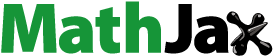
Abstract
As novel amphiphilic materials, six uncharged alkyl rhamnosides incorporating various alkyl chain and one rhamnose amine quaternary ammonium salt were successfully synthesized in this study. Their amphiphilic properties (HLB and CMC), antimicrobial and anti-biofilm activity against S. aureus and P. aeruginosa were investigated. Differentially regulated proteins and pathways were identified by comparative proteomics research to first give a sight on how alkyl rhamnosides performed the anti-biofilm activity at protein and pathway levels. Among the uncharged alkyl rhamnosides, dodecyl rhamnoside and octyl rhamnoside showed the best antimicrobial and anti-biofilm ability against S. aureus and against P. aeruginosa, respectively. Interestingly, the relationships between amphiphilic properties or MIC with anti-biofilm activity were first established. Uncharged alkyl rhamnoside with an optimized HLB value of 5.0 had both the strongest antibacterial and anti-biofilm activity against S. aureus, and MIC was the maximum biofilm inhibitory concentration for all alkyl rhamnosides. Alkyl rhamnosides have a significant overall regulatory effect on the proteomics and pathways of bacterial biofilms, including energy production, substrates transportation, signal transduction, key molecules balance, and so on. These amphiphilic materials have a great potential to be used as additives in pharmaceutic, cosmetic, food industry, hospital and in other non-medical fields.
Introduction
Although bacteria are commonly viewed as solitary life forms, these organisms are more typically colonial creatures. In response to certain environmental parameters, bacteria will differentiate from an independent free-living mode of growth and take up an interdependent surface-attached existence. These surface-attached microbial communities are known as biofilms [Citation1]. In flowing systems where nutrients are available, biofilms can develop into elaborate three-dimensional structures. The development of biofilms is a process involved both a quorum of cells and multicellular behaviour [Citation2], which has been linked to the emergence of a variety of opportunistic human pathogens [Citation3]. In addition, bacterial biofilms, resistant to antibiotics [Citation4], also play a critical role in in many fields, such as clinical infection such as patient's infections and device-related infections, food contamination, and marine biofouling, etc. [Citation5].
Inducing dispersal of harmful biofilms is an appealing strategy [Citation6]. Rhamnolipid, naturally produced by P. aeruginosa, induces detachment of P. aeruginosa cells from biofilms and disperse biofilms of other species [Citation7]. Besides, rhamnolipid also causes biofilm dispersal in vivo and promotes easy removal of bacteria by the host or traditional antibiotics [Citation8]. However, rhamnolipid as a potent virulence factor has detrimental effects on eukaryotic cells, lysing red blood cells and causing necrotic cell death in polymorphonuclear leukocytes and macrophages [Citation9], which limits the widespread use of rhamnolipids, especially in clinical applications. Besides, rhamnolipids extracted from bacteria are mixture that is difficult to be isolated and purified on a large scale, hindering the research on the relationship between structure and activity of rhamnolipid and its mechanism of action. Therefore, finding an easy-to-synthesize analogue with accurate structure is an attractive research direction.
We designed a simple molecule of amphipathic structural material, rhamnoside, which has the same hydrophilic group, rhamnose, and the typical hydrophobic group, fatty alcohol. It is hoped to have the same desirable antibacterial and biofilm effect. In our study, a series of uncharged alkyl rhamnosides with different lengths of fatty chains, as well as positive charged alkyl rhamnoside were synthesized. Hydrophilic–lipophilic balance (HLB) and critical micelle concentration (CMC) were determined which aimed at establishing the relationship between amphiphilic molecular structure parameters and antibacterial or anti-biofilm activity. Gram-positive S. aureus (ATCC 29213) and Gram-negative P. aeruginosa (ATCC 27853) were selected as model organisms. Antimicrobial and anti-biofilm ability of alkyl rhamnosides identified by minimum inhibitory concentration (MIC), biofilm formation inhibition as well as pre-formed biofilm destruction were investigated by broth dilution method, crystal violet staining method and confocal laser scanning microscopy (CLSM) assays, respectively. More meaningfully, we led a label-free comparative proteomics study to find the inner mechanisms of alkyl rhamnosides’ anti-biofilm activity against S. aureus and P. aeruginosa.
Materials and methods
Materials
Reagents and solvents were purchased from common commercial suppliers and were used without further purification. S. aureus (ATCC 29213) and P. aeruginosa (ATCC 27853) were obtained from the American Type Culture Collection (Manassas, VA). Con A-FITC was purchased from Sigma-Aldrich (St. Louis, MO). Hoechst 33258 was obtained from Gibco, Invitrogen (Ontario, CA). Mueller-Hinton Broth (MHB) was purchased from XinHuizeao Science and Technology Co., Ltd. (Beijing, China). Minute Detergent-Free Protein Extraction Kit YD-016 was purchased from Invent Biotechnologies, Inc. (Eden Prairie, MN). Bacterial Protein Extraction Kit C600596-0001 was purchased from Sangon Biotechnology Co., Ltd. (Shanghai, China).
Synthesize of nonionic alkyl rhamnosides
The synthesis of nonionic alkyl rhamnosides via the Fischer type of glycosylation reaction between unprotected, unactivated l-rhamnose and fatty alcohols was promoted by triflic acid immobilized on silica gel (TfOH-SiO2) as described by Yu and Chen with some modifications [Citation10]. First, TfOH (3.06 g, 20 mmol) was added to a suspension of silica gel (10 g, mesh no. 300-400) in ether (40 mL). The mixture was stirred magnetically for 30 min at room temperature. Ether was removed under reduced pressure and the residue was heated at 100 °C for 24 h under vacuum to afford TfOH-SiO2 (2 mmol/g) as a free-flowing powder [Citation11]. Thin-layer chromatography (TLC) was performed on precoated Merck silica gel 60 F254 plates (Merck, Kenilworth, NJ) using CH2Cl2:MeOH (10:1; v/v) as developing solvent and visualized by 10% (v/v) H2SO4 in ethanol. Column chromatography was performed on silica gel (200–300 mesh, Qingdao, China). Solutions were concentrated at a temperature <50 °C under diminished pressure.
l-Rhamnose (2.0 g, 10.98 mmol) was dispersed in n-hexanol (5.52 mL, 43.92 mmol), n-octanol (6.89 mL, 43.92 mmol), 5-nonanol (7.63 mL, 43.92 mmol), n-decanol (8.39 mL, 43.92 mmol), n-dodecanol (9.98 mL, 43.92 mmol) and n-tetradecanol (11.28 mL, 43.92 mmol), respectively. After stirred at 80 °C for several minutes, the above reaction mixtures were then mixed with TfOH-SiO2 (165 mg, 2 mmol/g), respectively. Stirring was continued for another 5 h until all solids dissolved. At this point, TLC showed complete conversion of the starting l-rhamnose to a faster running point. Then, the reaction mixture was rapidly transferred to a silica gel column (inner diameter of 3 cm, length 20 cm) and excess fatty alcohol was eluted with CH2Cl2 (150 mL) followed by elution with CH2Cl2/MeOH (20:1, v/v) to obtain the desired alkyl rhamnoside. The diagram of this glycosylation reaction is shown in .
Synthesize of N-dodecyl rhamnose amine quaternary ammonium salt [Citation12]
l-Rhamnose (2.55 g, 14 mmol) and lauryl amine (3.87 g, 21 mmol) were added into methanol (60 mL) and solid gradually dissolved. After stirred magnetically for 6–8 h at 25–30 °C, the mixture turned from a clear to a white precipitate. When the temperature was raised to 50–55 °C, the reaction system became clear again. After reacted for 2–3 h, TLC (developing agent: CH2Cl2–methanol–triethylamine =10:1:0.05, v/v/v) was performed to show complete conversion of the starting l-rhamnose to a faster running point. Stop heating, cooled down statically, white precipitate appeared again. After suction filtration, the mixture was washed three times with acetone/n-hexane (7:3, v/v) to remove the excess lauryl amine and collect the crude product. The crude product was recrystallized by ethanol to afford the desired white needle crystals (pure N-dodecyl rhamnose amine).
N-dodecyl rhamnose amine (1.65 g, 5 mmol) and CH3I (1.3 mL, 20 mmol) were dissolved in ethanol (70 mL). Then, NaHCO3 (0.50 g, 6 mmol) was added into the mixture. The mixture was stirred magnetically for 12 h at 50–55 °C. At this point, TLC showed complete conversion of the starting N-dodecyl rhamnose amine to a slower running point (nearly at the origin). After filtration, ethanol was removed under reduced pressure, and a crude product was obtained. Dichloromethane (30 mL) was added dropwise into the crude product, and a white powdery solid (inorganic salt) was precipitated out. Then, the mixture was left to stand overnight. After filtration, dichloromethane was distilled off under reduced pressure, and a sticky brown syrup-like pure 12N+-Rha was synthesized. The scheme for this reaction is shown in .
Structural characterization of the alkyl rhamnosides
The NMR spectra were recorded on a Bruker Avance III 400 MHz instrument (400 MHz for 1H and 100 MHz for 13C). Chemical shifts are reported in δ values. For 1H NMR spectra (CDCl3), the residual solvent peak was used as the internal reference (7.26 ppm), whereas the central solvent peak as the reference (77.03 ppm) for 13C NMR spectra (CDCl3). Data are reported as follows: chemical shift, multiplicity (s = singlet, d = doublet, t = triplet, q = quartet, m = multiplet, br = broad singlet), coupling constants (Hz) and integration.
Mass spectra (ESI-MS) were recorded on Waters SQD system.
Calculation of HLB values by McGowan’s atomic volume method
McGowan’s atomic volume method was used to calculate the HLB values of the synthesized alkyl rhamnosides in our study [Citation13]. The HLB values of different alkyl rhamnosides were calculated as:
(1)
(1)
Measurement of critical micelle concentration
Determination of the CMC of uncharged alkyl rhamnosides by pyrene fluorescence probe method
Uncharged alkyl rhamnoside in acetone with various concentrations was transferred to a series of glass tubes and the acetone was evaporated under a slow flow of nitrogen gas. Then, 10 mL of water-saturated solution of pyrene (6.0 × 10−7 mol/l) was added into each glass tube to obtain different concentration of alkyl rhamnosides (1 × 10−5 to 4 mg/mL). After incubation away from light for 24 h at 37 ± 0.5 °C, the fluorescence spectra of pyrene were detected using a Shimadzu fluorescence spectrophotometer (RF-5301, Kyoto, Japan) with an emission wavelength of 392 nm. The peak intensity ratio I335/I333 against the logarithm of concentrations was plotted.
Determination of the CMC of N-dodecyl rhamnose amine quaternary ammonium salt by conductivity method
Different concentrations of 12N+-Rha solution (0.1–5 mg/mL) and KCl calibration solution (0.01 mmol/L) were accurately prepared. The solutions were placed in a constant temperature water bath (25 ± 0.1 °C) for 30 min. After the conductivity electrode was calibrated by KCl calibration solution, the conductivity of 12N+-Rha solutions was measured by a DDSJ-308F Conductivity Meter (INESA Instrument, Shanghai, China) from low concentrations to high concentrations. Each measurement was repeated 5–10 times. The CMCs were determined by the intersection of the two straight lines with different slopes in the electrical conductivity curves.
Anti-bacterial activity: minimum inhibitory concentration
The preparation of stock solutions: in brief, seven alkyl rhamnosides samples were dissolved in PBS to form stock solutions (20 μmol/mL). With good water solubility, 6C-Rha and 12N+-Rha were dissolved in PBS to form a clear solution, respectively. The remaining five samples were first dissolved in five parts (volume) dimethyl sulphoxide (DMSO), and then diluted with 95 parts (volume) PBS to meet the final concentration of 20 μmol/mL (5% DMSO in PBS took no effect on bacterial growth). After treatment of ultrasonic and vortex, 8C-Rha and 4,4C-Rha were well dissolved to form clear solutions, but 10C-Rha, 12C-Rha and 14C-Rha were still suspended. Therefore, the stock solutions were fully vortexed and were added quickly when used in order to ensure the consistency of concentration.
The MIC, defined as the minimal concentration of antibiotic sufficient to prevent visible growth of the bacteria, was determined using the broth dilution technique as recommended by the National Committee for Clinical Laboratory Standards. Laboratory strains of S. aureus (ATCC 29213) and P. aeruginosa (ATCC 27853) were applied as reference strains for quality control as well as test organisms, respectively. In brief, 100 μL of bacterial suspension of ∼5 × 105 CFU/mL diluted in MHB was dispensed into a 96-well microplate. Then, 100 μL of alkyl rhamnoside stock solution (20 μmol/mL) was transferred into the first well in each row, respectively, and serially diluted by twofold method to meet the final concentration range from 19.5 nmol/mL to 10 μmol/mL. Bacterial culture without alkyl rhamnosides and blank growth media was used as positive control and negative control, respectively. After incubation for 18 h at 37 °C, the cultures were inspected for bacterial growth. The endpoint MIC is the lowest concentration of the compound at which the test strain does not demonstrate visible growth. The MICs of alkyl rhamnosides for each bacterial strain were recorded and were expressed in nmol/mL.
Anti-adhesive (biofilm formation inhibition) assay
The anti-adhesive activity of alkyl rhamnosides against S. aureus (ATCC 29213) and P. aeruginosa (ATCC 27853) was evaluated by growing cells in 96-well plates [Citation14,Citation15]. In brief, 100 μL of bacterial suspension of ∼5 × 105 CFU/mL diluted in MHB was dispensed into a 96-well microplate. The outer columns and rows of a 96-wells plate were filled with sterile PBS to prevent evaporation of solution in the central wells. Then, 100 μL of alkyl rhamnoside stock solution (20 μmol/mL) was transferred into the first well in each row, respectively, and serially diluted by twofold method to meet the final concentration range from 19.5 nmol/mL to 10 μmol/mL. For quantitative assay, 4–8 replicate wells were used for each treatment.
After 18 h incubation for S. aureus and 12 h incubation for P. aeruginosa at 37 °C without shaking, wells were emptied and the planktonic cells were discarded by washing the wells three times with PBS. Adhesion of cells and biofilm formation were quantified by using the crystal violet assay [Citation16]. The adherent microorganisms were fixed with 200 μL of methanol per well, and after 15 min, the plates were emptied and left to dry. Then, the wells were stained with 200 μL of crystal violet methanol solution (0.1%, w/v) for 20 min at room temperature. Excess dye was rinsed out by placing the plate under running tap water. Subsequently, the plates were air-dried, and the dye bound to the adherent microorganisms was resolubilized with 200 μL of acetic acid in water (33%, v/v) per well. The absorbance (A) of each well was measured in a microplate reader (Multiskan Mk3, Thermo Scientific, Waltham, MA) at 560 nm using 33% (w/v) acetic acid in water as the blank. The medium without the alkyl rhamnosides was used as non-treated control. The results were expressed in terms of percentage of inhibition compared to alkyl rhamnosides-untreated wells. The percentage of inhibition at different alkyl rhamnoside concentrations for each microorganism was calculated as:
(2)
(2)
Alkyl rhamnosides-mediated disruption of pre-formed biofilms
Pre-formed biofilm disassembly ability of alkyl rhamnosides was studied [Citation15]. Briefly, 100 μL of S. aureus and P. aeruginosa suspensions (approximately 5 × 105 CFU/mL) were dispensed into the wells of flat bottom 96-well plates, respectively. For quantitative assay, 4–8 replicate wells were applied for each treatment. Plates were incubated for 24 h at 37 °C without shaking. After incubation, unattached bacteria were removed and the medium was replaced with 100 μL of MHB containing various concentrations of alkyl rhamnosides (19.5–10 × 103 nmol/mL). These plates were further incubated for 12 h at 37 °C without shaking. At the end of incubation, the suspension in the wells was emptied and the planktonic cells were discarded by washing the wells three times with PBS. Adhesion of cells and biofilm formation were quantified by using the crystal violet assay as mentioned earlier. The medium without the alkyl rhamnosides was used as non-treated control. The percentage of inhibition at different alkyl rhamnosides concentrations for each microorganism was calculated as:
(3)
(3)
Confocal laser scanning microscopy study on anti-biofilm activity of alkyl rhamnosides
S. aureus and P. aeruginosa suspensions (∼5 × 105 CFU/mL, 1 mL) were dispensed into the 12-well plates containing pre-sterilized microscopic glass slides as surfaces for biofilm formation [Citation17]. The plates were incubated for 24 h at 37 °C without shaking to form biofilms. After incubation, wells were emptied and the planktonic cells were discarded by washing the wells three times with PBS. Then, MHB medium with 12C-Rha (312.5 nmol/mL, 1 mL) and 12N+-Rha (1250 nmol/mL, 1 mL) was added into the wells containing S. aureus and P. aeruginosa, respectively. MHB medium without the alkyl rhamnosides was assigned as non-treated control. The 12-well plates were further incubated for 12 h at 37 °C to investigate the effect of alkyl rhamnosides on the pre-formed biofilm of S. aureus and P. aeruginosa. Thereafter, the microscopic glass slides with untreated and treated biofilms were stained with Con A-FITC (Con A-FITC binds to the sugar residues in biofilm polysaccharide and stains them green) for 30 min at 4 °C, immobilized by 2.5% glutaraldehyde for 2 h at 4 °C, and then stained with Hoechst 33258 (Hoechst 33258, a nucleic acid staining dye stains the bacterial cells blue) for 20 min at room temperature. These microscopic glass slides were then examined under an A1R plus STORM confocal microscope system (Nikon, Tokyo, Japan). All images were recorded with a 40× lens. Laser beams with 488 nm and 405 nm excitation wavelengths were used for FITC and Hoechst 33258 imaging, respectively. A representative image is presented for each group in Results section.
Proteomics research of 12C-Rha and 12N+-Rha to biofilms
S. aureus suspension (∼5 × 105 CFU/mL) was added in 90 mm Petri dish and cultivated for 24 h at 37 °C to form biofilms. Then, unattached bacteria were removed and the medium was replaced with fresh blank MHB and MHB containing 12C-Rha (1/4 MIC), respectively. For quantitative assay, three replicate Petri dishes were used for each group. After another 12 h incubation at 37 °C, biofilms were collected with cell scraper. The proteome of S. aureus biofilms were extracted by the Minute Detergent-Free Protein Extraction Kit YD-016, identified by LC–MS, and finally analysed by Beijing BangFei Bioscience Co., Ltd. (Beijing, China). On the other hand, P. aeruginosa biofilms response to 12N+-Rha (1/4 MIC) was performed the same as mentioned above except using Bacterial Protein Extraction Kit C600596-0001.
Results
Synthesis and identification of alkyl rhamnosides
TfOH-SiO2 as an environmentally friendly solid acid catalyst with strong acidic sites was used to promote the glycosylation reaction between l-rhamnose and fatty alcohols. The glycosylation condition of using 4.0 equiv. fatty alcohols and 3% equiv. TfOH-SiO2 at 80 °C was considered as the optimal protocol for the synthesis of uncharged alkyl rhamnosides [Citation10]. The characteristics and yields of different uncharged alkyl rhamnosides are shown in .
Table 1. The characteristics and yields of the alkyl rhamnosides.
1H NMR spectrum of the alkyl rhamnosides (not shown in our study) showed a new characteristic peak at 4.74–4.84 ppm for the methenyl protons of rhamnose adjacent glycosidic bond (Rha-1′). The peak found around at 3.34–3.55 ppm, 3.57–3.69 ppm and 3.70–3.94 ppm in the 1H NMR spectrum assigned the methenyl proton of rhamnose (Rha-2′, Rha-3′, Rha-4′ and Rha-5′) and the peak around 0.88–0.90 ppm, 1.20–1.38 ppm, 1.41–1.64 ppm assigned the alkyl proton of fatty alcohol. The total number of C signals in the 13C NMR spectrum was the same as the number of carbons in the alkyl rhamnoside formula. All those spectra of NMR clearly indicated that fatty alcohol successfully conjugated with rhamnose. Chemical shift data was shown as follows.
Hexyl rhamnoside
1H NMR (400 MHz, CDCl3): δ 4.75 (s, 1H, H-1), 3.94–3.72 (m, 2H), 3.69–3.58 (m, 2H), 3.55–3.34 (m, 2H), 1.64–1.48 (m, 2H, OCH2CH2), 1.38–1.22 (m, 9H, CH3-6, (CH2)3), 0.89 (t, 3H, CH2CH3); 13C NMR (100 MHz, CDCl3): δ 99.66, 72.94, 71.88, 71.11, 67.93, 67.77, 31.58, 29.36, 25.74, 22.55, 17.50, 14.02.
Octyl rhamnoside
1H NMR (400 MHz, CDCl3): δ 4.74 (s, 1H, H-1), 3.95–3.72 (m, 2H), 3.69–3.57 (m, 2H), 3.54–3.34 (m, 2H), 1.64–1.48 (m, 2H, OCH2CH2), 1.38–1.20 (m, 13H, CH3-6, (CH2)5), 0.88 (t, 3H, CH2CH3); 13C NMR (100 MHz, CDCl3): δ 99.68, 72.88, 71.85, 71.11, 67.97, 67.79, 31.83, 29.41, 29.37, 29.23, 26.07, 22.63, 17.51, 14.07.
5-Nonyl rhamnoside
1H NMR (400 MHz, CDCl3): δ 4.84 (s, 1H, H-1), 3.90–3.70 (m, 3H), 3.63–3.55 (m, 1H, OCH(CH2)2), 3.48 (t, 1H), 1.55–1.41 (m, 4H, OCH(CH2)2), 1.38–1.20 (m, 11H, CH3-6, (CH2)2, (CH2)2), 0.90 (t, 6H, CH2CH3); 13C NMR (100 MHz, CDCl3): δ 98.54, 77.91, 73.02, 71.99, 71.67, 68.20, 34.14, 32.81, 27.57, 27.09, 22.88, 22.70, 17.38, 14.06, 13.99.
Decyl rhamnoside
1H NMR (400 MHz, CDCl3): δ 4.74 (s, 1H, H-1), 3.94–3.72 (m, 2H), 3.68–3.58 (m, 2H), 3.54–3.34 (m, 2H), 1.64–1.48 (m, 2H, OCH2CH2), 1.38–1.20 (m, 17H, CH3-6, (CH2)7), 0.88 (t, 3H, CH2CH3); 13C NMR (100 MHz, CDCl3): δ 99.66, 72.87, 71.85, 71.09, 67.95, 67.77, 31.88, 29.58, 29.43, 29.31, 26.08, 22.66, 17.51, 14.09.
Dodecyl rhamnoside
1H NMR (400 MHz, CDCl3): δ 4.74 (s, 1H, H-1), 3.94–3.72 (m, 2H), 3.68–3.58 (m, 2H), 3.52–3.34 (m, 2H), 1.63–1.48 (m, 2H, OCH2CH2), 1.38–1.20 (m, 21H, CH3-6, (CH2)9), 0.88 (t, 3H, CH2CH3); 13C NMR (100 MHz, CDCl3): δ 99.65, 72.98, 71.90, 71.13, 67.92, 67.81, 31.91, 29.66, 29.60, 29.44, 29.35, 26.10, 22.67, 17.51, 14.10.
Myristyl rhamnoside
1H NMR (400 MHz, CDCl3): δ 4.75 (s, 1H, H-1), 3.94–3.73 (m, 2H), 3.69–3.58 (m, 2H), 3.51–3.35 (m, 2H), 1.64–1.48 (m, 2H, OCH2CH2), 1.37–1.21 (m, 25H, CH3-6, (CH2)11), 0.88 (t, 3H, CH2CH3); 13C NMR (100 MHz, CDCl3): δ 99.63, 73.05, 71.90, 71.12, 67.88, 67.81, 31.92, 29.70, 29.61, 29.45, 29.36, 26.10, 22.68, 17.51, 14.11.
The synthesis of N-dodecyl rhamnose amine quaternary ammonium salt was divided into two steps. In the first step, white needle crystals (pure N-dodecyl rhamnose amine) were obtained after recrystallization in 80.2% yield. The second step is quaternization reaction. In order to accelerate the quaternization reaction, a large excess of methyl iodide was added into the mixture, n (rhamnose amine):n (methyl iodide) = 1:4. Due to the relatively low boiling point of methyl iodide, the excess of methyl iodide could be distilled off with the solvent after the reaction. The yield of the second step was 94.5%. So, the overall yield of N-dodecyl rhamnose amine quaternary ammonium salt, which was a sticky brown syrup-like substance, was 75.8%. The chemical formula of the 12N+-Rha was confirmed by the molecular ion peak in mass spectra (m/z 360.44 [M–I]+, as calculated m/z for C20H42N+O4 is 360.55[M–I]+) ().
HLB values and CMC of the alkyl rhamnosides
The HLB values of alkyl rhamnosides were calculated according to McGowan’s atomic volume method. For uncharged alkyl rhamnosides, the HLB values deceased from 7.794 to 3.994 with the increase in the fatty alcohol chain length as shown in . Besides, HLB of 12N+-Rha was 11.174 indicating strong hydrophilic capacity.
Table 2. Physicochemical parameters of the alkyl rhamnosides.
The CMC of uncharged alkyl rhamnosides and 12N+-Rha was measured by pyrene fluorescence probe method and conductivity method, respectively. The results are also shown in . There were apparently CMC for 8C-Rha, 4,4C-Rha, 10C-Rha and 12N+-Rha. Among them, the CMC of positive charged alkyl rhamnoside (12N+-Rha) was remarkably higher than uncharged alkyl rhamnosides. However, other uncharged alkyl rhamnosides did not show CMC due to the limitations of the assay method or poor water solubility.
Anti-bacterial activity of the alkyl rhamnosides
The antimicrobial activity of alkyl rhamnosides was investigated by their MICs against S. aureus (ATCC 29213) and P. aeruginosa (ATCC 27853). The MIC values are shown in . The alkyl rhamnosides presented various effective concentrations depending on their structures and the microorganism. For six uncharged alkyl rhamnosides, the MICs against S. aureus decreased remarkably with the increase in the length of carbon chain of fatty alcohol, and reached the minimum when the carbon number was 12 (12C-Rha). Only 8C-Rha and 4,4C-Rha had relatively high MIC values against P. aeruginosa while the other MICs exceed 104 nmol/mL. When positive charge was taken into consideration, the MIC value of 12N+-Rha against S. aureus was 39.1 nmol/mL, below 78.1 nmol/mL of uncharged 12C-Rha, and the MIC value of 12N+-Rha against P. aeruginosa was apparently far less than that of 8C-Rha and 4,4C-Rha. Besides, 8C-Rha showed lower MIC value than 4,4C-Rha, which means stronger antimicrobial effect of the alkyl rhamnosides with straight-carbon chain as compared to that with branched-carbon chain when the carbon number of fatty alcohol was same. In contrast, S. aureus was more sensitive to all alkyl rhamnosides, whereas P. aeruginosa was more resistant.
Table 3. Minimum inhibitory concentrations of alkyl rhamnosides.
Anti-biofilm activity of the uncharged alkyl rhamnosides
In general, the anti-biofilm activity of amphiphilic material was dependent on amphiphilic structure, material concentration and the microorganism tested. To test the anti-biofilm activity of the uncharged alkyl rhamnosides, biofilm formation inhibition assay and disruption of pre-formed biofilm assay were performed. The results of anti-biofilm formation inhibition are shown in . Uncharged alkyl rhamnosides showed clearly inhibition against S. aureus and P. aeruginosa within the concentration range examined indicating efficient anti-biofilm formation effect. Among them, 12C-Rha exhibited the strongest anti-adhesive activity against S. aureus (percentage inhibition 60–70%) even at the lowest concentration (39.1 nmol/mL) as shown in . And 8C-Rha showed the best anti-adhesive ability against P. aeruginosa (percentage inhibition 70–80%) at a concentration above 2500 nmol/mL (). Besides, the results of disruption of pre-formed biofilm are shown in , percentage inhibition for all alkyl rhamnosides increased with increasing material concentration, indicating that they disrupted pre-formed biofilms in a dose-dependent manner. Among them, 12C-Rha and 8C-Rha showed the best inhibition effect on S. aureus and P. aeruginosa biofilms dislodgement than any other at the same concentration, respectively, which was the same with the anti-biofilm formation results as shown in . Based on the above results as shown in and , there was an optimal carbon chain number for uncharged alkyl rhamnosides to show most potent anti-biofilm activity. Besides, the anti-biofilm ability of the alkyl rhamnoside with straight-carbon chain (8C-Rha) was stronger than that with branched-carbon chain (4,4C-Rha).
Figure 4. The anti-adhesive (biofilm formation inhibition) ability of the uncharged alkyl rhamnosides at different concentrations against S. aureus (A) and P. aeruginosa (B). Data represent the mean and SD of three independent experiments each in triplicate format.
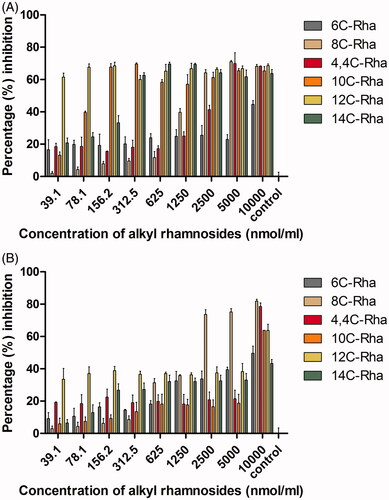
Figure 5. The effect of uncharged alkyl rhamnosides at different concentrations on one day developed biofilms disruption activity against S. aureus (A) and P. aeruginosa (B). Data represent the mean and SD of three independent experiments each in triplicate format. *p < .05; **p < .01; ***p < .001; ns, p > .05 versus control.
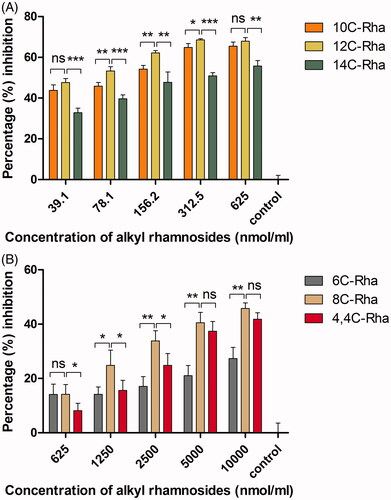
Anti-biofilm activity of N-dodecyl rhamnose amine quaternary ammonium salt
Effect of 12N+-Rha on S. aureus and P. aeruginosa biofilms at various concentrations is shown in . The percentage inhibition of anti-biofilm formation against S. aureus reached the highest point (66.77%) at a concentration of 39.1 nmol/mL, and declined slightly with further increase in concentration as shown in . Against P. aeruginosa, percentage inhibition was enhanced with the increase in the concentration from 19.5 to 625 nmol/mL, and reached maximum (66.64%) at a concentration of 625 nmol/mL. Interestingly, the MICs of 12N+-Rha against S. aureus and P. aeruginosa were 39.1 nmol/mL and 625 nmol/mL as shown in , respectively. It was demonstrated that 12N+-Rha also exhibited anti-adhesive ability under MIC and the effect reached the highest point at MIC. Besides, there was a maximum percent inhibition for 12N+-Rha against above two bacteria biofilms disruption within the concentration range examined as shown in . In detail, 12N+-Rha showed the highest inhibition effect of S. aureus biofilms dislodgement (55.98%) at a concentration of 312.5 nmol/mL (8 times as the MIC 39.1 nmol/mL), and P. aeruginosa biofilms dislodgement (61.16%) at a concentration of 1250 nmol/mL (two times as the MIC 625 nmol/mL), respectively. In contrast, 12N+-Rha inhibited more S. aureus biofilm formation at a lower concentration range (19.5–312.5 nmol/mL) than P. aeruginosa, whereas at high concentrations (1250–10,000 nmol/mL), the disruption of P. aeruginosa pre-formed biofilm was more pronounced than that of S. aureus pre-formed biofilm. At other concentration ranges, biofilm disruption did not differ significantly between above two bacteria.
Figure 6. The effect of concentration of 12N+-Rha on the anti-adhesive (biofilm formation inhibition) ability (A) and preformed biofilms disruption activity (B) against S. aureus and P. aeruginosa. Data represent the mean and SD of three independent experiments each in triplicate format. *p < .05; **p < .01; ***p < .001; ns, p > .05 versus control.
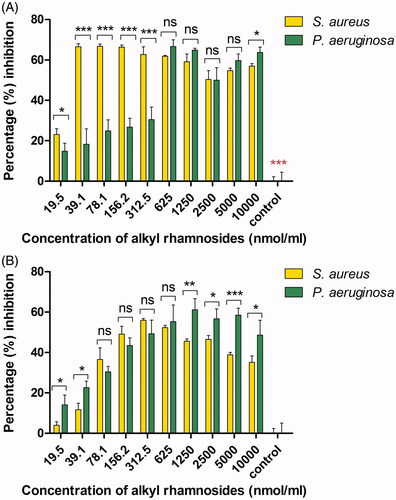
The comparison of anti-biofilm activity between N-dodecyl rhamnose amine quaternary ammonium salt and dodecyl rhamnoside
The most obvious distinction between 12N+-Rha and 12C-Rha is the presence of positive charge or not. Therefore, we chose 12C-Rha and 12N+-Rha to study the impact of positive charge for the anti-biofilm ability of alkyl rhamnosides. As shown in , the difference in the percentage inhibition against S. aureus biofilm formation between the two materials was unremarkable at a concentration of 312.5 nmol/mL or less, whereas above 1250 nmol/mL, the difference became significant, meaning that 12C-Rha had significantly stronger inhibition effect than 12N+-Rha (p < .05). Similarly, the superiority of 12C-Rha over 12N+-Rha was further clearly reflected in the disruption ability against S. aureus pre-formed biofilm (p < .01) in tested concentration range as shown in . The results demonstrated that nonionic alkyl rhamnosides have a more potent anti-biofilm effect against S. aureus than cationic alkyl rhamnosides. Another, against P. aeruginosa, the anti-adhesive ability of 12C-Rha was better than 12N+-Rha in low concentration range (39.1–312.5 nmol/mL) as shown in , while the result was opposite when the dose was higher than 625 nmol/mL, which indicated that the effect of 12N+-Rha was obvious in high concentration range. In the same way, the pre-formed biofilm disruption ability of 12N+-Rha against P. aeruginosa was significantly powerful than that of 12C-Rha (p < .01) in tested concentration range (). In conclusion, nonionic alkyl rhamnosides have a more potent anti-biofilm effect against S. aureus than cationic alkyl rhamnosides. On the contrary, the anti-biofilm activity of 12N+-Rha against P. aeruginosa was generally better than 12C-Rha. Therefore, uncharged alkyl rhamnoside was more suitable for Gram-positive bacteria, and Gram-negative bacteria were more sensitive to alkyl rhamnoside with positive charge.
Figure 7. The effect of 12C-Rha and 12N+-Rha on the anti-adhesive (biofilm formation inhibition) ability (A) and preformed biofilms disruption activity (B) against S. aureus. Data represent the mean and SD of three independent experiments each in triplicate format. *p < .05; **p < .01; ***p < .001; ns, p > .05 versus control.
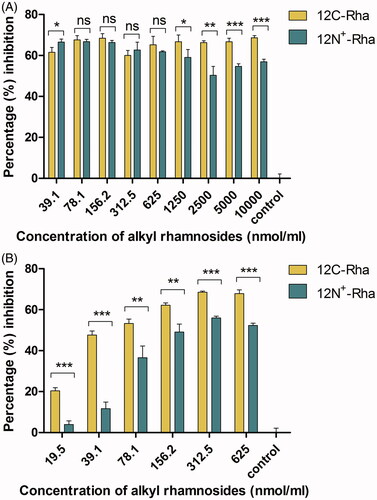
Figure 8. The effect of 12C-Rha and 12N+-Rha on the anti-adhesive (biofilm formation inhibition) ability (A) and preformed biofilms disruption activity (B) against P. aeruginosa. Data represent the mean and SD of three independent experiments each in triplicate format. *p < .05; **p < .01; ***p < .001; ns, p > .05 versus control.
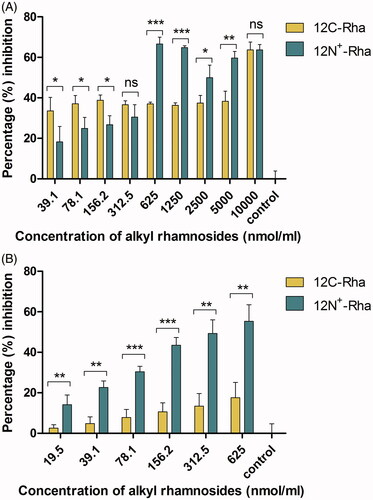
CLSM study on anti-biofilm activity of alkyl rhamnosides
Disruption of pre-formed biofilm promoted by conditioning the surface with alkyl rhamnosides was further confirmed by CLSM analysis. The untreated S. aureus cells grew as biofilms (), bacteria were closely connected to each other, forming a large number of micro-colonies. After treated by 12C-Rha (), S. aureus’ biofilms had been dispersed. The number of bacteria was significantly reduced and micro-colonies were disintegrated. In the control group, P. aeruginosa formed an intact biofilm with dense green and blue fluorescence (). However, the fluorescence coverage represented the area of biofilm surface coverage of P. aeruginosa treated by 12N+-Rha (1250 nmol/mL) was hardly detected (). The results of CLSM analysis were similar to those of quantitative crystal violet assays as mentioned above.
Proteomic analysis on biofilms response to 12C-Rha or 12N+-Rha
The list of differentially regulated proteins is shown in and . Comparing to control group, there were 308 proteins of S. aureus biofilm differentially regulated by exposure to 12C-Rha (136 up-regulated and 172 down-regulated). depicts the proteins involved in Kyoto Encyclopedia of Genes and Genomes (KEGG) pathways of S. aureus biofilm differentially regulated by 12C-Rha. In 12C-Rha treated group, most of the pathways were down-regulated including purine/pyrimidine metabolism, pentose phosphate pathway, ABC transporters, two-component system, ribosomal proteins, biosynthesis/metabolism of amino acids as well as fatty acid, folate biosynthesis, carbohydrate metabolism, mismatch repair and homologous recombination, decreased virulence. On the other hand, compared to control group, there were 487 proteins of P. aeruginosa biofilm differentially regulated by exposure to 12N+-Rha (283 up-regulated and 204 down-regulated). depicts the proteins involved in KEGG pathways of P. aeruginosa biofilm differentially regulated by 12N+-Rha. In 12N+-Rha treated group, most of the pathways were also down-regulated, such as purine/pyrimidine metabolism, pentose phosphate pathway, ABC transporters, two-component system, ribosomal proteins, fatty acid biosynthesis and metabolism that also occurred on S. aureus biofilms treated with 12C-Rha. The mechanisms above S. aureus and P. aeruginosa had in common suggested that alkyl rhamnosides had a broad-spectrum antibacterial biofilm activity. Besides, the other three down-regulated pathways including pyruvate metabolism, quorum sensing (QS) and oxidative phosphorylation were only exclusive to P. aeruginosa biofilms, which might be attributed to the introduction of charge for 12N+-Rha, thereby implementing anti-biofilm efficacy.
Table 4. The proteins involved in KEGG pathways of S. aureus biofilm differentially regulated by 12C-Rha.
Table 5. The proteins involved in KEGG pathways of P. aeruginosa biofilm differentially regulated by 12N+-Rha.
Discussion
The interest for application of novel amphiphilic materials in the medical field has been increased during the past decade due to their potential anti-microbial and anti-biofilm properties [Citation18]. The present study reported the potential of alkyl rhamnosides consisting of a rhamnose connected to a fatty alcohol with several carbon atoms. As a novel synthetic amphiphilic material, alkyl rhamnoside with precisely adjusted molecular structure is simply synthesized, which is superior to rhamnolipid. Moreover, the application of alkyl rhamnosides on biofilms and bacteria has not been reported yet.
It is well-known that the structure of amphiphilic materials has a direct impact on the activity of antimicrobial and anti-biofilm, however, the relationship between them has not been reported yet. The curve of the relationship between MIC and HLB of non-ionic alkyl rhamnoside against S. aureus is shown in . The MIC values of alkyl rhamnosides decreased by increasing HLB to 5.0. This trend was previously observed [Citation19]. The highest antibacterial activity was displayed for the alkyl rhamnoside (12C-Rha) with an HLB value of 5.0. The elongation of the hydrocarbon chain to 14 methylene group (HLB 4.0) caused increases in the MIC value. That is, MIC has a minimum value. The surface of S. aureus with peptidoglycan was lipophilic. The reduction of HLB for alkyl rhamnosides implied an increase in lipophilic capacity, which helped to bind to the bacteria surface and enter the bacteria inside to obtain anti-bacterial effect. However, a low HLB meant less solubility, so low concentrations might not meet the effective antibacterial. Coincidentally, the strongest anti-adhesive and biofilms dislodgement activity against S. aureus was 12C-Rha in non-ionic alkyl rhamnosides as shown in and . That is, the material with an HLB value of 5.0 also had the strongest antibacterial and anti-biofilm activity against S. aureus. The results indicated that HLB can be used to guide the selection of suitable non-ionic amphiphilic materials for antibacterial and anti-biofilm activities of gram-positive bacteria. It should be pointed out that whether HLB can be used to guide the antibacterial and anti-biofilm activities of ionic alkyl rhamnosides remained to be determined.
Figure 11. Schematic representation of KEGG pathways and functions responsible for bacterial biofilms formation and disruption treated by the alkyl rhamnosides.
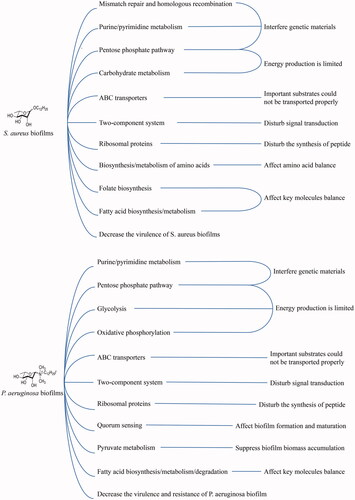
The charge of amphiphilic materials is also a factor that cannot be ignored. 12N+-Rha consisted of hydrophobic fatty chain and positive charge quaternized rhamnose, which was bound to the bacterial surface via electrostatic interaction between the positively charged hydrophilic face and the negatively charged head groups of lipopolysaccharide or biofilm extracellular polysaccharide. Then, the hydrophobic section of 12N+-Rha facilitated insertion into the membrane through hydrophobic interactions, causing increased permeability and loss of barrier function of the target gram-negative bacteria outer membrane and biofilms, eventually lysing the cell membrane or mediating biofilms detachment [Citation1]. In contrast, positively charged alkyl rhamnosides enhanced the ability of anti-negative and positive bacteria to achieve both broad-spectrum antibacterial and anti-bacterial biofilm effects, whereas non-ionic alkyl rhamnosides with the same carbon chain length were only positive for gram-positive effective bacteria. It demonstrated that the positively charged amphiphilic material was suitable for the biofilms produced by the mixed bacteria.
In vitro antibacterial and anti-biofilm activities are two very important parameters for evaluation of antimicrobial materials, however, the connection between them seemed to receive no attention. A comprehensive analysis of the results shown in and in our study showed that all alkyl rhamnosides at concentrations below their MIC produced certain inhibition effect of biofilm formation, and this effect was more pronounced with increasing concentration. Finally, this effect was maximized until near the MIC. Similarly, the biofilm inhibitory effect of cationic material (12N+-Rha) reached the maximum when the dose increased to or around its MIC, and then the further increase of the concentration did not further increase percentage inhibitory, indicating that MIC was the maximum biofilm inhibitory concentration for all alkyl rhamnosides. It was demonstrated that the stronger the antibacterial ability, the lower the dose required to obtain the maximum biofilm formation inhibitory effect. However, the preformed biofilm disruption effect continued to increase as the dose was increased above their MIC as shown in . Whether there was the relationship between the maximum preformed biofilm disruption effect and MIC needed further study. An increase of the hydrophobic alkyl chain length (from C6 to C12) for uncharged alkyl rhamnosides induced a decrease of the CMC from 3.36 to 0.238 μmol/mL and the MIC from over 104 nmol/mL to around 0.078 nmol/mL against S. aureus as shown in and . Noticeably, the antimicrobial ability against S. aureus was positively correlated with the surface activity of uncharged alkyl rhamnosides. These amphiphilic compounds with strong surface activity could interact with the bacterial cell bi-layer membrane, exerting a variety of effects [Citation20], thus leading to the bacterial lysis. Moreover, biofilm formation was inhibited below CMC as shown in , indicating that free alkyl rhamnosides could play this role. The formation of micelles consisted of alkyl rhamnosides seemed to be independent of anti-biofilm activity remarkably.
Amphiphilic materials-induced dispersal is not limited to some specific bacteria, they can lead to the dispersal of multi-species biofilms [Citation8]. Previous literature preferred to hold the opinion that amphiphilic materials disrupt biofilm cohesiveness in non-specific manners [Citation21]. For instance, due to their amphipathic nature, amphiphilic materials exhibited surface-acting properties that disrupted adhesiveness of cell–cell, cell–matrix and cell–surface interactions [Citation22], promoted bacterial motility contributing to the dispersal of biofilms, and acted directly on the biofilm matrix to degrade or repress production of adhesive components in the biofilm matrix [Citation23], perhaps even incorporating the matrix into micelles [Citation24]. Despite all the non-specific mechanisms above, none of them payed attention to the overall level of protein and pathway changes of biofilms, which was also valuable and instructive. Therefore, we led a comparative proteomics research to study on the differentially regulated proteins and pathways between the alkyl rhamnoside treated and untreated biofilms.
First of all, we compared the results of 12C-Rha treated biofilm proteomics with untreated ones. Stress-related protein DnaA that is abundant in mature biofilms [Citation25] was found downregulated after the treatment of 12C-Rha in our study. Glutamate dehydrogenase GudB, related to nitrogen metabolism and biofilm formation [Citation25], was suppressed in treated biofilms. Rpe acting as ribulose-phosphate 3-epimerase mapped to pentose phosphate pathway was also downregulated, so biofilm cells might not gain enough energy and 5-C sugars from this pathway for the synthesis of purines and pyrimidines [Citation25]. In accordance, four proteins (PurL, PurC, APRT and DPO1) mapped to purine metabolism pathway and four proteins (UMPS, Cdd, DPO1 and ComEB) mapped to pyrimidine metabolism pathway were all downregulated. The results strongly indicated that the suppression of purine/pyrimidine metabolism might be a mechanism of anti-biofilm function of 12C-Rha [Citation26]. In addition, two immunogens of S. aureus biofilm (CARP: leucyl aminopeptidase and FruK: 1-phosphofructokinase, Citation27] and one S. aureus infection related protein (Eap) were clearly downregulated after the treatment of 12C-Rha, which meant the virulence of S. aureus biofilms was also decreased.
The suppression of ABC transporters was another mechanism of anti-biofilm by 12C-Rha since nine proteins (OpuC, CycB, PstC, PhnC, ZnuA, MtsA, MtsB, HrtA and BraD) mapped to ABC transporters were downregulated. Thus, related substrates such as osmoprotectant, arabinogalactan oligomer/maltooligosaccharide, phosphate, phosphonate, iron/zinc/manganese/copper, hemin and bacitracin could not be transported properly in biofilm cells, causing the disruption of S. aureus biofilms [Citation28]. Moreover, the function of ZnuA and MtsA that is directly related with iron transportation should be emphasized because iron is important for biofilm development [Citation29]. Lack of iron might lead to the deficiency and disruption of biofilm [Citation30].
Six proteins (DnaA, KdpC, ResE, SaeR, Brad and BraR) mapped to two-component system were suppressed, most of them act as ATPase, kinase, response regulator or ATP-binding protein. As two-component system is important in nutrient acquisition, energy metabolism, virulence, adaptation to physical or chemical aspects of environment and complex developmental pathways of biofilm cells [Citation31–33], 12C-Rha played an important role in anti-biofilm by disturbing two-component system.
Two small subunits ribosomal proteins (RP-S14 and RP-S20) and one large subunit ribosomal protein (RP-L35) were found being downregulated after the treatment of 12C-Rha, indicating excellent ability to suppress ribosome, disturb the synthesis of peptide and then further influence the function and structure of biofilms [Citation34]. Besides, MOCS2 is molybdopterin synthase catalytic subunit protein and is important in folate biosynthesis. Its underexpression indicated that 12C-Rha inhibited the synthesis of folate that is essential for bacterial survival. It was noticed that FabG mapped to fatty acid biosynthesis/metabolism was downregulated in treated biofilm cells, meaning that 12C-Rha also suppressed the biosynthesis/metabolism of fatty acid, thus performing its anti-biofilm function [Citation35,Citation36].
It has been observed that 12C-Rha significantly disturbed biosynthesis/metabolism of amino acids, owing to the results that six proteins (Rpe, SerA, CysE, GLYCTK, MmuM and HutI) related to biosynthesis/metabolism of amino acids pathways were under-expressed in treated biofilm cells. Since more complex amino acids are needed in biofilm cells [Citation37], the disordered amino acid balance in S. aureus caused the un-proper formation and disruption of biofilm. As to carbohydrate metabolism related proteins, five proteins (MtlD, FruK, PTS-Gat-EIIA, Rpe and TarJ) were downregulated in 12C-Rha treated biofilm cells. That is, the energy production highly required in biofilm formation and maintenance was limited by 12C-Rha. In the contrary, we could also notice that several proteins (such as: HisA, TPI, PGAM and GpmI) related to biosynthesis/metabolism of amino acids pathways and several proteins (such as: GalM, RbsK and ManA) related to carbohydrate metabolism were over-expressed in treated biofilm cells, which might be explained by the normal stress response of S. aureus to 12C-Rha. In addition, seven proteins (MutS, UvrD, RecJ, Ssb, DPO3A1, RecG and RecD) mapped to mismatch repair and homologous recombination were changed differently (up or down), this might indicate that 12C-Rha also took some effects on S. aureus DNA.
As to analyse the effect of 12N+-Rha on P. aeruginosa biofilms, the contribution of positive charge should be taken into account. Two proteins of P. aeruginosa (FBP: fructose-1,6-bisphosphatase and G6PD: glucose-6-phosphate 1-dehydrogenase) mapped to pentose phosphate pathway were downregulated. So, similar to S. aureus, P. aeruginosa also gained too little energy and 5-C sugars from this pathway to synthesize purines and pyrimidines [Citation25]. In accordance, four proteins (PurM, PurC, RelA and Dgt) mapped to purine metabolism pathway and three proteins (CMPK1, Upp and TrxB) mapped to pyrimidine metabolism pathway were all suppressed. Thus, the suppression of purine/pyrimidine metabolism may be a mechanism of anti-biofilm function of 12N+-Rha. Since pyruvate metabolism has been proven to correlate with biofilm biomass accumulation and microcolony formation [Citation38], two downregulated important proteins in pyruvate metabolism (ME2 and ACSS) of treated biofilm cells indicated that 12N+-Rha inhibited P. aeruginosa biofilms by suppressing pyruvate metabolism.
Five proteins (PotF, PstS, AotP, LivK and MetQ) mapped to ABC transporters were downregulated. Thus, related substrates such as: putrescine, phosphate, arginine/ornithine, branched-chain amino acid and d-methionine could not be transported properly in biofilm cells, which might lead to deficiency of biofilm forming and disruption of preformed biofilm [Citation39]. Besides, 11 proteins (PstS, OmpR, ArnB, FliC, DctP, ME2, EvgS, Aer, CheV, CYTB and CcoP) mapped to two-component system were suppressed, most of them act as receptor, kinase, dehydrogenase, response regulator or binding protein. Similar to the effects of 12C-Rha on S. aureus biofilms, 12N+-Rha also disturbed ABC transporters and two-component system of the P. aeruginosa biofilms.
The underexpression of ribosomal proteins was also noticed. Three small subunit ribosomal proteins (RP-S14, RP-S7 and RP-S12) and two large subunit ribosomal proteins (RP-L16 and RP-L13) were found being downregulated after the treatment of 12N+-Rha, which suppressed ribosome, disturbed the synthesis of peptide and then further influenced the structure and function of biofilm [Citation40,Citation41], just like 12C-Rha did. Besides, five relevant proteins (PaaF, FabG, FabA, Fas and GCDH) were suppressed in treated biofilm cells, pointing same mechanism that 12N+-Rha performed its anti-biofilm function by disturbing fatty acid biosynthesis/metabolism/degradation [Citation42,Citation43] as 12C-Rha.
Interestingly, six downregulated QS related proteins (PlcC, LasB, TrpE, LivK, SecY and OprM) were found in 12N+-Rha treated biofilm cells. An impaired QS system may not regulate biofilm formation and/or maturation properly as it aimed to do [Citation29,Citation44,Citation45], thereby the suppression of QS was another important mechanism of anti-biofilm function of 12N+-Rha. Two proteins (FBP and ACSS) mapped to glycolysis were suppressed in treated biofilm cells, thus the energy production was limited in P. aeruginosa after the treatment of 12N+-Rha, which induced the dispersal of biofilms. Moreover, the underexpression of two oxidative phosphorylation related proteins (FbcH and CcoP) also confirmed the lack of energy production in treated biofilm cells [Citation46].
Different from 12C-Rha, 12N+-Rha specifically influenced the virulence and resistance of P. aeruginosa biofilms strongly. Vancomycin resistance relevant protein (MurF), lipopolysaccharide biosynthesis relevant protein (KdsA), four proteins mapped to cationic antimicrobial peptide (CAMP) resistance (LasB, AcrA, AcrB and ArnB) and four proteins mapped to beta-lactam resistance (AcrB, AcrA, OprM and TolC) were all downregulated after the treatment of 12N+-Rha, which reflected the impairment of virulence and the regain of antibiotic sensibility of P. aeruginosa biofilms due to 12N+-Rha [Citation47,Citation48]. What is more, the underexpression of GST (glutathione S-transferase) meant drug metabolism by cytochrome P450 was suppressed. These results provided a possible efficient combination therapy of 12N+-Rha together with antibiotic against P. aeruginosa biofilms infection.
Conclusions
In this study, a novel amphiphilic material, alkyl rhamnoside, was successfully synthesized for the first time. They gained amazing advantages such as simple synthesis process, excellent amphiphilic characteristics, and unexpected broad-spectrum antibacterial effect as well as broad-spectrum anti-biofilm activity, which has a wide range of applications. In addition, the relationships between the structural parameters of the alkyl-rhamnoside, as a representative of amphiphilic material, with the antibacterial activity and the anti-biofilm activity were established, which provided a useful reference for appropriate selection of amphiphilic materials as well as dose through HLB and MIC. More importantly, this study, for the first time, used proteomics to reveal the effect of amphipathic materials represented by alkyl rhamnosides on the bacterial proteome and suggested that alkyl rhamnosides interfered with the formation of bacterial biofilms or the dispersion of biofilms through multiple mechanisms that down-regulated the proteome as shown in . This may be a universal mechanism for amphipathic materials, hoping to help design and construct more novel amphiphilic materials for use in clinical and other fields.
Abbreviations | ||
6C-Rha | = | Hexyl rhamnoside |
8C-Rha | = | Octyl rhamnoside |
4,4C-Rha | = | 5-Nonyl rhamnoside |
10C-Rha | = | Decyl rhamnoside |
12C-Rha | = | Dodecyl rhamnoside |
14C-Rha | = | Myristyl rhamnoside |
12N+-Rha | = | N-Dodecyl rhamnose amine quaternary ammonium salt |
HLB | = | Hydrophilic–lipophilic balance |
MIC | = | Minimum inhibitory concentration |
CMC | = | Critical micelle concentration |
S. aureus | = | Staphylococcus aureus |
P. aeruginosa | = | Pseudomonas aeruginosa |
CFU | = | Colony-forming unit |
Rha | = | Rhamnose |
CLSM | = | Confocal laser scanning microscope |
MHB | = | Mueller-Hinton broth |
PBS | = | Phosphate-buffered saline |
Disclosure statement
The authors report no declarations of interest. The authors alone are responsible for the content and writing of the paper.
Additional information
Funding
References
- Davey ME, Caiazza NC, O'Toole GA. Rhamnolipid surfactant production affects biofilm architecture in Pseudomonas aeruginosa PAO1. J Bacteriol. 2003;185:1027–1036.
- Davey ME, O'Toole GA. Microbial biofilms: from ecology to molecular genetics. Microbiol Mol Biol Rev. 2000;64:847–867.
- Costerton JW, Stewart PS, Greenberg EP. Bacterial biofilms: a common cause of persistent infections. Science. 1999;284:1318.
- Teixeira PC, Leite GM, Domingues RJ, et al. Antimicrobial effects of a microemulsion and a nanoemulsion on enteric and other pathogens and biofilms. Int J Food Microbiol.. 2007;118:15–19.
- Otto M. Staphylococcal biofilms. Curr Top Microbiol Immunol. 2008;322:207–228.
- Brindle ER, Miller DA, Stewart PS. Hydrodynamic deformation and removal of Staphylococcus epidermidis biofilms treated with urea, chlorhexidine, iron chloride, or DispersinB. Biotechnol Bioeng. 2011;108:2968–2977.
- Zeraik AE, Nitschke M. Biosurfactants as agents to reduce adhesion of pathogenic bacteria to polystyrene surfaces: effect of temperature and hydrophobicity. Curr Microbiol. 2010;61:554–559.
- Boyle KE, Heilmann S, van Ditmarsch D, et al. Exploiting social evolution in biofilms. Curr Opin Microbiol. 2013;16:207–212.
- Jensen PO, Bjarnsholt T, Phipps R, et al. Rapid necrotic killing of polymorphonuclear leukocytes is caused by quorum-sensing-controlled production of rhamnolipid by Pseudomonas aeruginosa. Microbiology. 2007;153:1329–1338.
- Yu Y, Chen M. A protocol for the synthesis of C8-16alkyl 2,3-isopropylidene-α-l-rhamnosides. J Carbohydr Chem. 2014;33:489–497.
- Yan S, Ding N, Zhang W, et al. An efficient and recyclable catalyst for the cleavage of tert-butyldiphenylsilyl ethers. Carbohydr Res. 2012;354:6–20.
- Liu ZD, Liang PL, Chen XB, et al. Synthesis and characterization of sugar-based quaternary ammonium salt surfactants. Fine Chem. 2007;24:870–880.
- Sowada R, McGowan J. Calculation of HLB values. Tenside Surf Detergents. 1992;29:109–113.
- Luna JM, Rufino RD, Sarubbo LA, et al. Evaluation antimicrobial and antiadhesive properties of the biosurfactant Lunasan produced by Candida sphaerica UCP 0995. Curr Microbiol. 2011;84:1–1534.
- Zou Y, Lee Y, Huh J, et al. Synergistic effect of xylitol and ursolic acid combination on oral biofilms. Restor Dent Endod. 2014;39:288–295.
- Dalili D, Amini M, Faramarzi MA, et al. Isolation and structural characterization of Coryxin, a novel cyclic lipopeptide from Corynebacterium xerosis NS5 having emulsifying and anti-biofilm activity. Colloids Surf B Biointerfaces. 2015;135:425–432.
- Meng Y, Hou X, Lei J, et al. Multi-functional liposomes enhancing target and antibacterial immunity for antimicrobial and anti-biofilm against methicillin-resistant Staphylococcus aureus. Pharm Res. 2016;33:763–775.
- Dusane DH, Nancharaiah YV, Zinjarde SS, et al. Rhamnolipid mediated disruption of marine Bacillus pumilus biofilms. Colloids Surf B Biointerfaces. 2010;81:242–248.
- Brycki B, Kozirog A, Kowalczyk I, et al. Synthesis, structure, surface and antimicrobial properties of new oligomeric quaternary ammonium salts with aromatic spacers. Molecules. 2017;22:1810.
- Schreier S, Malheiros SV, de Paula E. Surface active drugs: self-association and interaction with membranes and surfactants. Physicochemical and biological aspects. Biochim Biophys Acta. 2000;1508:210–234.
- Lister JL, Horswill AR. Staphylococcus aureus biofilms: recent developments in biofilm dispersal. Front Cell Infect Microbiol. 2014;4:178.
- Kaplan JB. Biofilm dispersal: mechanisms, clinical implications, and potential therapeutic uses. J Dent Res. 2010;89:205–218.
- Ma Q, Yang Z, Pu M, et al. Engineering a novel c-di-GMP-binding protein for biofilm dispersal. Environ Microbiol. 2011;13:631–642.
- Gakhar L, Bartlett JA, Penterman J, Jr. et al. PLUNC is a novel airway surfactant protein with anti-biofilm activity. PLoS One. 2010;5:e9098.
- Resch A, Leicht S, Saric M, et al. Comparative proteome analysis of Staphylococcus aureus biofilm and planktonic cells and correlation with transcriptome profiling. Proteomics. 2006;6:1867–1877.
- Rebecca Y, Peng C, Shi W, et al. Genetic screen reveals the role of purine metabolism in Staphylococcus aureus persistence to rifampicin. Antibiotics. 2015;4:627–642.
- Brady RA, Leid JG, Camper AK, et al. Identification of Staphylococcus aureus proteins recognized by the antibody-mediated immune response to a biofilm infection. Infect Immun. 2006;74:3415–3426.
- Li L, Cheung A, Bayer AS, et al. The global regulon sarA regulates beta-lactam antibiotic resistance in methicillin-resistant Staphylococcus aureus (MRSA) in vitro and in endovascular infections. J Infect Dis. 2016;214:1421–1429.
- Khemiri A, Jouenne T, Cosette P. Proteomics dedicated to biofilmology: What have we learned from a decade of research? Med Microbiol Immunol. 2016;205:1–19.
- Arciola CR, Campoccia D, Speziale P, et al. Biofilm formation in Staphylococcus implant infections. A review of molecular mechanisms and implications for biofilm-resistant materials. Biomaterials. 2012;33:5967–5982.
- Brooks JL, Jefferson KK. Staphylococcal biofilms: quest for the magic bullet. Adv Appl Microbiol. 2012;81:63–87.
- Kolar SL, Nagarajan V, Oszmiana A, et al. NsaRS is a cell-envelope-stress-sensing two-component system of Staphylococcus aureus. Microbiology. 2011;157:2206–2219.
- Waters NR, Samuels DJ, Behera RK, et al. A spectrum of CodY activities drives metabolic reorganization and virulence gene expression in Staphylococcus aureus. Mol Microbiol. 2016;101:495–514.
- Metselaar KI, den Besten HM, Boekhorst J, et al. Diversity of acid stress resistant variants of Listeria monocytogenes and the potential role of ribosomal protein S21 encoded by rpsU. Front Microbiol. 2015;6:422.
- Korem M, Gov Y, Rosenberg M. Global gene expression in Staphylococcus aureus following exposure to alcohol. Microb Pathog. 2010;48:74–84.
- Mirani ZA, Jamil N. Role of extra-cellular fatty acids in vancomycin induced biofilm formation by vancomycin resistant Staphylococcus aureus. Pak J Pharm Sci. 2013;26:383–389.
- Prax M, Bertram R. Metabolic aspects of bacterial persisters. Front Cell Infect Microbiol. 2014;4:148–148.
- Petrova OE, Schurr JR, Schurr MJ, et al. Microcolony formation by the opportunistic pathogen Pseudomonas aeruginosa requires pyruvate and pyruvate fermentation. Mol Microbiol. 2012;86:819–835.
- Park AJ, Murphy K, Krieger JR, et al. A temporal examination of the planktonic and biofilm proteome of whole cell Pseudomonas aeruginosa PAO1 using quantitative mass spectrometry. Mol Cell Proteomics. 2014;13:1095–1105.
- Imperi F, Leoni L, Visca P. Antivirulence activity of azithromycin in Pseudomonas aeruginosa. Front Microbiol. 2014;5:178.
- Irie Y, MS, Edwards AN, Wozniak DJ, et al. Pseudomonas aeruginosa biofilm matrix polysaccharide Psl is regulated transcriptionally by RpoS and post-transcriptionally by RsmA. Mol Microbiol. 2010;78:172.
- Deschamps JD, Ogunsola AF, Jameson JB, et al. Biochemical/cellular characterization and inhibitor discovery of Pseudomonas aeruginosa 15-lipoxygenase. Biochemistry. 2016;55:3329–3340.
- Kannan A, Karumanchi SL, Ramalingam S, et al. Quantitative study on the effect of calcium and magnesium palmitate on the formation of Pseudomonas aeruginosa biofilm. J Microbiol Immunol Infect. 2016;49:988–991.
- Sakuragi Y, Kolter R. Quorum-sensing regulation of the biofilm matrix genes (pel) of Pseudomonas aeruginosa. J Bacteriol. 2007;189:5383–5386.
- Seyer D, Cosette P, Siroy A, et al. Proteomic comparison of outer membrane protein patterns of sessile and planktonic Pseudomonas aeruginosa cells. Biofilms. 2005;2:27–36.
- Saville RM, Rakshe S, Haagensen JAJ, et al. Energy-dependent stability of Shewanella oneidensis MR-1 biofilms. J Bacteriol. 2011;193:3257–3264.
- Guo QYW, Xia B, Jin Y, et al. Identification of a small molecule that simultaneously suppresses virulence and antibiotic resistance of Pseudomonas aeruginosa. Sci Rep. 2016;6:1305–1307.
- Shin S, Ahmed I, Hwang J, et al. A microfluidic approach to investigating a synergistic effect of tobramycin and sodium dodecyl sulfate on Pseudomonas aeruginosa biofilms. Anal Sci. 2016;32:67–73.