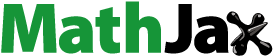
Abstract
Utilizing novel approaches for the green synthesis of metal nanoparticles are of great importance. Therefore, we reported biogenic synthesis of silver nanoparticles (AgNPs) using extracts of Leptolyngbya strain JSC-1, and their significant applications against pathogenic bacteria and cancerous HeLa cell line. The biofabricated AgNPs were characterized by UV–visible spectroscopy, FTIR, SEM, TEM, DLS and zeta-potential. The as prepared AgNPs were assessed for inhibition of bacterial growth and induction of apoptosis in HeLa cells by different doses of AgNPs was evaluated. UV–visible spectroscopy and FTIR of AgNPs demonstrated the surface plasmon resonance at 413 nm and interaction among extract and nanoparticles, respectively. Electron microscopy revealed the morphology and DLS demonstrated size distribution of the particles (10–100 nm). Zeta potential values were between −47 and 0 mV, indicating stability of the particles. Proliferation of HeLa cells was significantly inhibited and severe cytotoxicity with higher intracellular uptake were observed after applying high concentration of AgNPs. Efficient inhibition zones (17 ± 2 and 21 ± 2 mm) were produced at maximum concentration (100 µl from 1 mg ml−1 stock of AgNPs) for Staphylococcus aureus and Escherichia coli, respectively. These findings reveal that the biofabricated AgNPs possess strong antibacterial activity and ability to induce apoptosis in cancer cell line (HeLa).
Introduction
Recently, numerous important technological innovations occurred in the field of nanotechnology. Nanoscience and nanotechnology deal with the synthesis of nanoparticles (NPs) of different chemical compositions [Citation1]. At present, the importance of this field emerges in environmental science because of wide applications of nanoparticle for removal of toxic chemicals and pollutants from environment [Citation2]. It is a nanorevolution persisted to unfold the manufacturing of environment friendly and non-polluting nanomaterials. Chemical synthesis of such materials might lead to addition of some deadly chemicals on their surfaces that may have unfavourable effects in medical applications [Citation3]. Therefore, the researchers are engaged to think about alternatives and find other ways; hence, green synthesis and assembly of nanoparticles might be a best choice [Citation4]. The use of eco-friendly materials like whole plant, parts of plant, algae and cyanobacteria for the synthesis of nanoparticles (NPs) offer several benefits for environment and compatibility for biomedical applications [Citation5]. Free metal nanoparticles due to their unique physical and chemical properties have potential applications in catalysis, bio-sensing, bio-labelling, electronics, optical devices and controlled drug delivery [Citation6,Citation7].
Bioactive materials in the extracts of photosynthetic cyanobacteria can act as reducing and stabilizing agents and confer more biocompatibility to biosynthesized NPs [Citation8]. Algae including cyanobacteria are a group of naturally available bio-factories for bioactive products [Citation9]. These bioactive compounds might be proteins, carbohydrates, lipids, vitamins, carotenoids and many other secondary metabolites with a wide range of biological activities and applications [Citation10]. These bio-nanofactories possess unique ability to synthesize biochemicals, involved in the synthesis of metallic nanoparticles with high stability [Citation11]. Recently, metal nanoparticles were synthesized using the extracts of algae such as Turbinaria conoides, Kappaphycus alvarezii, Laminaria japonica, Chlorella pyrenoidusa, Stoechospermum marginatum, Sargassum wightii, Acanthophora spicifera and Sargassum myriocystum [Citation7]. One of the largest group of algae namely cyanobacteria (blue-green algae) found in natural aquatic environment were also reported for the same purpose of bio-fabrication of metal nanoparticles [Citation12].
Because of wide applications of AgNPs in health care, they might be exploited for cancer treatment, which is one of the most deadly diseases in the world. Currently, for cancer treatment different ways are under practice, including surgical intervention, chemotherapy and radiation, which might also destroy healthy cells, thus causing other complications and are harmful for the patients [Citation13]. Hence, there is a dire need to find new ways of treatment that might have fewer or no side effects and safer for cancer patients. Nanoparticles are widely investigated for biological applications and medical purposes due to their unique optical properties, eco-friendly nature and electrochemical stability. Therefore, in this study, AgNPs were synthesized using cyanobacterial extracts and then their apoptotic effects against HeLa cells and some pathogenic bacteria were investigated.
Materials and methods
Reagents and instruments
The experimental materials including silver salts (analytical grade) were procured from Sigma Aldrich and the solvent used throughout the experiments was double distilled water (Millipore 18.2 MΩ cm). All other instruments used in the study were High speed refrigerated centrifuge (JW-3021HR), UV/Vis spectrophotometer (Shimadzu, 2450), X-ray diffraction (Powder X-ray-D8 advanced diffractometer, BRUKER), scanning electron microscope (JEOL JEM-3100), EDX (JEOL-JEM 3010), transmission electron microscope (FEI-Tecnai G2 20 TEM), dynamic light scattering and zeta potential (HORIBA Zetasizer SZ100), FTIR (Nicolet 6700 FT-IR spectrometer), MTT assay (MAXline microplate reader), ICP-AES (Skyray instruments ICP2060T) and confocal microscope (Leica TCS SP8).
Cyanobacterial cultivation
Leptolyngbya JSC-1 (a siderophilic cyanobacterium) was gifted by Igor I. Brown (Beijing University of Chemical Technology, Beijing) [Citation14]. It was grown in modified DH medium (pH 8) using 4.6 mM 4-(2-hydroxyethyl)-1-piperazine ethane sulfonic acid (HEPES) as a buffer. The medium was supplemented with 40 µM iron for its optimum growth. The growth was carried out for 10 days in 500 ml flasks (45 ± 1 °C) under 24 h light condition in a growth chamber [Citation14].
Preparation of extracts
Biomass of Leptolyngbya JSC-1 was acquired by centrifugation of the culture at 5000g for 12 min. The growth medium (supernatant) was discarded and the collected pellet (biomass) was thoroughly washed with distilled water. Finally, the pellet (biomass) was dried at 60 °C and crushed into powder using an agate mortar. One gram of the powdered biomass was dispersed in 100 ml of distilled water and maintained at 60 °C for 30 min on mild stirring. The extracted biochemicals from JSC-1 biomass were separated by centrifugation at 6000 rpm (10 min) [Citation13].
Synthesis of silver nanoparticles
For the preparation of AgNPs, 10 ml of the JSC-1 extract was treated with 50 ml of 1 mM silver nitrate (AgNO3) solution and kept on stirring (500 rpm) at room temperature [Citation15]. The development of blackish colour in the reaction mixture designates the synthesis of AgNPs. Optical property such as localized surface plasmon resonance (LSPR) for the AgNPs was identified by UV–visible spectrometry (Shimadzu, 2450). The reaction was terminated after SPR bands saturation. The acquired product in suspension was recovered by centrifugation at 12,000 rpm for 15 min. The collected AgNPs were repeatedly washed and freeze-dried [Citation13].
Characterization of nanoparticles
UV–visible spectroscopy
The SPR pattern of AgNPs was investigated by UV–vis spectroscopy. The materials were scanned in the range of 200–800 nm [Citation13,Citation15].
Transmission electron microscopy (TEM)
Particles sizes and shapes of the AgNPs were visualized by transmission electron microscopy. The homogeneous suspension of biogenic particles of silver were mounted on the carbon-coated copper grid, dried in air and then analysed with FEI-Tecnai G2 20 TEM [Citation15,Citation16].
Scanning electron microscopy and energy-dispersive X-ray (SEM and EDX)
Surface features and elemental analysis of AgNPs maintained on graphite grid were studied by SEM and EDX techniques (JEM-3100 equipped with an EDX unit) [Citation13,Citation15,Citation16].
X-ray diffraction (XRD)
The X-ray diffraction (XRD) profile of the synthesized materials was obtained with X-ray diffractometer (Powder X-ray-D8 advanced diffractometer, Burker) at 2θ, 10–100 using Cu Kα radiation at 40 kV and 30 mA [Citation13,Citation15,Citation16].
Fourier transformed infrared (FTIR)
FTIR spectral bands in the prepared materials (AgNPs) were determined using a Nicolet 6700 FT-IR spectrometer, @ 500–4000 cm−1 in transmittance mode. Samples for FTIR analysis were prepared using the KBr pellet technique, which involves mixing thoroughly the as prepared AgNPs with KBr before forming a pellet at high pressure. IR bands of Leptolyngbya JSC-1 powder extracts and biosynthesized AgNPs were compared to evaluate the probable functional groups involved in AgNPs formation [Citation13,Citation15,Citation16].
DLS and zeta potential
The distribution and size of AgNPs were measured using dynamic light scattering (DLS) and zeta potential (HORIBA Zetasizer SZ-100) [Citation17].
Biological activity of AgNPs
Growth inhibition study of bacteria
Both strains of bacteria (Staphylococcus aureus and Escherichia coli) were cultured in LB broth, which were further inoculated in fresh 10 ml liquid medium. Both the strains were then treated with different concentrations of biosynthesized AgNPs (0, 25, 50 and 100 µg/ml) under optimum conditions of bacterial growth (37 °C and 150 rpm continuous shaking). Growth of both the strains in LB liquid medium was measured by optical density (OD) @ λ = 600 nm at regular intervals (1, 2, 4, 6, 8, 10, 12, 14, 16, 18, 20, 22 and 24 h) using UV–Vis spectrophotometer. The growth curve was obtained by plotting optical density against time [Citation18].
Well diffusion method
Well diffusion method was used to assess the antibacterial potential of the biosynthesized AgNPs against two pathogenic strains (S. aureus and E. coli). Four Petri-dishes were prepared with Luria Bertani (LB) agar medium having wells of 8 mm diameter at equal distance. After inoculation, the wells were loaded with different concentrations (0, 25, 50 and 100 µl) from stock solution of AgNPs (1 mg ml−1). The plates were incubated at 37 °C and after 24 h, the inhibition zones were measured [Citation19].
Cellular damage
Cellular morphologies of S. aureus and E. coli treated with varying concentrations of AgNPs (0, 25, 50 and 100 µl) were examined after 24 h of incubation using the standard method for scanning electron microscopy [Citation16].
Anticancer activity of AgNPs
Anticancer activity of the AgNPs was evaluated by a dose-dependent manner using HeLa cells of human epithelioid cervix carcinoma cells. The cells were exposed to 5, 10, 20, 40, 80 and 160 μg ml−1 of AgNPs, in culture media for 24 h and the viability of cells was determined by MTT assay. The cells were washed thrice with DMSO and added to each well having MTT solution (10%) and incubated for 5 h. The absorbance was measured @ 570 nm and the percent cell survival was determined as the percent MTT reduction by cells exposed to different concentrations of AgNPs relative to control group [Citation20].
AO/EB fluorescent staining for analysis of apoptosis
Cells of human epithelioid cervix carcinoma (HeLa) in the logarithmic growth phase were cultured in 96-well plate containing Dulbecco's modified Eagle's medium (DMEM) (100 μl/well). Cells were added to a final concentration of 5 × 103 ml−1 and were incubated. HeLa cells were left untreated as control, treated with only extracts, and different concentrations of AgNPs, i.e. 5, 10, 20, 40, 80 and 160 µg ml−1. The samples in 96-well plate were divided into eight groups, with 12 samples in each group corresponding to different treatments. After being cultured for 24 h, the cell culture suspensions (20 μl each) were mounted on glass slides. Dual fluorescent staining solution (1 μl) containing acridine orange/ethidium bromide (AO/EB, 100 μg each ml−1) was added. The morphology of apoptotic cells was examined using a fluorescent microscope [Citation21].
In vitro cellular uptake of AgNPs
In vitro cellular uptake of biogenic AgNPs was determined after the HeLa cells previously grown in Dulbecco's modified Eagle's medium (DMEM) in the presence of different concentrations of AgNPs, at 37 °C for 24 h and adjusted the pH at 7. These cells were treated with trypsin and collected by centrifugation (1600 rpm, for 5 min) and washed the cells with PBS thrice during centrifugation to remove extracellular AgNPs. Then resuspend the cells pellet in PBS (2 ml) and according to Privies protocol the samples were prepared for ICP-AES analysis [Citation20].
Results and discussion
Synthesis of AgNPs and UV–visible spectroscopy
After mixing the Leptolyngbya JSC-1 extracts with the AgNO3 solution, the colour change occurred from transparent to dark blackish. The colour change of the mixture was noted visually (). After 20 min of the reaction, both changes in colour and the absorbance were recorded at regular intervals, as the colour change was the first evidence of nanoparticles formation. Generally, the colour change occurred due to reducing agents released from algal extracts into solution [Citation21]. This was also supported by UV–visible spectra, when exposed to light of specific wavelength, the NPs gave a unique surface phenomenon called surface plasmon resonance (SPR); due to this, a specific peak formation occurred for each kind of NPs by UV–Vis spectroscopy [Citation7]. The synthesis of AgNPs using (Leptolyngbya JSC-1) extract was monitored by using UV–Vis spectroscopy; the light absorption pattern of the algal biomass was observed in the range of 300–700 nm. The silver ions reduction peak of the SPR occurred at 413 nm (). The same peak area for AgNPs at 413 nm was also reported by other researchers, one of them was incubation of Ag+ with fungal biomass of Trichoderma koningii [Citation19].
Figure 1. Confirmation of AgNPs synthesis by visual colour change (A). UV–Vis spectra of the biofabricated AgNPs at different time intervals (B).
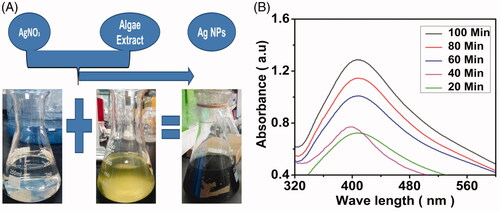
Algal extracts are rich in bioactive molecules and functional groups like –OH, carboxylic and amide groups which improved the quantitative production of AgNPs and their stable dispersion. Therefore, algal extracts have the power to be used for the synthesis of nanoparticles. It is well known that the extinction coefficient of AgNPs at their SPR band peak is proportional to the nanoparticle concentration. Thus, the method publicized powerful potential in producing high-concentration of AgNPs with controlled range of particles size which is the priority for large-scale fabrication and development of value-added nano products [Citation22].
TEM analysis of AgNPs
The TEM images confirmed that the newly biofabricated AgNPs are well dispersed consisting mostly of spherical and nearly spherical shapes (). These micrographs showed a spherical conformation of majority of the AgNPs with a size range of 5–50 nm (). Selected area electron diffraction (SAED) of a single spherical particle was explained and the size measured by TEM analysis was found to be lower than that observed in DLS analysis. Similar results were also found by other researchers recently [Citation23–25].
Scanning electron microscopy (SEM) and energy-dispersive X-ray (EDX) analysis
SEM analysis revealed the morphology and size of the biosynthesized AgNPs (). It is also evident that the biomolecules in the extracts of JSC-1 promoted synthesis of AgNPs. The SEM images of AgNPs reported by other researchers, from different extracts showed spherical particles, aggregated spherical particles, irregularly shaped particles and cubic particles. The moderate particles size observed were 12, 25, 35 and 50 nm, for AgNPs synthesized by JSC-1 extracts using water as a solvent [Citation26–29].
EDX of the NPs was performed to investigate the elemental composition and stoichiometry of the biosynthesized AgNPs (). The EDX spectra revealed the presence of silver peaks around 3 and 3.1 keV, which were appeared due to the discharge of different electrons from L and K shells of silver, respectively. The lower energy peak (3 keV) was responsible for the outer shell electrons (L) and higher energy peak (3.1 keV) was responsible for inner shell electrons (K). The carbon and platinum peaks present in the spectra were mainly due to the carbon adhesive tape and platinum coating used and other peaks were due to inorganic impurities from the biomolecules in the Leptolyngbya JSC-1 extract. The EDX data of AgNPs showed that the weight percentage of Ag was 53.77% [Citation26,Citation27].
XRD and FTIR analysis
The biosynthesized silver nanostructures were further analysed by XRD that determined the crystalline structure of the bio-synthesized AgNPs (). The XRD pattern of the AgNPs from 20 to 90 2θ using X-ray diffractometer confirmed that there were five well-defined characteristic diffraction peaks at 34.5°, 35.1°, 47.1°, 58.4° and 86.7°, these peaks corresponds to the 111, 200, 220, 311 and 422 diffractions, respectively. The XRD features of AgNPs are matched with standard silver which was reported by JCPDS {file no. 04–0784}. Also widening of the diffraction peaks was observed which might be the effect of the size of nanoparticles. As the nanoparticles are capped by the moieties of JSC-1 biomolecules, therefore, the background observed was high [Citation26–28].
Figure 3. XRD spectrum of AgNPs displaying characteristic diffraction peaks (A) and FTIR spectrum of the biosynthesized AgNPs shows different functional groups involved in capping (B).
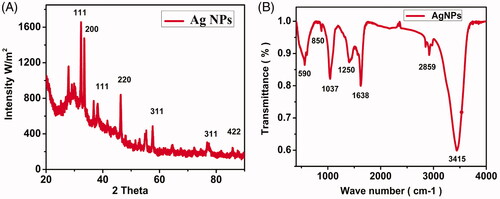
FTIR was employed to quantify and determine the functional biomolecules in the JSC-1 extracts which were important for the reduction of silver ions into relative metal nanoparticles. The JSC-1 extracts have variety of biomolecules, which might possibly be involved in the synthesis of these NPs (). The occurrence of many outstanding signatory peaks in the IR region of electromagnetic spectrum is due to different functional groups. The active and extended band was observed at 3415 cm−1 that confirmed the presence of polyphenolic –OH group. The second band was observed relative to alkanes group of C–H stretching vibrations at the absorption band 2859 cm−1, the third narrow band occurred at 1638 cm−1 indicating the presence of amide I group, the fourth short band appeared at 1250 cm−1 assigned to C–C stretching of aromatic ring, the fifth narrow band at 1037 cm−1 corresponds to C–N stretching vibrations of aliphatic amines of protein [Citation28]. The sixth very short band at 850 cm−1 attached to S–O stretching of sulfonates, while the last band at 590 cm−1 represented alkyl halides. This investigation also demonstrated that protein and amino acids have the capacity to bind metal. It is evident that polysaccharides, sulfonated compounds and amide linkages are strongly involved in the reduction of silver ions into nanoparticles. Earlier reports are also in line with this study confirming the presence of carboxylic, amine, phosphate and hydroxyl functional groups in the algal extract of Sargassum polycystum and are involved in the reduction of silver ions. The biomolecules present in algal extract have dual functions of reducing silver ions and stabilizing the AgNPs [Citation23,Citation30].
Dynamic light scattering (DLS) distribution and zeta potential
Dynamic light scattering (DLS) analysis is very important and useful approach to analyse particle size and its distribution in the nanomaterials. DLS of the biosynthesized AgNPs displayed a particle size range of 10–138 nm (). The average expected size of AgNPs was identified to be 71.5 nm. The variation in particles size as determined by two techniques (DLS and TEM) may be ascribed to the fact that these techniques are based on different detection methods and principles. Besides, DLS measures the hydrodynamic diameter of the particles which might be augmented by water molecules, thus, the resulting particles size is generally broader than that obtained from TEM analysis [Citation31–33].
Figure 4. Dynamic light scattering (A) and Zeta potential (B) of the biosynthesized silver nanoparticles, illustrating the particle size distribution and surface charge value, respectively.
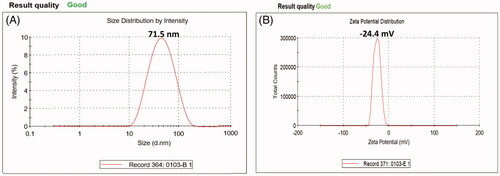
Zeta potential analysis of the biosynthesized AgNPs was found as a single sharp peak between −47 and 0 mV while having a maximum intensity at −24.4 mV (). It indicates that the negatively charged moieties are present on the surface of the AgNPs that expanded in the medium. The repulsion among the particles might be due to the negative values that proved that the particles are very stable. Low zeta potential values of particles suggest no flocculation and no tendency to assemble together due to repulsion forces among particles [Citation33,Citation34].
Inhibition of bacterial growth and antibacterial activity
Antibacterial activities of the biosynthesized AgNPs were performed for E. coli and S. aureus and the growth rates were measured by optical densities at regular intervals after treating with different doses of AgNPs (0–100 µl from stock solution 1 mg ml−1) (). It was found that the growth of both bacteria was decreased with increasing the concentration of AgNPs and vice versa. The minimum inhibitory concentration (MIC) of AgNPs was 25 µl while the growth rates of both strains of bacteria were quite low at 50 and 100 µl.
Figure 5. Growth variation of E. coli and S. aureus (A and B, respectively) at different concentrations (0, 25, 50 and 100 µl) of AgNPs after incubation for 24 h. Error bars indicate the triplicates in experiment where p values was ≤.05. Inhibition zones in culture plates at varying concentrations of AgNPs like 0, 25, 50 and 100 µl indicated as a, b, c and d for E. coli while A, B, C and D for S. aureus, respectively.
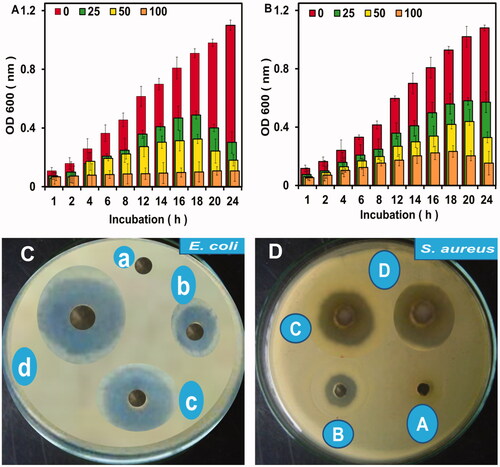
The antibacterial potency of the biosynthesized AgNPs was inspected against the pathogenic bacteria (E. coli and S. aureus). The diameter of inhibition zones were measured as a score, indicating that the diameter of inhibition zones increases with increasing the concentration of AgNPs. No inhibition zone was noted in the control (having no AgNPs). The average diameter of inhibition zones were 9 ± 2 mm, 15 ± 2 mm and 21 ± 2 mm at 25, 50 and 100 µl, respectively for E. coli (). Inhibition zones for S. aureus were measured as 7 ± 2 mm, 12 ± 2 mm and 17 ± 2 mm at 25, 50 and 100 µl concentrations of AgNPs, respectively (). The maximum inhibition zone was observed at 100 µl concentration of AgNPs for E. coli (21 ± 2 mm), suggested the efficacy of the biosynthesized NPs against the pathogenic bacterial strains. Comparatively higher activity against E. coli may be due to the structural variation and cell wall composition in both species. The thin peptidoglycan layer in E. coli would produce less barriers for particles to enter the cells. The probable mechanism of action of the noble metal nanoparticles to eradicate bacteria might be the membrane damage, creation of reactive oxygen species (ROS), inhibition of some essential enzymes and DNA damage, etc. [Citation7]. Among these mechanisms, the most effective was found to be the ROS production and cell membrane disruption leading to leakage of nucleic acids and protein from bacterial cells [Citation16,Citation19].
Cell membrane disruption
SEM micrographs displayed a sever membrane disruption of bacterial cells exposed to four different concentrations of biogenic AgNPs (0, 25, 50 and 100 µl from stock solution 1 mg ml−1). The extracellular morphology of treated cells indicated pores formation in both strains at 25 µl AgNPs (). Subsequent leakage of the cytoplasmic materials and cell membrane disruption was observed at 50 and 100 µl of AgNPs (), respectively. In comparison to control group (), the cellular deformation was found to be more severe in case of E. coli than S. aureus [Citation34,Citation35].
Anticancer activity using HeLa cell line
The anticancer efficiency of biofabricated AgNPs were evaluated using HeLa cell line as the target and both percent viability () and cancer cell mortality rate () were examined. HeLa cells treated with several concentrations of biogenic AgNPs (0, 5, 10, 20, 40, 80 and 160 µl from 1 mg ml−1 stock) for a period of 24 h, and then the morphology of these cells were studied by using MTT assay (). Significant difference in terms of decline in number of viable HeLa cells were observed after treating them with biogenic AgNPs as compared to extracts only, where p value was ≤.05 as calculated using student’s t test.
Figure 7. Percent viability of cancer cells after treating with AgNPs (0, 5, 10, 20, 40, 80 and 160 µl) for 24 h (A), cancer cells mortality rate (B) and cytotoxicity to HeLa cells studied by MTT assay (C). Errors bars indicate that the experiments were carried out in triplicates and where p value was ≤.05 as calculated using student’s t test. The further division of (C) such as i, ii, iii, iv, v, vi, vii and viii correspond to different treatments, e.g. extracts only, 0, 5, 10, 20, 40, 80 and 160 µl, respectively.
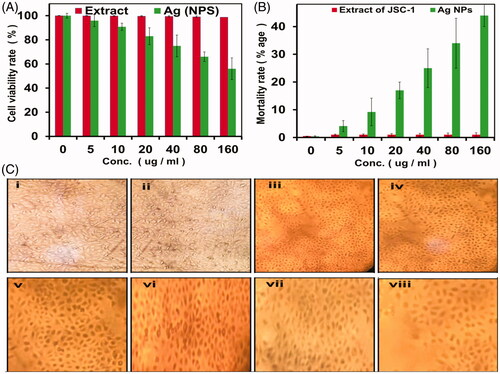
Cellular viability and mortality were determined after applying AgNPs by MTT assay and it was observed that the number of viable cells was decreased with increasing concentration of biogenic AgNPs. Finally, 55% viability was detected at the highest concentration (160 µl). The “extract only” did not show any significant cytotoxicity towards HeLa cells [Citation36,Citation37]. This indicated that the AgNPs become more potent after reduction of silver ions by the extracts utilized, showing high mortality rate (45%) when applied to HeLa cells (). Our findings are in line with the previous studies, suggesting potential use of metallic NPs against cancer cells [Citation38–40].
Analysis of apoptosis
The apoptotic morphological changes in HeLa cells caused by AgNPs were studied by using dual staining AO/EB. It was found that HeLa cells showed apoptotic features after treatment with AgNPs, such as condensed nuclei, membrane blebbing, apoptotic bodies, etc. The fluorescence from green to red shows the level of damage and death of the cell. The stained cells (green, control) were characterized as viable with no damage occurred, while the apoptotic effect started from early apoptotic (light red), mid apoptotic with condensed chromatin (bright red), late apoptotic and nonviable cells (dark red) (). With increasing concentration of AgNPs, the change in fluorescence switched from greenish to dark red, evidently described that the cells are going to die. In case of plant extracts only, no such features were observed even at the higher concentration tested (). Thus, these were our preliminary findings that the AgNPs capped with bioactive components of JSC-1 extract possess potential to cure the deadly cancer. An in-depth study is required to further evaluate the molecular changes occurred in these cells and also mechanism of action of the said AgNPs against HeLa cell line and other cancerous cells [Citation41,Citation42].
Analysis of specificity and cellular uptake of AgNPs by HeLa cell line
Specificity of the as prepared AgNPs for HeLa cells was determined after treating the normal cells with different concentrations of AgNPs. The bio-synthesized AgNPs were well tolerated by normal human cells and did not caused any significant toxicity up to 80 µg ml−1. However, about 30% mortality was observed at the highest dose (160 µg ml−1) of AgNPs (). This enhanced anticancer activity and specificity of biogenic AgNPs for cancerous cells while low cytotoxicity to normal cells could be of significant importance in the treatment of cancer cells. This specificity could be attributed to the less compact cellular architecture of cancerous cells that allow penetration of AgNPs into cytoplasm of the cells. Similar findings have been reported by other researchers [Citation20,Citation43,Citation44].
Figure 9. Normal cells viability after treatment with different concentrations of AgNPs as determined by MTT assay (A). Intracellular accumulation of AgNPs by HeLa cells using ICP-AES (B). Errors bars indicate that the experiment were carried out in triplicates and where p ≤ .05.
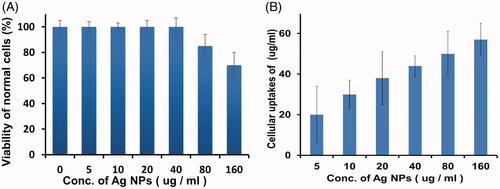
On the other hand, ICP-AES studies were carried out to confirm the cellular uptake of AgNPs by HeLa cells. It was observed that increasing the dose of AgNPs the amount of intracellular AgNPs accumulation were increased (). ICP-AES data suggested that diffusion might be the probable mechanism of AgNPs’ entry into the cells; thus, it might lead the cancer cell to display apoptotic features and ultimately dies. A previous study indicated that AgNPs (under cell culture condition) displayed lesser endocytosis and clathrin pits formation [Citation20].
Dissolution of silver ions from AgNPs
The cytotoxicity of AgNPs is basically associated with the dissolution and release of toxic silver ions (Ag+) in biological media. The release of Ag+ depend upon the pH value and O2 concentration in the medium and is also affected by the availability of reducing sugars, chlorides, sulphides and sulphur containing components. The mechanism of dissolution of AgNPs in biological media, either cell culture media or in cells has hardly been investigated. However, preliminary studies on the dissolution of AgNPs in NaCl solution (10 mM) viz far below the isotonic concentration (154 mM) indicate that even under such mild conditions quick degradation of the particles occur. In general, the dissolution of NPs can be ascribed to their surface functionalization. It is well known that AgNPs can be oxidized in aqueous solutions exposed to air (EquationEquation (1)(1)
(1) ) resulting in the release of Ag+ under acidic conditions (EquationEquation (2)
(2)
(2) ) [Citation45,Citation46].
(1)
(1)
(2)
(2)
Conclusion
The bioactive components in the extract of cyanobacteria Leptolyngbya JSC-1 have great potential to be used as reducing and capping agents for biosynthesis of stable AgNPs. As mentioned in our previous study of gold nanoparticles synthesis with the same extracts, it was observed that the JSC-1 extract has a strong redox potential which can be exploited for the synthesis of a variety of metal NPs. Currently, the as prepared AgNPs have strong antibacterial activities against both E. coli and S. aureus. The most important aspect of this study was the application of the biogenic AgNPs against the deadly cancer (HeLa cell line). Our preliminary findings unveil that the AgNPs capped with JSC-1 extracts depicted excellent anticancer activity against HeLa cells. This might be an area of interest for other researchers to further investigate and disclose more important findings.
Disclosure statement
All the authors declare that there is no potential conflict of interests.
Additional information
Funding
References
- Saleh T, Ahmed E, Yu L, et al. Silver nanoparticles improve structural stability and biocompatibility of decellularized porcine liver. Artif Cell Nanomed B. 2018. Available from: https://doi.org/10.1080/21691401.2018.1457037
- Shankar SS, Rai A, Ahmad A, et al. Rapid synthesis of Au, Ag, and bimetallic Au core–Ag shell nanoparticles using Neem (Azadirachta indica) leaf broth. J Colloid Interf Sci. 2004;275:496–502.
- Nadagouda MN, Varma RS. Green synthesis of silver and palladium nanoparticles at room temperature using coffee and tea extract. Green Chem. 2008;10:859–862.
- Barabadi H, Ovais M, Shinwari ZK, et al. Anti-cancer green bionanomaterials: present status and future prospects. Green Chem Lett Rev. 2017;10:285–314.
- Singh M, Kalaivani R, Manikandan S, et al. Facile green synthesis of variable metallic gold nanoparticle using Padina gymnospora, a brown marine macroalga. Appl Nanosci. 2013;3:145–151.
- Lewis LN. Chemical catalysis by colloids and clusters. Chem Rev. 1993;93:2693–2730.
- Zada S, Ahmad A, Khan S, et al. Biofabrication of gold nanoparticles by Lyptolyngbya JSC-1 extract as super reducing and stabilizing agents: Synthesis, characterization and antibacterial activity. Microb Pathogenesis. 2018;114:116–123.
- Dumur F, Guerlin A, Dumas E, et al. Controlled spontaneous generation of gold nanoparticles assisted by dual reducing and capping agents. Gold Bull. 2011;44:119–137.
- Inbakandan D, Venkatesan R, Khan SA. Biosynthesis of gold nanoparticles utilizing marine sponge Acanthella elongata (Dendy, 1905). Colloid Surface B. 2010;81:634–639.
- Kim S-K. Handbook of marine macroalgae: biotechnology and applied phycology. New Jersey: John Wiley & Sons; 2011.
- Song JY, Kim BS. Rapid biological synthesis of silver nanoparticles using plant leaf extracts. Bioprocess Biosyst Eng. 2009;32:79.
- Gadd GM. Heavy metal accumulation by bacteria and other microorganisms. Experientia 1990;46:834–840.
- Rajeshkumar S, Malarkodi C, Paulkumar K, et al. Algae mediated green fabrication of silver nanoparticles and examination of its antifungal activity against clinical pathogens. Int J Met. 2014;2014:1.
- Brown II, Bryant DA, Casamatta D, et al. Polyphasic characterization of a thermotolerant siderophilic filamentous cyanobacterium that produces intracellular iron deposits. Appl Environ Microb. 2010;76:6664–6672.
- Ahmad A, Syed F, Shah A, et al. Silver and gold nanoparticles from Sargentodoxa cuneata: synthesis, characterization and antileishmanial activity. RSC Adv. 2015;5:73793–73806.
- Ahmad A, Wei Y, Syed F, et al. Isatis tinctoria mediated synthesis of amphotericin B-bound silver nanoparticles with enhanced photoinduced antileishmanial activity: a novel green approach. J Photoch Photobio B. 2016;161:17–24.
- Dinesh D, Murugan K, Madhiyazhagan P, et al. Mosquitocidal and antibacterial activity of green-synthesized silver nanoparticles from Aloe vera extracts: towards an effective tool against the malaria vector Anopheles stephensi?. Parasitol Res. 2015;114:1519–1529.
- Baghbani-Arani F, Movagharnia R, Sharifian A, et al. Photo-catalytic, anti-bacterial, and anti-cancer properties of phyto-mediated synthesis of silver nanoparticles from Artemisia tournefortiana Rchb extract. J Photoch Photobio B. 2017;173:640–649.
- Tripathi R, Gupta RK, Shrivastav A, et al. Trichoderma koningii assisted biogenic synthesis of silver nanoparticles and evaluation of their antibacterial activity. Adv Nat Sci: Nanosci Nanotechnol. 2013;4:035005.
- Ullah S, Ahmad A, Wang A, et al. Bio-fabrication of catalytic platinum nanoparticles and their in vitro efficacy against lungs cancer cells line (A549). J Photoch Photobio B. 2017;173:368–375.
- Subbaiya R, Saravanan M, Priya AR, et al. Biomimetic synthesis of silver nanoparticles from Streptomyces atrovirens and their potential anticancer activity against human breast cancer cells. IET Nanobiotechnol. 2017;11:965–972.
- Kim D-Y, Saratale RG, Shinde S, et al. Green synthesis of silver nanoparticles using Laminaria japonica extract: Characterization and seedling growth assessment. J Clean Prod. 2018;172:2910–2918.
- Anandalakshmi K, Venugobal J, Ramasamy V. Characterization of silver nanoparticles by green synthesis method using Pedalium murex leaf extract and their antibacterial activity. Appl Nanosci. 2016;6:399–408.
- Kasithevar M, Saravanan M, Prakash P, et al. Green synthesis of silver nanoparticles using Alysicarpus monilifer leaf extract and its antibacterial activity against MRSA and CoNS isolates in HIV patients. J Interdi Nanomed. 2017;2:131–141.
- Lee S-W, Chang S-H, Lai Y-S, et al. Effect of temperature on the growth of silver nanoparticles using plasmon-mediated method under the irradiation of green LEDs. Materials 2014;7:7781–7798.
- Omar HH, Bahabri FS, El-Gendy AM. Biopotential application of synthesis nanoparticles as antimicrobial agents by using Laurencia papillosa. Int J Pharmacol. 2017;13:303–312.
- Vivek M, Kumar PS, Steffi S, et al. Biogenic silver nanoparticles by Gelidiella acerosa extract and their antifungal effects. Avicenna J Med Biotechnol 2011;3:143.
- Gnanajobitha G, Paulkumar K, Vanaja M, et al. Fruit-mediated synthesis of silver nanoparticles using Vitis vinifera and evaluation of their antimicrobial efficacy. J Nanostruct Chem. 2013;3:67.
- Roy S, Anantharaman P. Biosynthesis of Silver Nanoparticles by Chaetomorpha antennina (Bory de Saint-Vincent) Kutzing with Its Antibacterial Activity and Ecological Implication. J Nanomed Nanotechnol 2017;8:2.
- Rajeshkumar S, Malarkodi C, Gnanajobitha G, et al. Seaweed-mediated synthesis of gold nanoparticles using Turbinaria conoides and its characterization. J Nanostruct Chem. 2013;3:44.
- Roy S, Mukherjee T, Chakraborty S, et al. Biosynthesis, characterisation & antifungal activity of Silver nanoparticles synthesized by the fungus aspergillus Foetidus mtcc8876. Dig J Nanomater Bios 2013;8:197–205.
- Gurunathan S, Jeong J-K, Han JW, et al. Multidimensional effects of biologically synthesized silver nanoparticles in Helicobacter pylori, Helicobacter felis, and human lung (L132) and lung carcinoma A549 cells. Nanoscale Res Lett. 2015;10:35.
- Roda E, Barni S, Milzani A, et al. Single Silver Nanoparticle Instillation Induced Early and Persisting Moderate Cortical Damage in Rat Kidneys. Ijms. 2017;18:2115.
- Carlson C, Hussain SM, Schrand AM, et al. Unique cellular interaction of silver nanoparticles: size-dependent generation of reactive oxygen species. J Phys Chem B. 2008;112:13608–13619.
- Park H-J, Kim JY, Kim J, et al. Silver-ion-mediated reactive oxygen species generation affecting bactericidal activity. Water Res. 2009;43:1027–1032.
- Guilger M, Pasquoto-Stigliani T, Bilesky-Jose N, et al. Biogenic silver nanoparticles based on trichoderma harzianum: synthesis, characterization, toxicity evaluation and biological activity. Sci Rep. 2017;7:44421.
- Panzarini E, Mariano S, Dini L, editors. Investigations of the toxic effects of glycans-based silver nanoparticles on different types of human cells. AIP Conf Proc. New York, NY: AIP Publishing; 2017.
- Datkhile KD, Durgawale PP, Patil MN. Biogenic silver nanoparticles are equally Cytotoxic as Chemically Synthesized silver nanoparticles. Biomed Pharmacol J. 2017;10:337–344.
- Sadat Shandiz SA, Shafiee Ardestani M, Shahbazzadeh D, et al. Novel imatinib-loaded silver nanoparticles for enhanced apoptosis of human breast cancer MCF-7 cells. Artif Cell Nanomed B. 2017;45:1082–1091.
- Yakop F, Abd Ghafar SA, Yong YK, et al. Silver nanoparticles Clinacanthus nutans leaves extract induced apoptosis towards oral squamous cell carcinoma cell lines. Artif Cell Nanomed B. 2018;1:1–9.
- Dini L, Panzarini E, Serra A, et al. Synthesis and in vitro cytotoxicity of glycans-capped silver nanoparticles. Nanomater Nanotechno. 2011;1:10.
- Ovais M, Khalil AT, Raza A, et al. Green synthesis of silver nanoparticles via plant extracts: beginning a new era in cancer theranostics. Nanomedicine 2016;11: 3157–3177.
- Moulton MC, Braydich-Stolle LK, Nadagouda MN, et al. Synthesis, characterization and biocompatibility of “green” synthesized silver nanoparticles using tea polyphenols. Nanoscale 2010;2:763–770.
- Amooaghaie R, Saeri MR, Azizi M. Synthesis, characterization and biocompatibility of silver nanoparticles synthesized from Nigella sativa leaf extract in comparison with chemical silver nanoparticles. Ecotox Environ Safe. 2015;120:400–408.
- Graf C, Nordmeyer D, Sengstock C, et al. Shape-Dependent Dissolution and Cellular Uptake of Silver Nanoparticles. Langmuir 2018;34:1506–1519.
- Xiu Z-m, Zhang Q-b, Puppala HL, et al. Negligible particle-specific antibacterial activity of silver nanoparticles. Nano Lett. 2012;12:4271–4275.