camptothecin (CPT) loaded folic acid conjugated dextran tumor-targeted nanoparticles. Int J Mol Sci. 2011;12:4237–4249.
- Zhu Q, Bao B, Zhang Q, et al. Maleimidation of dextran and the application in designing a dextran–camptothecin conjugate. RSC Adv. 2018;8:2818–2823.
- Hervault A, Thanh NTK. Magnetic nanoparticle-based therapeutic agents for thermo-chemotherapy treatment of cancer. Nanoscale. 2014;6:11553–11573.
- Sudha N, Sameena Y, Enoch IVMV, et al. On the accessibility of surface-bound drugs on magnetic nanoparticles. Encapsulation of drugs loaded on modified dextran-coated superparamagnetic iron oxide by β-cyclodextrin. Colloids Surf B Biointerfaces. 2016;141:423–428.
- Sivaraj R, Beniya S, Reuther H, et al. Molecular encapsulator on the surface of magnetic nanoparticles. Controlled drug release from calcium Ferrite/Cyclodextrin–tethered polymer hybrid. Colloids Surf B Biointerfaces. 2018;161:347–355.
- Ak G, Yilmaz H, Güneş A, et al. In vitro and in vivo evaluation of folate receptor-targeted a novel magnetic drug delivery system for ovarian cancer therapy. Artif Cells Nanomed Biotechnol. 2018; 19. doi:10.1080/21691401.2018.1439838
- Sonvico F, Mornet S, Vasseur S, et al. Folate-conjugated iron oxide nanoparticles for solid tumor targeting as potential specific magnetic hyperthermia mediators: synthesis, physicochemical characterization, and in vitro experiments. Bioconjugate Chem. 2005;16:1181–1188.
- Barz M, Canal F, Koynov K, et al. Synthesis and in vitro evaluation of defined HPMA folate conjugates: influence of aggregation on folate receptor (FR) mediated cellular uptake. Biomacromolecules. 2010;11:2274–2282.
- Majd MH, Akbarzadeh A, Sargazi A. Evaluation of host–guest system to enhance the tamoxifen efficiency. Artif Cells Nanomed Biotechnol. 2017;45:441–447.
- Chandrasekaran S, Sameena Y, Enoch IV. Tuning the binding of coumarin 6 with DNA by molecular encapsulators: effect of β‐cyclodextrin and C‐hexylpyrogallol[4]arene. J Mol Recognit. 2014;27:640–652.
- Chandrasekaran S, Sudha N, Premnath D, et al. Binding of a chromen-4-one Schiff’s base with bovine serum albumin: capping with β-cyclodextrin influences the binding. J Biomol Struct Dyn. 2015;33:1945–1956.
- Morrison PWJ, Connon CJ, Khutoryanskiy VV. Cyclodextrin-mediated enhancement of riboflavin solubility and corneal permeability. Mol Pharmaceutics. 2013;10:756–762.
- Gorjikhah F, Jalalian FA, Salehi R, et al. Preparation and characterization of PLGA-β-CD polymeric nanoparticles containing methotrexate and evaluation of their effects on T47D cell line. Artif Cells Nanomed Biotechnol. 2016;45:432–440.
- Boztas AO, Karakuzu O, Galante G, et al. Synergistic interaction of paclitaxel and curcumin with cyclodextrin polymer complexation in human cancer cells. Mol Pharmaceutics. 2013;10:2676–2683.
- Al Qubaisi MS, Rasedee A, Flaifel MH, et al. Cytotoxicity of nickel zinc ferrite nanoparticles on cancer cells of epithelial origin. Int J Nanomedicine. 2013;8:2497–2508.
- Chatterjee A, Das D, Pradhan SK, et al. Synthesis of nanocrystalline nickel-zinc ferrite by the sol-gel method. J Magn Magn Mater. 1993;127:214–218.
- Raoov M, Mohamad S, Abas MR. Synthesis and characterization of β-cyclodextrin functionalized ionic liquid polymer as a macroporous material for the removal of phenols and As(V). Int J Mol Sci. 2014;15:100–119.
- Izawa H, Kawakami K, Sumita M, et al. β-Cyclodextrin-crosslinked alginate gel for patient-controlled drug delivery systems: regulation of host–guest interactions with mechanical stimuli. J Mater Chem B. 2013;1:2155–2116.
- Meybodi ZE, Imania M, Atai M. Kinetics of dextran crosslinking by epichlorohydrin: a rheometry and equilibrium swelling study. Carbohyd Polym. 2013;292:1792–1798.
- Zhu A, Yuan L, Jin W, et al. Polysaccharide surface modified Fe3O4 nanoparticles for camptothecin loading and release. Acta Biomater. 2009;5:1489–1498.
- Cao Z, Yue X, Li X, et al. Stabilized magnetic cerasomes for drug delivery. Langmuir. 2013;29:14976–14983.
- Jenkins R, Snyder RL. Introduction to X-ray powder diffractometry. New York (NY): John Wiley & Sons Inc.; 1996.
- Mansfield E, Tyner KM, Poling CM, et al. Determination of nanoparticle surface coatings and nanoparticle purity using microscale thermogravimetric analysis. Anal Chem. 2014;86:1478–1484.
- Hassan D, Khalil AT, Saleem J, et al. Biosynthesis of pure hematite phase magnetic iron oxide nanoparticles using floral extracts of Callistemon viminalis (bottlebrush): their physical properties and novel biological applications. Artif Cells Nanomed Biotechnol. 2018. doi:10.1080/21691401.2018.1434534
- Gigliotti CL, Ferrara B, Occhipinti S, et al. Enhanced cytotoxic effect of camptothecin nanosponges in anaplastic thyroid cancer cells in vitro and in vivo on orthotopic xenograft tumors. Drug Deliv. 2017;24:670–680.
- Feng L, Dong Z, Tao D, et al. The acidic tumor microenvironment: a target for smart cancer nano-theranostics. Natl Sci Rev. 2018;5:269–286.
- Parker N, Turk MJ, Westrick E, et al. Folate receptor expression in carcinomas and normal tissues determined by a quantitative radioligand binding assay. Anal Biochem. 2005;338:284–293.
- Hilgenbrink AR, Low PS. Folate receptor-mediated drug targeting: from therapeutics to diagnostics. J Pharm Sci. 2005;94:2135–2146.