Abstract
Currently available chemotherapy is associated with serious side effects, and therefore novel drug delivery systems (DDSs) are required to specifically deliver anticancer drugs to targeted sites. In this study, we evaluated the feasibility of visible light-cured glycol chitosan (GC) hydrogels with controlled release of doxorubicin⋅hydrochloride (DOX⋅HCl) as local DDSs for effective cancer therapy in vivo. The storage modulus of the hydrogel precursor solutions was increased as a function of visible light irradiation time. In addition, the swelling ratio of the hydrogel irradiated for 10 s (GC10/DOX) was greater than in 60 s (GC60/DOX). In vitro release test showed that DOX was rapidly released in GC10/DOX compared with GC60/DOX due to the density of cross-linking. In vitro and in vivo tests including cell viability and measurement of tumor volume showed that the local treatment of GC10/DOX yielded substantially greater antitumor effect compared with that of GC60/DOX. Therefore, the visible light-cured GC hydrogel system may exhibit clinical potential as a local DDS of anticancer drugs with controlled release, by modulating cross-linking density.
Introduction
Systemic drug delivery systems (DDSs), including commercially available and nanomaterial-based chemotherapies for cancer still have side effects such as fatigue, hair loss, infection and diarrhea [Citation1]. Due to deficiency of the specific biodistribution and controlled drug release, the systemic DDSs must be administered multiple times [Citation2]. In addition, the loading capacity of anticancer drugs into nanomaterials may be too low to have anticancer effect [Citation2]. Furthermore, appropriate surface modifications are required, because the cellular uptake rate of nanomaterials depends on the surface characteristics [Citation3].
Compared with systemic DDSs, local DDSs not only reduce the toxicity of normal tissues, but also deliver anticancer drugs to target tissues in a sustained manner, avoiding systemic circulation and improving therapeutic effect [Citation4]. For local DDSs, injectable hydrogel systems, prepared in situ by chemical or physical cross-linking methods, provide sustained and controlled drug delivery to targeted tumor sites and enhance water solubility of anticancer drugs via encapsulation [Citation4].
Unlike physically cross-linked hydrogels, chemical cross-linking of polymer chains facilitates swelling-controlled DDSs, via modulation of pore size in the cross-linked hydrogels leading to control release of anticancer drugs based on the diffusion coefficient of small molecules through the gel network [Citation5]. Therefore, appropriate chemical cross-linking methods are essential for fine-tuning drug release. In this regard, photo-crosslinked hydrogel systems represent alternatives because the drug release may be regulated by light irradiation time. So far, most of the photo-crosslinked hydrogels have been prepared by ultra-violet (UV) or visible irradiation [Citation6]. However, UV light is known to induce DNA damage [Citation7], warranting the need for safe light curing systems. Visible light curing system is cytocompatible and an effective tool for preparation of injectable hydrogels without risk of physical damage [Citation8,Citation9].
Various polymers have been used to prepare injectable hydrogels. The glycol chitosan (GC), one of the water-soluble chitosan derivatives, has been widely used as a carrier for controlled DDS due to its water solubility, biocompatibility and biodegradability [Citation10,Citation11]. In addition, the abundant amine groups of GC chains conjugate with photo-curable groups, resulting in hydrogel formation by ultra-violet (UV) or visible light irradiation [Citation8,Citation9,Citation12].
Therefore, in this study, we prepared an injectable GC hydrogel system crosslinked by visible light irradiation with a controlled release of doxorubicin⋅hydrochloride (DOX⋅HCl) as a local DDS and investigated its antitumor effect on breast cancer in vivo. The in vivo test was carried out using a tumor-bearing mouse animal model.
Experimental section
Materials
Glycol chitosan (GC, ≥60% calculated by titration, crystalline, MW ≅ 585,000 g/mol) and glycidyl methacrylate (GM) were purchased from Sigma-Aldrich (St. Louis, MO). Doxorubicin⋅hydrochloride (DOX HCl) was supplied by Tokyo Chemical Industry Co., Ltd. (Tokyo, Japan). Cellulose membrane tube for purification was purchased from Spectrum Laboratories Inc. (Rancho Dominguez, CA). MCF-7 human breast cancer cell line was obtained by American Type Culture Collection (ATCC, Manassas, VA). All the chemicals were used as received without further purification.
Preparation of visible light-cured GC hydrogels containing DOX⋅HCl
Visible light-cured GC hydrogel was prepared as reported previously () [Citation8,Citation9]. Briefly, GC (0.003 mmol, 1.5 g) was dissolved in water, and the pH was adjusted to 9. After adding GM (0.05 mmol, 7 mg), the mixture was continuously stirred at room temperature for 2 days to generate an epoxy ring opening reaction. The reactant was neutralized and dialyzed (cut off: 20 kDa) in water for 7 days. The purified solution was lyophilized at –90 °C for 7 days. The white solid (1 w/v%) was dissolved in water for injection (Huons, Jechon, Republic of Korea). Riboflavin (12 μM) was added to the solution and shaken continuously for homogenous dispersion. Two types of hydrogel systems containing DOX⋅HCl were prepared for the evaluation of storage/loss moduli, swelling behavior and drug release kinetics. Prior to gelation, DOX⋅HCl (2 mg/mL) was added and the mixture was irradiated with visible light [430–485 nm, 2100 mW/cm2, light-emitting diode (LED) curing light, Foshan Keyuan Medical Equipment Co., Ltd., Guangdong, China] for 10 and 60 s, respectively. The hydrogel systems were designated as GC10/DOX and GC60/DOX, respectively.
Measurement of storage and loss moduli
The storage and loss moduli of hydrogel samples were examined using an AR 2000 EX rheometer (TA instruments, New Castle, DE) set with a cone and plate geometry of 4 cm diameter and 1° cone angle. Both moduli in the plate were measured ranging from 0 to 100 Hz.
Measurement of swelling ratio
The swelling ratio was calculated as the ratio of the swollen weight to the initial weight of the hydrogel samples. After measuring the initial weights of hydrogels, they were immersed in water and extracted at predetermined time intervals. The extracts were washed with water three times and the swollen weights were measured.
In vitro release test of DOX⋅HCl
The release of DOX⋅HCl was examined as reported previously. DOX⋅HCl (3.5 μmol, 2 mg) was added to GM-conjugated GC (1 w/v %) containing riboflavin (12 μM) in phosphate buffered saline (PBS) (pH 7.4, 1 ml), and the mixture was irradiated with visible light for 10 and 60 s to produce hydrogels, respectively. The hydrogels were transferred to a cellulose membrane (MWCO: 3,500 g/mol), and immersed in conical tubes filled with PBS (pH 7.4, 8 ml). The tubes were incubated at 37 °C under 100 rpm. Effluent (2 ml) was extracted at predetermined time intervals (1, 3, 6, 12 and 24 h, and 2, 3, 4, 5, 6 and 7 days), followed by the addition of equal volume of fresh PBS. The amount of DOX⋅HCl in effluents was analyzed using a microplate reader at 480 nm [Citation13].
In vitro cell viability
The in vitro cell viabilities of GC10/DOX and GC60/DOX in MCF-7 cells were evaluated using a CCK-8 agent, compared with untreated (control). GM-conjugated GC (1 wt %) and riboflavin (12 μM) were dissolved in PBS (pH 7.4), and layered on trans-well plates. After irradiation with visible light for 10 and 60 s, the cells (5 × 103 cells/well) were seeded on the hydrogel surfaces and incubated for 1, 3 and 7 days. At the predetermined time intervals, the cell-seeded hydrogels were washed with PBS and treated with the CCK-8 reagent. The hydrogels were incubated for additional 4 h. The optical density was measured by a microplate reader at 450 nm [Citation14].
MCF-7 cancer-bearing mouse model
Animal care, experiments, and euthanasia were performed in accordance with protocols approved by Chonnam National University Animal Research Committee. Adult (6-week-old) male NCRNU nude mice weighing approximately 21 g (N = 3 independent experiments) were purchased from Orient (Seongnam, South Korea). MCF-7 human breast adenocarcinoma cell line was obtained from the American Type Culture Collection (ATCC®HTB-22TM). MCF-7 cells were harvested and suspended in 100 μl of PBS followed by subcutaneous injection into the right flank of each mouse. When tumor size reached about 1 cm in diameter, the GC hydrogels were subcutaneously injected next to the tumor. Animals were imaged and sacrificed eventually.
In vivo evaluation of antitumor effect
The study groups were divided into a tumor control and GC10/DOX-treated tumor. The GC10/DOX (6 mg/kg in 100 μl of water for injection) was subcutaneously administered adjacent to tumor tissue once. The change in tumor volume was monitored for 7 days and compared with that of control. At determined time intervals (days 0, 1, 3, 5 and 7), the tumor volume was calculated using the following formula: V = 0.5 × longest diameter × (shortest diameter)2 [Citation15].
Histology and fluorescence microscopy
Resected tumors were preserved for hematoxylin and eosin (H&E) staining to confirm necrosis followed by fluorescence microscopic assessment to confirm DOX⋅HCl in the tumor. Briefly, samples were fixed in 2% paraformaldehyde (PFA) and flash frozen in optimal cutting temperature (OCT) compound using liquid nitrogen. Frozen samples were cryo-sectioned (10 µm in thickness per slice), observed by fluorescence microscopy, and stained with H&E. Fluorescence imaging was performed on a four filter set of Nikon Eclipse Ti-U inverted microscope equipped with a 100-W halogen lamp, optics, and a 10× Plan Fluor objective lens (Nikon, Seoul, South Korea). Image acquisition and analysis were performed using NIS-Elements Basic Research software (Nikon). All fluorescence images had identical exposure times and normalizations.
Statistical analysis
All quantitative data were expressed as the mean ± standard deviation. Statistical analysis was performed with one-way analysis of variance (ANOVA) using SPSS software (SPSS Inc., Chicago). A value of *p < .05 was considered statistically significant.
Results and discussion
Preparation of GM-conjugated GC
GM was conjugated to GC backbones via epoxy ring opening reaction in alkaline solution to provide functionality for photocuring, resulting in production of methacrylated GC [Citation8,Citation9]. This photocuring system was obtained via initiation of free radicals using a photoinitiator following visible light irradiation [Citation16]. Therefore, it is reasonable that free radicals produced by riboflavin photoinitiator attack the double bond of the methacrylated GC and the two vinyl groups are interconnected by a radical reaction. 1H NMR analysis revealed successful conjugation of GM with GC as our previous reports [Citation8,Citation9] (data not shown). The two doublets at 5.69 ppm and 6.08 ppm were assigned for GM. In addition, the degree of substitution of GM was 10%.
Storage/loss moduli
Generally, photo-cured hydrogels can be prepared by free radicals produced by photoinitiator upon UV or visible light irradiation. UV light carries higher energy than visible light and affects a higher number of chemical bonds [Citation17–19]. Therefore, UV-irradiated cross-linking is more popular than visible light-irradiation in the field of industrial fabrication [Citation17–19]. However, overexposure to UV light causes sunburn and specific types of skin cancer, including malignant melanoma, due to indirect DNA damage by free radicals and oxidative stress [Citation17–19]. These challenges limit the use of UV in biomedical applications, and therefore harmless visible light may outscore UV in biomedicine. Visible light is not only cytocompatible but also the depth of penetration increases with increasing wave length [Citation20]. Photoinitiators are required to develop photocured hydrogels, and riboflavin (vitamin B2) represents an attractive non-toxic alternative to currently used photoinitiators such as Eosin Y, Igarcure 2959 and lithium phosphanate [Citation21]. shows the storage/loss moduli of GC10/DOX and GC60/DOX after visible light irradiation for 10 and 60 s, respectively. Prior to irradiation, a marginal difference between storage and loss moduli was observed in GM-GC (data not shown). On the other hand, the storage modulus of GC10/DOX and GC60/DOX was higher than the loss modulus after visible light irradiation. These results demonstrated that the GM-GC solution gelated because GMs were photo-crosslinked by free radicals after visible light irradiation. In addition, prolonged visible light irradiation resulted in larger storage modulus because the rheological properties of hydrogels were closely related to the degree of cross-linking density [Citation22], contributing to improved elasticity of hydrogels.
Swelling ratio
The swelling ratios of GC10/DOX and GC60/DOX after visible light irradiation were examined at 37 °C for 7 days as shown in . All the hydrogels were rapidly swollen and reached a swelling equilibrium within 5 h. The swelling ratios at 5 h exhibited 1.68 and 1.23-fold higher than those of initial weights, respectively. Furthermore, the swelling ratio of hydrogel irradiated for 10 s was 2.6-fold higher than that of prolonged irradiation for 60 s. These results demonstrated that the swelling behavior has no influence on the loading of small-sized molecules, but only on the degree of cross-linking density [Citation23].
Release behavior of DOX
The swelling behavior of hydrogels is influenced by the density of cross-linking, which contributes to loading efficiency of small molecules. The entrapment efficiency decreased with increase in cross-linking, probably due to differences in the swelling behavior [Citation24]. Furthermore, the swelling ratio may affect the release behavior of small molecules. The release behavior of GC10/DOX and GC60/DOX irradiated for 10 and 60 s is shown in . The hydrogel systems exhibited both initial burst and sustained release of DOX⋅HCl for 7 days. The initial burst was observed within 12 h, and the percentage was 53% and 25%, respectively. Subsequently, DOX⋅HCl was released for 7 days in a sustained manner. Most of the photo-cured hydrogels reported previously are known to exhibit two patterns of drug release behavior: initial burst and sustained release. Immersion of hydrogels in water leads to swelling due to water penetration into the polymer network, swelling of hydrated polymer and drug diffusion from the swollen matrix [Citation25]. Therefore, drugs near the hydrogel surface are rapidly released and those in hydrogel bulk are released in a sustained manner by passive diffusion. Hence, the two release behaviors of DOX⋅HCl on GC10/DOX and GC60/DOX can be attributed to the passive diffusion of the molecules from surface to bulk. Furthermore, the cumulative percentage of DOX⋅HCl in GC10/DOX was higher than in GC60/DOX due to the swelling ratio of hydrogels. Hence, small-molecule drugs in GC10/DOX with a higher swelling ratio were easily released.
In vitro cell viability
The in vitro antitumor effect of GC10/DOX and GC60/DOX on MCF-7 cells was evaluated by examining the in vitro cell viability compared with untreated control (). A gradual decrease in cell viability was observed in both GC/DOX-treated cells. During the 7-day incubation period, the two GC/DOX cells exhibited lower viability than control. Further, GC10/DOX reduced the cell viability more than GC60/DOX, which is attributed to the effect of DOX⋅HCl release. Therefore, it is reasonable to assume that a larger amount of DOX⋅HCl release leads to greater antitumor effect. On day 7, the cell viability of GC10/DOX treatment was 5.26- and 2.09-fold lower than in control and GC60/DOX, respectively. GC has been widely used as a platform for anticancer drug delivery in vitro and in vivo, due to its non-toxicity, low immunogenicity, biodegradability, biocompatibility and water solubility [Citation26]. GC-based carriers exhibit negligible cytotoxicity against several cancer cell lines; however, those containing anticancer drugs such as paclitaxel (PTX), cisplatin, camptothecin (CPT), docetaxel (DTX) and DOX decrease the cell viabilities [Citation27–30]. Depending on the specific delivery of drugs into cancer cells, drug-loaded GC carriers bind to the cell membrane and are internalized via endocytosis [Citation27–30]. Similarly, the local administration of GC10/DOX near tumor tissue may result in specific tissue delivery and apoptosis of cancer cells, and consequently decrease the cell viability. Accordingly, our data demonstrate that GC10/DOX treatment results in superior antitumor effect because the polymer matrix facilitates the effective release and delivery of DOX⋅HCl into tumor tissue.
Figure 5. In vitro viability of MCF-7 cells cultured on GC10/DOX and GC60/DOX by visible light irradiation for 10 or 60 s, as compared with that on control (untreated). The cell viability rate (%) was determined by CCK-8 assay at 1, 3 and 7 days. Error bars represent mean ± SD (n = 3); these experiments were repeated three times.
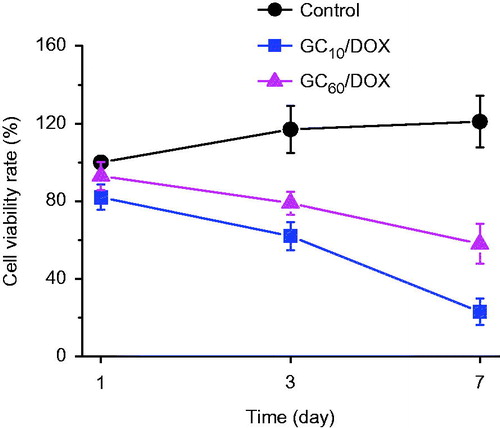
In vivo antitumor effect
As a model drug, DOX, which is a potent and fluorescence-spectrum cytotoxic drug, was used in this study due to its significant antitumor activity against a variety of cancers including human hepatoma, malignant glioma, fibrosarcoma, bone sarcomas, breast and ovarian cancer, carcinomas of the neck and head, hung, prostate, bladder and Ewing’s sarcomas [Citation31–38]. Hence, we investigated the antitumor effect of visible light-cured GC hydrogel system containing DOX⋅HCl using a mouse model carrying MCF-7 tumor-xenograft for 7 days (). GC10/DOX was used for this experiment because it had larger antitumor effect than GC60/DOX in vitro as shown in .
Figure 6. (A) Size appearance of tumor in control and GC10/DOX-treated mouse on days 0, 1, 3, 5 and 7. (B) Comparison of tumor size extracted from control and GC10/DOX-treated mouse at day 7. (C) Tumor volume (mm3) of control and GC10/DOX-treated mice. Error bars represent mean ± SD (n = 3); these experiments were repeated three times. The white scale bars indicate 1 cm.
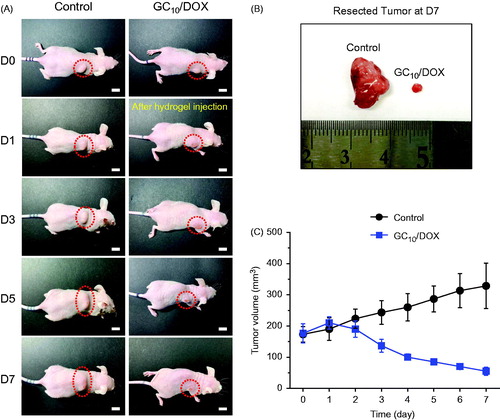
The GC10/DOX was subcutaneously injected next to tumor tissue only once. The appearance of tumor tissues in the subcutaneous site at predetermined time intervals is shown in . Eventually, a decrease in the size was observed in GC10/DOX-treated tumor tissue (). On day 7 after GC10/DOX treatment, the size of tumor tissue treated with GC10/DOX was substantially lower than that of control (). By contrast, the tumor size in control showed a gradual increase. The tumor volume of each sample was examined to analyze the tumor size quantitatively (). On day 0, the volumes of control and tumor tissue treated with GC10/DOX were 173 and 176 mm3, respectively. After 7 days, the tumor volumes were 328 and 55 mm3, respectively. These results demonstrated that local treatment of GC10/DOX improves antitumor effect on MCF-7 cancer in vivo.
Specific delivery and sustained effect of anticancer drugs in target sites are prerequisites for effective chemotherapy in vivo. In this regard, injectable hydrogels have attracted great attention due to their unique characteristics: they are hydrophilic polymers with three-dimensional polymer networks, and therefore, absorb high amounts of water in their pore structures. The pore structure can be easily controlled by modulating the cross-linking density in hydrogel matrix. This structure facilitates the loading of anticancer drugs into the gel matrix and subsequent drug release at a rate dependent on the diffusion coefficient of the molecules. Therefore, the degree of cross-linking density in hydrogels may affect the effectiveness of chemotherapy, because controlled release of anticancer drugs can be fine-tuned by porosity, which is consistent with the results of storage/loss modulus, in vitro drug release and cell viability. Consequently, these results may be explained by the compact three-dimensional network of the modified hybrid hydrogels, which slowly release the drug over a prolonged period, resulting in superior antitumor effectiveness at a relatively low non-toxic concentration as shown in [Citation39].
Figure 7. Schematic illustration showing possible mechanism of GC10/DOX on the improvement of cancer therapy. GC10/DOX was locally injected next to tumor.
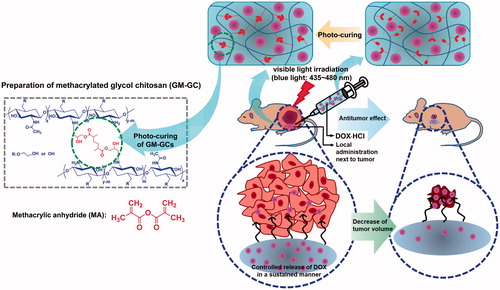
Changes in the body weight of control and GC10/DOX-treated mice were examined for 7 days (). The body weight of GC10/DOX-treated mice was smaller than that of control, which indicates the antitumor effect of GC10/DOX. As shown by the in vitro cell viability assay, GC has no cytotoxicity on MCF-7 breast cancer cell line [Citation40], and the decrease in the body weight is attributed to the antitumor effect of DOX⋅HCl.
Histological and fluorescent evaluations
shows H&E stained and fluorescent images of untreated and GC10/DOX-treated tumor tissues after 7 days. As shown in few necrotic areas were observed in untreated tumor tissue, resulting in rapid tumor growth. In GC10/DOX-treated tumor tissue, together with shrinkage of tumor tissue, most of the tissue except for the red-stained area turned necrotic due to the apoptosis of cancer cells following exposure to DOX⋅HCl (). This finding was supported by the results of fluorescent images (). No DOX was observed in untreated tumor tissue. On the other hand, the accumulation of DOX⋅HCl in GC10/DOX-treated sample was observed in red color. Furthermore, DOX⋅HCl was remarkably distributed in the necrotic area. Therefore, these results suggested that DOX⋅HCl in GC hydrogel was delivered to the tumor tissue, resulting in necrosis.
Conclusions
Herein, we reported the preparation of injectable GC hydrogel systems with a controlled release of DOX⋅HCl using visible light irradiation and evaluated its antitumor effect in vivo. The photo-cured hydrogel systems control the elasticity and swelling ratio as a function of curing time, as the cross-linking density is controlled by the irradiation time, resulting in a controlled and sustained release of DOX⋅HCl. The results of in vivo animal test, H&E-staining and fluorescence images indicated antitumor effect of a GC10/DOX hydrogel system administered locally next to a tumor tissue. Therefore, the visible light-cured hydrogel systems may represent a potential carrier of anticancer drugs for the local DDS resulting in improved cancer therapy.
Disclosure statement
The authors declare that there are no conflicts of interest. The authors are responsible for the conduction of experiments and writing of the paper.
Additional information
Funding
References
- Wolinsky JB, Newman DJ, Grinstaff MW, et al. Local drug delivery strategies for cancer treatment: gels, nanoparticles, polymeric film, rods, and wfers. J Control Release. 2012;159:14–26.
- Lee J-Y, Chung S-J, Cho H-J, et al. Phenylboronic acid-decorated chondroitin sulfate A-based theranostic nanoparticles for enhanced tumor targeting and penetration. Adv Funct Mater. 2015;25:3705–3717.
- Zhong Y, Meng F, Deng C, et al. Ligand-directed active tumor-targeting polymeric nanoparticles for cancer chemotherapy. Biomacromolecules. 2014;15:1955–1969.
- Norouzi M, Nazari B, Miller DW. Injectable hydrogel-based drug delivery systems for local cancer therapy. Drug Discov Today. 2016;1:1835–1849.
- Mahkam M, Doostie L. The relation between swelling properties and crosslinking of hydrogels designed for colon-specific drug delivery. Drug Deliv. 2005;12:343–347.
- Bastiancich C, Danhier P, Préat V, et al. Anticancer drug-loaded hydrogels as drug delivery systems for the local treatment of glioblastoma. J Control Release. 2016;243:29–42.
- D’Orazio J, Jarrett S, Amaro-Ortiz A, et al. UV radiation and the skin. Int J Mol Sci. 2013;14:12222–12248.
- Yang DH, Seo DI, Lee D-W, et al. Preparation and evaluation of visible light cured glycol chitosan hydrogel dressing containing dual growth factors for accelerated wound healing. J Ind Eng Chem. 2017;53:360–370.
- Yoon S-J, Hyun H, Lee D-W, et al. Visible light-cured glycol chitosan hydrogel containing a beta-cyclodextrin-curcumin inclusion complex improves wound healing in vivo. Molecules 2017;22:1513.
- Yoon HY, Son S, Lee SJ, et al. Glycol chitosan nanoparticles as specialized cancer therapeutic vehicles: sequential delivery of doxorubicin and Bcl-2 siRNA. Sci Rep. 2014;4:6878.
- Lee SJ, Min HS, Ku SH, et al. Tumor-targeting glycol chitosan nanoparticles as a platform delivery carrier in cancer diagnosis and therapy. Nanomedicine (Lond). 2014;9:1697–1713.
- Cho IS, Cho MO, Li Z, et al. Synthesis and characterization of a new photo-crosslinkable glycol chitosan thermogel for biomedical applications. Carbohy Polym. 2016;144:59–67.
- Zhang W, Zhou X, Liu T, et al. Supramolecular hydrogels co-loaded with camptothecin and doxorubicin for sustainedly synergistic tumor therapy. J Mater Chem B. 2015;3:2127–2136.
- Cao W, Ma SL, Tang J, et al. A combined treatment TNF-α/doxorubicin alleviates the resistance of MCF-7/Adr cells to cytotoxic treatment. Biochim Biophys Acta (BBA). 2006;1763:182–187.
- Reis-Sobreiro M, Roué G, Moros A, et al. Lipid raft-mediated Akt signaling as a therapeutic target in mantle cell lymphoma. Blood Cancer J. 2013;3:e118.
- Mironi-Harpaz I, Wang DY, Venkatraman S, et al. Photopolymerization of cell-encapsulating hydrogels: crosslinking efficiency versus cytotoxicity. Acta Biomater. 2012;8:1838–1848.
- Atsumi T, Iwakura I, Fujisawa S, et al. Free-radical generation by camphorquinone and other photosensitizers and their cytotoxicity. J Dent Res. 2000;9:75–83.
- Fedorovich NE, Oudshoorn MH, van Geemen D, et al. The effect of photopolymerization on stem cells embedded in hydrogels. Biomaterials. 2009;30:344–353.
- Di Z, Yoshihiro I. Visible light-curable polymers for biomedical applications. Sci China Chem. 2014;57:510–521.
- Wang J, Stanic S, Altun AA, et al. A highly efficient waterborne photoinitiator for visible-light-induced three-dimensional printing of hydrogels. Chem Commun (Camb). 2018;54:920–923.
- Batchelor RR, Kwandou G, Spicer PT, et al. (-)Riboflavin (vitamin B2) and flavin mononucleotide as visible light photo initiators in the thiol-ene polymerization of PEG-based hydrogels. Polym Chem. 2017;8:980–984.
- Weng L, Chen X, Chen W. Rheological characterization of in situ crosslinkable hydrogels formulated from oxidized dextran and N-carboxyethyl chitosan. Biomacromolecules. 2007;8:1109–1115.
- Cheng C, Zhang X, Meng Y, et al. Development of a dual drug-loaded hydrogel delivery system for enhanced cancer therapy: in situ formation, degradation and synergistic antitumor efficiency. J Mater Chem B. 2017;5:8487–8497.
- Deepa G, Thulasidasan AKT, Anto RJ, et al. Cross-linked acrylic hydrogel for the controlled delivery of hydrophobic drugs in cancer therapy. Int J Nanomed. 2012;7:4077–4088.
- Sriamornsak P, Thirawong N, Weerapol Y. Swelling and erosion of pectin matrix tablets and their impact on drug release behavior. Eur J Pharm Biopharm. 2007;67:211–219.
- Riva R, Ragelle H, des Rieux A, et al. Chitosan and chitosan derivatives in drug delivery and tissue engineering. In: Jayakumar R, Prabaharan M, Muzzarelli R, editors. Chitosan for Biomaterials II. Advances in Polymer Science. Vol. 244. Berlin, Heidelberg: Springer. p. 19–44.
- Koo H, Min KH, Lee SC, et al. Enhanced drug-loading and therapeutic efficacy of hydrotropic oligomer-conjugated glycol chitosan nanoparticles for tumor-targeted paclitaxel delivery. J Control Release. 2013;172:823–831.
- Kim JH, Kim YS, Park K, et al. Antitumor efficacy of cisplatin-loaded glycol chitosan nanoparticles in tumor-bearing mice. J Control Release. 2008;127:41–49.
- Min KH, Park K, Kim YS, et al. Hydrophobically modified glycol chitosan nanoparticles-encapsulated camptothecin enhance the drug stability and tumor targeting in cancer therapy. J Control Release. 2008;127:208–218.
- Hwang HY, Kim IS, Kwon IC, et al. Tumor targetability and antitumor effect of docetaxel-loaded hydrophobically modified glycol chitosan nanoparticles. J Control Release. 2008;128:23–31.
- Yoo HS, Lee KH, Oh JE, et al. In vitro and in vivo anti-tumor activities of nanoparticles based on doxorubicin-PLGA conjugates. J Control Release. 2000;68:419–431.
- Arai T, Joki T, Akiyama M, et al. Novel drug delivery system using thermoreversible gelation polymer for malignant glioma. J Neurooncol. 2006;77:9–15.
- Lesniak MS, Upadhyay U, Goodwin R, et al. Local delivery of doxorubicin for the treatment of malignant brain tumors in rats. Anticancer Res. 2005;25:3825–3831.
- Konishi M, Tabata Y, Kariya M, et al. In vivo anti-tumor effect of dual release of cisplatin and Adriamycin from biodegradable gelatin hydrogel. J Control Release. 2005;103:7–19.
- Wong HL, Bendayan R, Rauth AM, et al. Simultaneous delivery of doxorubicin and GG918 (Elacridar) by new polymer-lipid hybrid nanoparticles (PLN) for enhanced treatment of multidrug-resistant breast cancer. J Control Release. 2006;116:275–284.
- Minkoa T, Kopečkováab P, Pozharovc V, et al. HPMA copolymer bound Adriamycin overcomes MDR1 gene encoded resistance in a human ovarian carcinoma cell line. J Control Release. 1998;54:223–233.
- Benjamin RS, Riggs CE, Jr, Bachur NR. Pharmacokinetics and metabolism of Adriamycin in man. Clin Pharmacol Ther. 1973;14:592–600.
- Lin R, Shi Ng L, Wang CH. In vitro study of anticancer drug doxorubicin in PLGA-based microparticles. Biomaterials. 2005;26:4476–4485.
- Ju C, Sun J, Zi P, et al. Thermosensitive micelles-hydrogel hybrid system based on poloxamer 407 for localized delivery of paclitaxel. J Pharm Sci. 2013;102:2707–2717.
- Wang Y, Qin F, Tan H, et al. pH-responsive glycol chitosan-cross-linked carboxymethyl-β-clodextrin nanoparticles for controlled release of anticancer drugs. Int J Nanomed. 2015;10:7359–7370.