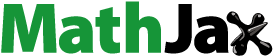
Abstract
Toxoplasma gondii is one of the most widely spread parasitic organisms in the world. T. gondii causes primary, chronic infection and mortality. Major surface antigen 1 is the most abundant tachyzoite surface protein and highly conserved between species and causes strong humoural response. Some studies showed that the peptide sequence of surface antigen has immunity. Therefore, tachyzoite surface antigenic peptide sequence is one of the good candidates for vaccine development. However, conformational information and delivery systems are very important parameters for vaccine development. Computational chemistry which is used as an effective method to perform drug or vaccine design provides important information on structure–activity relationship, biological effects of functional groups, molecular geometry, design of enzyme inhibitors and antagonists. The interaction of immunological peptides with protein systems was carried out by means of computing the free energy of binding using the molecular docking technique. Due to the major histocompatibility complex (MHC), proteins play a substantial role for adaptive immunity, the crystal structure of a MHC class I, which plays a pivotal role in the adaptive branch of the immune system, was preferred for docking calculations. A delivery system based on poly(lactic-co-glycolic acid) (PLGA) nanoparticles and peptide loaded PLGA nanoparticles was prepared in this study to improve the bioavailability of tachyzoite surface antigenic peptide sequence. Double emulsion method (water-in-oil-in-water or w/o/w) was used for synthesis of PLGA and peptide loaded PLGA nanoparticles. The average particle size, polydispersity index and zeta potential values of PLGA and peptide loaded PLGA nanoparticles were measured with zeta-sizer by using dynamic light scattering (DLS) technique. The scanning electron microscope (SEM) (Zeiss Supra 50 V) was used for imagining the peptide loaded PLGA nanoparticles. Cell toxicity of nanoparticles was assayed on AGS (gastric adenocarcinoma) cell line. To evaluate mitochondrial activity of cells and toxicity studies, XTT methods were carried out. In this study, we aimed to obtain specific immunological peptide loaded PLGA nanoparticles and characterize the formation with FTIR, zeta sizer and SEM imaging, and evaluate cytotoxicity and carry out molecular docking calculations of peptide–MHC protein in order to enlight in vivo events as vaccine candidate against T. gondii.
Introduction
Toxoplasmosis, caused by an intracellular protozoan parasite, Toxoplasma gondii, is widespread disease throughout the world. Toxoplasma is considered as one of the most successful eukaryotic pathogens which is of major medical and veterinary importance. The parasite is ubiquitous throughout the world and is estimated to infect approximately half of the world’s population [Citation1]. People can be infected with T. gondii by contact with infected animals, transfusion of contaminated blood or organ, congenital way, and foodborne route. Especially, there is a risk for infection of pregnant women, because the foetus may be stillborn or have some abnormalities such as hydrocephalus, intracranial calcifications, microcephaly, foetal growth retardation, ascites or hepatosplenomegaly [Citation2]. Infection can result in encephalitis in immunocompromised hosts, or serious congenital disease in developing foetus and causes a great economic loss as it may lead to abortion, stillbirth and neonatal loss [Citation3].
Effective vaccines may contribute toward preventing and controlling of disease. There is only one commercial vaccine for toxoplasmosis. The vaccine comprises live tachyzoites of the S48 “incomplete” strain of Toxoplasma gondii, but this vaccine is only approved for to control toxoplasma in sheep. So, there is no effective commercial vaccine for human use against toxoplasmosis. In order to address this problem, numerous vaccination trials against disease have been performed for their immunological effects in animal models, including inactivated and attenuated vaccine, subunit vaccine, genetically engineered vaccine and DNA vaccine [Citation3]. Recent vaccine studies were focused on the immunodominant surface antigens of parasite. Among them, SAG1 protein has important role in host cell invasion and stimulate immune response against parasite. It has role in protection against T. gondii infection and it is shown as important vaccine candidate [Citation4]. However, different sources or isolation, purification methods affect quality and amount of protein [Citation5]. Many researchers have tried to produce recombinant SAG1 but have not succeeded because of the loss of immunoactivity due to insoluble forms and incorrect folding [Citation6]. Therefore, the use of whole protein may not be an effective approach. On the other hand, peptide-based subunit vaccines target an immune response using a minimal microbial component. These vaccines are usually chemically synthesized and have no infectious properties. They are physically more stable and obtained easier and cheaper than conventional vaccines. Also the risk of allergic reaction and autoimmune response is reduced because the pathogen component is at a minimal level [Citation7]. However, because of the small size and physiological degradation risk in body fluids, encapsulation with polymeric nanoparticles is become important approach [Citation8]. Nanoparticles based on polymer have been emerging for clinical and biological applications because of their low toxicity, low immunogenicity and biocompatibility [Citation9]. Encapsulated peptide vaccines presented to the immune systems more effectively, more stable to enzymatic degradation than peptide used alone [Citation7].
Besides formulation of peptides for delivery, it is important to identify the conformational and dynamic information of peptides and know the interactions with immunological proteins of immune systems. Molecular docking calculations were employed to model and predict protein–drug interactions. Docking technique also provides substantial information about prediction of the binding mode of novel drugs, and peptide–protein interactions. Since MHC proteins are notable for adaptive immunity, the crystal structure of antigenic peptide in complex with the murine MHC class I was preferred as the target protein for the docking simulations (). Dynamic light scattering (DLS) technique with zeta-sizer was used to obtain the average particle size, polydispersity index and zeta potential values of poly(lactic-co-glycolic acid) (PLGA) and peptide loaded PLGA nanoparticles (. Zeta potential values of the PLGA and peptide loaded PLGA nanoparticles were measured –21.4 and –14.3, respectively (). The morphology of the peptide loaded PLGA nanoparticles was investigated with spherical morphology by using SEM (. Peptide loaded nanoparticles were first synthesized and characterized and toxicity of this loaded nanoparticles were examined for different concentration on standard cell culture line AGS (gastric adenocarcinoma) with XTT method (). In this study, we aimed to characterize the formation of specific immunological peptide loading PLGA nanoparticles and their formation with FTIR (), zeta sizer and SEM imaging, and to evaluate cytotoxicity and to perform molecular docking computations of peptides against T. gondii as a vaccine candidate.
Figure 3. Schematic hydrogen bonding (yellow) for the docked conformation of active site of title compound with GLU-102, GLU-232 and GLN-96. Refer on-line version for coloured figure.
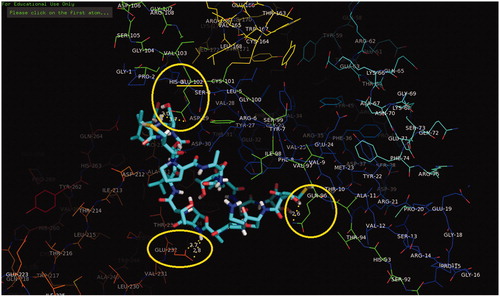
Figure 4. Schematic for the docked conformation of active site of title compound with ARG-6 and TYR-27.
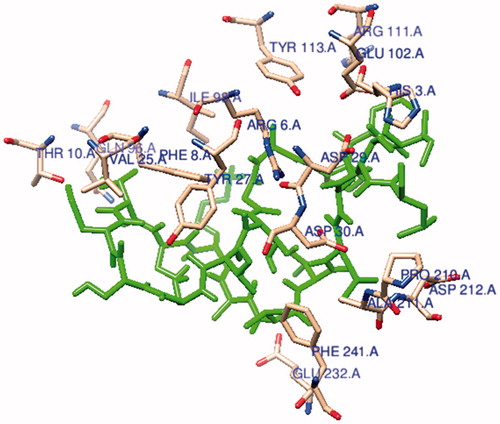
Figure 5. The ligand (Ile-Cys-Pro-Ala-Gly-Thr-Thr-Ser-Ser-Cys-Thr-Ser-Lys-Ala-Val-Thr-Leu-Ser-Ser-Leu) is connected to the active site of MHC protein.
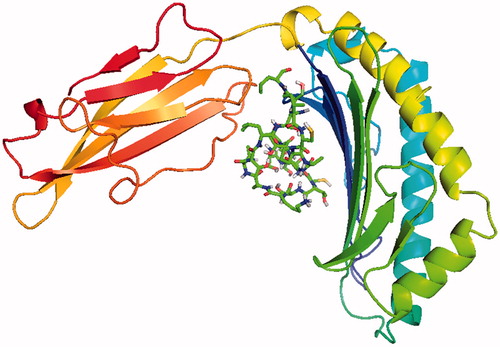
Materials and methods
The peptide sequence of T. gondii was commercially purchased from BioSynthesis Inc. (Lewisville, TX). The peptide sequence was obtained from NH2 – Ile – Cys – Pro – Ala – Gly – Thr – Thr – Ser – Ser – Cys – Thr – Ser – Lys – Ala – Val – Thr – Leu – Ser – Ser – Leu – COOH. The molecular weight of the peptide and isoelectric point (pI) was 1927.21 g/mol and 8.11, respectively. The peptide was hydrophilic and water soluble.
PLGA (lactide:glycolide = 50:50, Mw∼28–34 kDa), and PVA were purchased from Sigma-Aldrich (St. Louis, MO). All the chemicals and solvents were of analytical grade and used without further purification. Ultra-pure water was obtained using the Millipore MilliQ Gradient System.
The initial conformation of the peptide sequence of T. gondii was generated using Hyperchem 8.0 professional program [Citation10] with an ideal geometry of alpha helix (φ = –58° and ψ = –47°) backbone geometry.
Encapsulation with PLGA polymer
Double emulsion method (water-in-oil-in-water or w/o/w) was used for synthesis of PLGA and peptide-loaded PLGA nanoparticles [Citation11]. PLGA was dissolved in dichloromethane (DCM), and peptide in aqueous solution was added. Then, sonication was applied for obtaining homogenized water/oil emulsion. PVA was added in next step and sonicated again. Dichloromethane was evaporated at room temperature and solution including nanoparticles was centrifuged. Pellet was resuspended with distilled water and used for characterization with zeta-sizer ( and ) and FTIR [Citation12] ().
Encapsulation and loading efficiency
UV–vis spectrometer (Shimadzu, Kyoto, Japan) was used for obtaining the standard curve of peptide at 270 nm. Encapsulation and loading efficiency of peptide loaded PLGA nanoparticles were measured. The concentration of free peptide in the supernatant was determined by a UV-Visible Spectrometer via peptide standard curve.
Encapsulation and loading efficiency of peptide were calculated by the following formulas, respectively:
(1)
(1)
(2)
(2)
In vitro release study of peptide loaded PLGA nanoparticles
An in vitro release study was estimated in pH 7.4 PBS (Sigma-Aldrich, St. Louis, MO) by dialysis method. Concisely, 1 mL peptide loaded PLGA nanoparticles were sealed in a cellulose dialysis membrane (MWCO = 10 kDa, Sigma-Aldrich, St. Louis, MO) and incubated in 50 mL of release medium at 37 °C under orbital shaking (150 rpm). At designated intervals, 1 mL samples were removed for analysis and replaced with the same volume of the fresh PBS. The release of peptide was determined as detailed in the following equation via UV–vis spectrometer analysis.
(3)
(3)
Size, size distribution and polydispersity measurements of nanoparticles
The Zetasizer Nano ZS (Malvern Instruments, Malvern, UK), which measures particle size based on photon correlation spectroscopy, was used to determine the size distribution, average size and PdI of nanoparticles at a temperature of 25 °C with a 4.0 mV He–Ne laser (633 nm). In order to remove the impurities from the solutions, before electrophoretic light scattering measurement, each sample was prepared with phosphate-buffered saline (PBS) and filtered with 0.2 μm regenerated cellulose membrane (Sartorius, Göttingen, Germany) filters.
Scanning electron microscopy (SEM) analysis of nanoparticles
The SEM (Zeiss Supra 50 V) was used for imaging the peptide loaded PLGA nanoparticles. Nanoparticle samples were fixed on metal plates and sputtered with gold–palladium mixture at a thickness of 100 Å and observed at an accelerated voltage of 20 kV ().
Transmission electron microscopy (TEM)
Transmission electron microscopy (JEOL, Tokyo, Japan, JEM 1400 Plus) was used for the observation of morphology of peptide loaded PLGA nanoparticles. Briefly, peptide loaded PLGA nanoparticle was diluted with distilled water solution, and placed directly into an ultrasound bath at room temperature for 4 min, then two or three drops of this sample were deposited on a formvar-coated carbon-supported copper grid, and then TEM images were observed at 120 kV ().
Cytotoxicity experiments
An AGS (gastric adenocarcinoma) cell line and XTT method was selected for cytotoxicity studies as described before in literature [Citation13]. Cells were cultured in a DMEM-F12 medium (supplemented with 10% foetal bovine serum, penicillium–streptomycin) at 37 °C with 5% CO2. Cells were trypsinized and collected with centrifugation. The AGS cells were seeded (105 cells/well) in 96-well flat-bottom microplates with 100 μL of medium. They were incubated for 24 h at 37 °C and different concentrations (0, 2, 10, 20, 40, 70 and 100 µg/mL) of the peptide, peptide loaded nanoparticles and empty nanoparticles (without peptide) were added and incubated for another 24 h. The media were then discarded and 100 μL of 2,3-bis-(2-methoxy-4-nitro-5-sulfophenyl)-2H-tetrazolium-5-carboxanilide (XTT) solution in fresh medium was added to the wells at a concentration of 0.5 mg/mL (with 7.5 μg/mL phenazine methosulphate). The plates were incubated for 3 h at 37 °C and optical density was measured at 450 nm with a multi-plate reader (Thermo Labsystems, Philadelphia, PA, Multiskan Ascent 354 Microplate Photometer). Then, the percent viability was calculated with the equation ():
(4)
(4)
Preparation of the rhodamine labelled peptide loaded PLGA nanoparticle for cellular uptake experiments
The powerful fluorescence dye, rhodamine 123 (Sigma, St. Louis, MO, 83689) was dissolved in ultrapure water. To determine, whether peptide loaded PLGA nanoparticles were transferred into the cells, peptide loaded PLGA nanoparticles were labelled with rhodamine 123. Then, rhodamine labelled nanoparticles were incubated for 2 h in dark at the magnetic stirrer. After that cells in 25 cm2 cultured as explained above, exposed the rhodamine labelled peptide loaded nanoparticles and images were taken after 1 h incubation.
Fourier transform infrared (FTIR) spectroscopy analysis
Functional groups of PLGA nanoparticles and peptide loaded PLGA nanoparticles were analysed with IR Prestige21 FT-IR spectrophotometer (Shimadzu, Kyoto, Japan in universal attenuation total reflectance (ATR) mode). The FT-IR spectra were obtained with four scans per sample ranging from 4000 to 750 cm−1 and a resolution of 4 cm−1 [Citation12] ().
Statistical analysis
The data were analysed using the statistical program SPSS version 24.0 (SPSS, Chicago, IL). The mean and standard deviation within samples were calculated for all cases and at least five independent experiments were performed. One-way ANOVA and Mann–Whitney’s test were used to assess the statistical significance value. p < .05 was considered statistically significant.
Results
Molecular docking results
The main purpose of the molecular docking is to identify a ligand that binds to a specific receptor binding site, and the identification of its preferred, energetically most favourable binding pose. Not only all the ligand’s conformations but also the orientation of a ligand relative to its receptor is taken into account in binding pose. Major histocompatibility complex (MHC) proteins play a substantial role for adaptive immunity. The crystal structure of a well-characterized antigenic peptide from ovalbumin in complex with the murine MHC class I H-2 Kb molecule at 2.5-A resolution, which plays a pivotal role in the adaptive branch of the immune system was preferred as the target macromolecule for the docking simulations [Citation14]. Crystal structure of MHC class 1 protein is downloaded from RSCB PDB website (PDB code: 1VAC) [Citation15]. The protein was primarily prepared by removing the Beta-2 microglobulin, chicken ovalbumin and waters. The active site of the protein was defined to include residues of the active site within the grid size of 26 Å – 26 Å – 26 Å. To find an efficient tool for finding binding poses and binding orientations of the ligands, AutoDock-Vina program package was carried out [Citation16]. PyMOL and Chimera molecular graphics system were used for graphical interpretation and representation of docking results [Citation17,Citation18]. RMSD measures the average distance between atoms of two protein or ligand structures via the simple equation which generally used for a ligand, when ligand shows different poses at a particular binding site cluster of a protein. To identify the accuracy of predicting a ligand binding pose, the RMSD value must be smaller than 2 Å. For all obtained conformations, one conformation well bound to the active site were preferred having binding energy with –5.4 kcal/mol with RMSD results in . Ile-Cys-Pro-Ala-Gly-Thr-Thr-Ser-Ser-Cys-Thr-Ser-Lys-Ala-Val-Thr-Leu-Ser-Ser-Leu peptide is connected to the receptor protein primarily by the residues GLU-102, GLU-232, GLN-96 in active site within protein (). Protein–ligand interaction shows that there are three H-bonds with GLU-102: The H atoms in N-terminal and peptide groups of ligands are involved in the interaction with O atoms in COO group of GLU-102 (2.0 Å, 2.5 Å and 3.5 Å). Other H-bond interaction is also identified with GLU-232 (2.7 Å), GLN-96 (2.3 Å, 2.6 Å and 2.9 Å) and THR-10 (2.2 Å) in . In addition to the hydrogen bond interactions, the weak non-covalent interactions are also observed with ARG-6 (2.9 Å, 3.3 Å, 3.4 Å and 3.7 Å), TYR-27 (2.4 Å, 3.5 Å and 3.7 Å) () and PRO-2 (2.8 Å and 2.9 Å) (). These results suggest that the ligand is able to interact MHC protein as seen in which exhibits an important role in the immune system. This may be an important result for designing a new generation vaccine in future.
Table 1. Binding affinity of different poses of the title compound as predicted by AutoDock Vina.
Zeta-sizer results
The average particle size, polydispersity index and zeta potential values of PLGA and peptide loaded PLGA nanoparticles are measured with zeta-sizer by using DLS technique (). As shown in , the size of PLGA nanoparticles was 255.2 nm with the 99% of the particle size distribution. PdI value of nanoparticles in aqueous solution was detected as 0.114, which means that the particles have a very narrow molecular weight distribution. Size and PdI values of peptide loaded PLGA nanoparticles were measured as 231 nm with the 99% of the particle size distribution analysis and 0.173, meaning that the molecular weight distribution of peptide loaded nanoparticles has a wider distribution range than the PLGA nanoparticles, respectively in . Zeta potential values of the PLGA and peptide loaded PLGA nanoparticles were measured –21.4 and –14.3, respectively ().
Table 2. Zeta-sizer results of nanoparticles.
The morphology of the peptide-loaded PLGA nanoparticles was investigated with SEM shown in . As shown in the figure, the peptide-loaded PLGA nanoparticles have spherical morphology. As shown in , the peptide loaded PLGA nanoparticle formation was observed via the TEM images such as SEM images in .
Cytotoxicity results
Cytotoxicity of immunological peptides belonging to T. gondii, peptide loaded nanoparticles and polymeric nanoparticles was examined on standard cell culture line AGS (gastric adenocarcinoma) with XTT method. XTT methods are generally used for toxicity studies and to evaluate mitochondrial activity of cells.
shows the effect of different concentration of peptide (0, 2, 10, 20, 40, 70 and 100 µg/mL) on cells. As shown in , only in higher concentration (100 µg/mL), peptide has slightly toxicity on cells (p < .05). Lower concentrations of peptide do not have lower absorbance than control which depends on viability.
The nanoparticles which do not include peptide were exposed to the cells and XTT results are shown in . PLGA is known as non-toxic polymer and belongs to the FDA-approved polymer family [Citation19]. Our results also showed that PLGA has not any toxic effect on examined concentrations. Even, the cell viability was higher than control group (except 10 µg/mL p > .05).
Peptide loaded nanoparticles were synthesized and characterized in this study and toxicity of this loaded nanoparticles is shown in . The absorbance value of peptide loaded nanoparticles was not lower than control even in concentration of 100 µg/mL.
The overall results of toxicity of peptide, nanoparticles and peptide loaded nanoparticles are shown in as % viability. Cell viability percent was calculated according to control group and was given with standard derivations. The viability of cells was 89.6% in higher concentration of peptide (100 µg/mL) and cell viability percent of all other groups are higher than 90% in .
Table 3. % Viability of cells was exposed peptide, peptide loaded nanoparticles and nanoparticles (±values indicate standard derivations).
Fourier transform infrared spectroscopy results
To determine the absorption bands of peptide loaded-PLGA NPs, and PLGA NPs, the ATR spectrums were recorded. gives the absorption bands of dl-lactide monomer, glycolide monomer, PLA polymer, PLGA co-polymer and peptide loaded PLGA NPs, these characteristic bands agree with those given in the literature [Citation20,Citation21]. Due to stretch of the carbonyl groups, the sharp bands in the region between 1900 cm−1 and 1550 cm−1 are presented at 1760 cm−1, 1750 cm−1, 1750 cm−1, 1761 cm−1 and 1765 cm−1 for dl-lactide, glycolide, PLA, PLGA NPs and peptide loaded PLGA NPs, respectively. The bands between 1300 cm−1 and 1150 cm−1 which relate to the C–O stretching bands, due to asymmetric and symmetric C–C(=O)–O stretching vibrations, are useful in the characterization of esters groups. The C–O ester stretching bands are observed at 1103 cm−1 for both PLGA NPs and peptide loaded PLGA NPs. In literature, the characteristics of ester groups are recorded at 1100 cm−1, 1050 cm−1 and 1090 cm−1 for dl-lactide, glycolide and PLA, respectively [Citation21]. The bands at 999 and 997 cm−1 correspond to C–H bending vibrations. The profile of the spectra presented for PLGA NPs and peptide loaded PLGA NPs in is practically identical, due to the encapsulation. With the aid of FTIR analysis, it is possible to determine all of the reference functional groups (C=O, C–O, CH3, CH2 and CH) in the spectra. The spectra of PLGA NPs and peptide loaded PLGA NPs indicate great similarity and are in agreement with the literature for the PLGA co-polymer, PLA polymer and lactide and glycolide monomers.
Table 4. Absorption bands of PLGA NPs and peptide-loaded PLGA NPs particles.
Loading and encapsulation efficiency and in vitro release study results
As shown in , the standard curve of the peptide was prepared by UV–vis analysis at 270 nm to calculate the encapsulation efficiency of the peptide-loaded PLGA nanoparticles. The encapsulation efficiency of peptide was calculated via EquationEquation (1)(1)
(1) as 81.6%. The amount of peptide in the total peptide-loaded PLGA nanoparticle mass was calculated via EquationEquation (2)
(2)
(2) . The loading efficiency of the peptide-loaded PLGA nanoparticles was determined as 18%.
As shown in , the in vitro release profile of peptide loaded PLGA nanoparticles is depicted. The amount of the free peptide at the different times was measured by UV–Vis spectrometer for 72 h. Finally, it was determined that 34% of peptide was released at the end of the 72 h.
Cellular uptake
shows the cellular uptake of rhodamine labelled peptide loaded PLGA nanoparticles. The florescence-labelled nanoparticles were seen inside the cells. These images were taken after 1 h incubation with nanoparticles and show the nanoparticles can enter inside the cells.
Discussion
PLGA particles were successfully obtained in nanoscale (211.4 nm) according to DLS measurement with zeta-sizer. Both nanoparticles, PLGA and peptide loaded PLGA, were homogenously obtained with 98% distribution. This means nanoparticles are almost identical in size. On the other hand, peptide loaded PLGA nanoparticles have little barely larger empty PLGA nanoparticles. It may be caused by steric effect of functional groups of amino acids in peptide backbone.
Toxicity of peptides, peptide loaded nanoparticles and nanoparticles was measured with mitochondrial activity assay. As given in ISO 10993-5 standards, percentages of cell viability above 80% are considered as non-cytotoxicity; within 80–60% weak toxicity; 60–40% moderate toxicity and below 40% strong cytotoxicity [Citation22]. According to these values, peptides, peptide loaded nanoparticles or nanoparticles have no toxic effect with investigated concentrations on cell line.
The knowledge of dynamic behaviour of drug molecules is important to comprehend biophysical feature of their function. Molecular docking is an essential method to elucidate the predominant binding modes of a drug molecule with a protein. Since MHC proteins play a substantial role for adaptive immunity, the crystal structure of antigenic peptide in complex with the murine MHC class I H-2 Kb molecule was preferred as the target protein for the docking simulations. Due to smaller RMSD value, the accuracy of predicting a ligand binding pose is proven. For all obtained conformations, one conformation which is connected to the receptor protein primarily by the residues GLU-102, GLU-232, GLN-96, well bound to the active site was preferred having binding energy with –5.4 kcal/mol.
Conclusions
As a result of this study, we obtained specific immunological SAG1 peptide loaded PLGA nanoparticles which can be used for vaccine candidate in future. The nanoparticle formation was characterized and proved with various methods. Also for the first time in literature, we showed molecular docking of peptide and MHC molecules and this important information may enlight in vivo events as vaccine for future studies.
Ethical approval
This article does not contain any studies with human participants or animals performed by any of the authors.
Acknowledgements
The authors thank the Turkey General Directorate of Agricultural Research and Policies (Project Number: TAGEM/15/AR-GE/41) for providing peptide used in this study.
Disclosure statement
No potential conflict of interest was reported by the authors.
References
- Liu Q, Singla LD, Zhou H. Vaccines against Toxoplasma gondii: status, challenges and future directions. Hum Vac Immunother. 2012;8:1305–1308.
- Cakir-Koc R, Özdemir B. A global problem of toxoplasmosis. In: The microbiology of central nervous system infections. London (UK): Elsevier; 2018. p. 305–317.
- Hill DE, Chirukandoth S, Dubey J. Biology and epidemiology of Toxoplasma gondii in man and animals. Anim Health Res Rev. 2005;6:41–61.
- Burg JL, Perelman D, Kasper LH, et al. Molecular analysis of the gene encoding the major surface antigen of Toxoplasma gondii. J Immunol. 1988;141:3584–3591.
- Beghetto E, Spadoni A, Bruno L, et al. Chimeric antigens of Toxoplasma gondii: toward standardization of toxoplasmosis serodiagnosis using recombinant products. J Clin Microbiol. 2006;44:2133–2140.
- Makioka A, Suzuki Y, Kobayashi A. Recognition of tachyzoite and bradyzoite antigens of Toxoplasma gondii by infected hosts. Infect Immun. 1991;59:2763–2766.
- Ahmad Fuaad AAH, Jia Z, Zaman M, et al. Polymer-peptide hybrids as a highly immunogenic single-dose nanovaccine. Nanomedicine (Lond). 2014;9:35–43.
- Chang TM. Bioencapsulation in biotechnology. Biomater Artif Cells Immobil Biotechnol. 1993;21:291–297.
- Asadi N, Davaran S, Panahi Y, et al. Application of nanostructured drug delivery systems in immunotherapy of cancer: a review. Artif Cells Nanomed Biotechnol. 2017;45:18–23.
- Froimowitz M. HyperChem: a software package for computational chemistry and molecular modeling. J Comput Chem. 1993;14:934–1013.
- Gorjikhah F, Azizi Jalalian F, Salehi R, et al. Preparation and characterization of PLGA-β-CD polymeric nanoparticles containing methotrexate and evaluation of their effects on T47D cell line. Artif Cells Nanomed Biotechnol. 2017;45:432–440.
- Budama-Kilinc Y, Cakir-Koc R, Horzum-Bayir O. The cytotoxicity, characteristics, and optimization of insulin loaded nanoparticles. Orbital: Electron J Chem. 2017;9:1–6.
- Gharaati-Far N, Tohidkia MR, Dehnad A, et al. Efficiency and cytotoxicity analysis of cationic lipids-mediated gene transfection into AGS gastric cancer cells. Artif Cells Nanomed Biotechnol. 2017. Forthcoming. doi:10.1080/21691401.2017.1355311
- Mitaksov V, Fremont DH. Structural definition of the H-2Kd peptide-binding motif. J Biol Chem. 2006;281:10618–10625.
- Fremont DH, Stura EA, Matsumura M, et al. Crystal structure of an H-2Kb-ovalbumin peptide complex reveals the interplay of primary and secondary anchor positions in the major histocompatibility complex binding groove. Proc Natl Acad Sci. 1995;92:2479–2483.
- Trott O, Olson AJ. AutoDock Vina: improving the speed and accuracy of docking with a new scoring function, efficient optimization, and multithreading. J Comput Chem. 2010;31:455–461.
- DeLano WL. Pymol: an open-source molecular graphics tool. CCP4 Newslett Protein Crystallogr. 2002;40:82–92.
- Pettersen EF, Goddard TD, Huang CC, et al. UCSF Chimera—a visualization system for exploratory research and analysis. J Comput Chem. 2004;25:1605–1612.
- Makadia HK, Siegel SJ. Poly lactic-co-glycolic acid (PLGA) as biodegradable controlled drug delivery carrier. Polymers. 2011;3:1377–1397.
- Erbetta CDAC, Alves RJ, Resende JM, et al. Synthesis and characterization of poly(dl-lactide-co-glycolide) copolymer. J Biomater Nanobiotechnol. 2012;3:208.
- Gumusderelioglu M, Deniz G. Synthesis, characterization and in vitro degradation of poly(dl-lactide)/poly(dl-lactide-co-glycolide) films. Turk J Chem. 1999;23:153–162.
- López-García J, Lehocký M, Humpolíček P, et al. HaCaT keratinocytes response on antimicrobial atelocollagen substrates: extent of cytotoxicity, cell viability and proliferation. JFB. 2014;5:43–57.