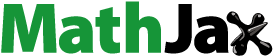
Abstract
Our previous works have shown that tanshinone IIA inhibited maladaptive extracellular matrix remodeling in cardiac fibroblasts implicating its potential role in treating of pathologic cardiac remodeling. However, the intrinsically poor solubility and bioavailability of tanshinone IIA hindered its clinical application. Here we develop monomethoxy-poly (ethylene glycol)-poly (lactic acid)-D-α-Tocopheryl polyethylene glycol 1000 succinate (mPEG-PLA-TPGS) nanoparticle incorporating tanshinone IIA (tanshinone IIA-NPs) and study its efficacy in post-infarction left ventricular (LV) remodeling. Male C57BL/6 mice underwent left coronary artery ligation followed by subsequent intravenously injected tanshinone IIA-NPs therapy for 5 consecutive days. Treatment with tanshinone IIA-NP improved cardiac function, limited infarct expansion, and prevented left ventricle dilation at 4 weeks post-MI. Furthermore, cardiomyocytes inflammation, apoptosis and myocardial fibrosis were significantly attenuated in tanshinone IIA-NP treated mice. These effects also correlated with inhibition of IκB protein phosphorylation and NF-κB activation, leading to suppression of proinflammatory cytokine expression. Together, these results demonstrate tanshinone IIA-NP attenuated adverse cardiac remodeling and dysfunction mediated through prevention of IκB phosphorylation and NF-κB activation. Tanshinone IIA-NP is a novel approach to treat myocardial IR injury in patients with MI.
Introduction
Despite advances in pharmacotherapy and non-surgical means to improve cardiac reperfusion, patients suffering from myocardial infarction (MI)continue to experience significant morbidity and mortality [Citation1]. The loss of functional myocardium within the infarcted area results in the progressive remodeling. This maladaptive remodeling causes ventricular dilation and decreased left ventricular (LV) function, which contributes to the progression of heart failure and sudden cardiac death [Citation2]. As such, numerous studies have been aimed at identifying novel pharmacological agents to attenuate or reverse this pathological process of myocardial remodeling.
In consequence of the pharmacological action in alleviating symptoms of coronary heart disease, ischemic stroke, hypertension and other vascular disorders, tanshinone IIA extracted from Salvia miltiorrhiza Bunge has received a great deal of attention in past decades [Citation3]. This plant-derived molecule containing an estrogen ring (namely, phytoestrogens), has been wildly investigated for its effects in inhibitory effects on inflammation and cardiac hypertrophy, scavenger activity on free radicals and vasodilatory potential [Citation4, Citation5]. Animal studies have shown in post-myocardial ischemic reperfusion injury, tanshinone IIA reduced levels of high mobility group box B1 protein and oxidative stress [Citation6]. Furthermore, our previous work demonstrated that tanshinone IIA potentiates its effects in elastogenesis while decreasing collagen deposition through activation of the PKA/CREB phosphorylation pathway in cultures of human cardiac fibroblasts [Citation7]. However, the clinical application of tanshinone IIA is hindered by its low solubility and slow dissolution rate, poor bioavailability, short half-life, and first pass metabolism. Several strategies have been undertaken to improve the clinical delivery of pharmacological agents with properties similar to tanshinone IIA. Notably, nanotechnology and biotechnological approaches for controlled drug delivery and release has gained significant momentum. Specifically, polymeric nanoparticles such as PLGA-PEG, chitosan, 2-hydroxypropyl-beta-cyclodextrin have been adopted as potential carriers to improve the solubility of tanshinone IIA [Citation8–10]. The polymeric composite of nanoparticle and tanshinone IIA has allowed improved dissolution and increased drug-loading capacity. Nevertheless, there lacks a nanoparticle that can deliver this therapeutic agent specifically in the context of an infarcted myocardium.
Here, we developed a nanoparticle-mediated drug delivery system using a new conjugate of diblock copolymer with a core-shell structure monomethoxy-poly (ethylene glycol)-poly (lactic acid)-D-α-Tocopheryl polyethylene glycol 1000 succinate (mPEG-PLA-TPGS) nanoparticle. Both PEG and PLA have been approved by the Food and Drug Administration for medical applications. We hypothesized that nanoparticle-mediated targeting of tanshinone IIA (tanshinone IIA-NP) with higher stability can be a novel therapeutic approach for improving adverse cardiac remodelling. We synthesized mPEG-PLA by ring opening polymerization. mPEG-PLA-TPGS copolymer was prepared by integrating the polymers with active terminal groups, and subsequently conjugating tanshinone IIA by using the thin film hydration method. The cardioprotective effects of tanshinone IIA-NPs on the post-infarct LV remodeling were assessed by echocardiographic characteristics, scar circumference and fibrosis areas. Pro-inflammatory cytokines infiltration and level of cardiomyocytes apoptosis were also examined. In order to elucidate the probable mechanisms of tanshinone IIA-NPs in attenuating cardiac remodeling, downstream signaling factors were evaluated by Western blotting and immunohistochemical analysis.
Material and methods
Materials
Isoflurane was purchased fromClipper Distributing Company (St. Joseph, MO, USA). The primary antibodies: Cleaved caspase-3 (ASP175), Bax (14796), IL-1β (12703), IL-6 (12912), MCP-1 (2029), phospho-p44/42 ERK1/2 (Thr202/Tyr204), TGFβ1 (5231), Smad3 (9523), NF-κB p65 (9460) antibodies were purchased from Cell Signaling Technology (Danvers, MA, USA). Secondary antibody fluorescein-conjugated goat anti-rabbit and fluorescein-conjugated goat anti-mouse were purchased from Sigma-Aldrich Corp. (St. Louis, MO). Anti-angiotensin II type 1 receptor antibody (ab18801), MMP-2 antibody (sc-13594) and MMP-9 antibody (sc-393859) were obtained from Santa Cruz Biotechnology (Santa Cruz, CA, USA). Mouse MCP1 (ab208979), TNFα (ab208348), IL-1β (ab100704), IL-6 (ab100712), IL-18 (ab216165) ELISA Kit were purchased from Abcam (Cambridge, MA, USA). TPGS, monomethoxy-Poly(ethylene glycol) with a molecular weight of 1500 (mPEG1500), 1,3-diisopropylcarbodiimide (DCC), succinic anhydride,triethanolamine(TEA), L-lactide, tanshinone IIA and 4-(dimethylamino) pyridine (DMAP), Stannous octoate [Sn(Oct)2] were acquired from Aladdin chemistry Co. Ltd. (Shanghai, China). All other chemicals and solvents were of analytical or chromatographic grade.
Measurements
1H NMR spectra was recorded on a superconducting Fourier transform nuclear magnetic resonance spectrometry (Bruker Company, Billerica, MA) at 400 MHz using Deuterium oxide(D2O) as a solvent. FTIR spectra were obtained by an infrared spectrometer(FTIR-650). The analysis was done in the wave number range of 500–4000 cm−1, microspheres were crushed to make KBr pellets under a hydraulic pressure of 600 kg/cm2. Mean size distribution and zeta potential were measured by dynamic light scattering (DLS) with a Zetasizer Nano ZSP system (NanoZS90). The scattering angle was fixed at 90 °C, and the measurement was carried out at a constant temperature of 25 °C. Data was obtained as the average of three measurements. The morphology of nanoparticle was observed under a transmission electron microscopy (TEM, at an accelerating voltage of 200 kV). Briefly, one drop of mixed copolymer suspension was added to a copper grid and negatively stained with a phosphotungstic acid solution (2%, w/v) for 30 s. The sample was dried in air and examined under the TEM.
Synthesis of mPEG-PLA
Triblock copolymer mPEG-PLA was prepared by the ring-opening polymerization of L-lactide (LA) in the presence of PEG and stannous octoate [Sn(Oct)2] [Citation11].
Synthesis of carboxyl-terminated copolymer PEG-PLA
Carboxyl-terminated copolymer mPEG-PLA was prepared with succinic anhydride in the presence of DMAP and trimethylamine (TEA). mPEG-PLA (1.0 g), succinic anhydride(0.023 g), DMAP (0.029 g), and triethylamine(0.030 ml) was dissolved in 1,4-dioxane (10 ml) and left overnight at room temperature. The filtered solution was precipitated by the addition of diethyl ether, and the polymer precipitate was purified by dialysis with a cellulose membrane [cutoff number-average molecular weight (Mn) = 8000] and dried in vacuo for 3 days at room temperature.
Synthesis of mPEG-PLA-TPGScopolymer
The mPEG-PLA-TPGS copolymer was prepared by integrating the polymers with active terminal groups. TPGS (0.954g), mPEG-PLA-COOH (0.513g), DCC (3 0.618g), DMAP (0.366g), and 10 ml of triethanolamine (TEA) were all dissolved in 50 ml anhydrous dichloromethane (DCM) and stirred for 24 h at room temperature under nitrogen protection. The mixture was concentrated in vacuum, precipitated in cold diethylether, and collected by filtration. To remove unreacted TPGS, the filter through was dialyzed against DCM in a dialysis tube with molecular weight cut-off at 8 kDa. The final product (mPEG-PLA-TPGS) was freeze-dried and collected.
Preparation of tanshinone IIA-loaded mixed copolymer
TAN-loaded mixed polymeric nanoparticle was prepared using the thin film hydration method. Briefly, the mixture containing 40 mg copolymers (mPEG-PLA-TPGS) and 1 mg tanshinone IIA was dissolved in acetone. The mixed solution was evaporated with a rotary vacuum until a thin film was formed. The film was freeze-dried overnight at 40 °C and rehydrated in 5 ml of phosphate buffer solution by stirring at a water bath (60 °C) for 30 min. Unencapsulated tanshinone IIA crystals were removed by filtration with a 0.22 mm filter membrane, and tanshinone IIA-loaded mixed polymeric nanoparticle (tanshinone IIA-NP) was obtained.
Determination of entrapment efficiency
To determine the weight of tanshinone IIA in tanshinone IIA-NP, the drug-loaded copolymer was dissolved in anhydrous ethanol and analyzed with UV spectrometer using a standard curve method. The drug encapsulation efficiency (EE%) and drug loading percentage (DL%) were respectively calculated by the subsequent equations:
In vitro drug release
In vitro tanshinone IIA release behaviors from tanshinone IIA-NP was studied in PBS at pH 7.4. Weighed lyophilized tanshinone IIA-NP was suspended in 2.0 ml of release medium and introduced into a dialysis bag (MWCO 8000 Da) [Citation12]. The release test was started by placing the end-sealed dialysis bag into 50.0 ml of release medium at 37 °C with continuous shaking at 100 rpm. At scheduled time points, 2.0 ml of external release medium was removed and the same volume of fresh release medium was added to the dialysis system. The amount of released tanshinone IIA was measured by UV using a standard curve method.
Induction of myocardial infarction and experimental design
All experiments were evaluated and approved by the committee on ethics on animal experiments at Guangdong Provincial Hospital of Chinese medicine and were performed according to the principles of the American Physiological Society. Myocardial infarction was induced in the C57BL/6J mice by ligating the left anterior descending coronary artery as previously described [Citation7]. Briefly, mice were anesthetized with sodium pentobarbital (50 mg/kg) intraperitoneally, intubated and mechanically ventilated with a rodent respirator, and exposed by a left thoracotomy. MI was induced by creating a slipknot (8–0 silk) around the LAD artery and 2 mm below the tip of the left atrial appendage. All animals were transferred to constant temperature pads and allowed to recover from the surgery. Following the procedure, the surviving mice were randomly allocated into 2 groups receiving vehicle (saline 0.5 ml/kg) or tanshinone IIA-NP (0.5 ml/kg) intravenously for 5 consecutive days. Echocardiographic assessment excluded mice with infarction that involved <30% of the LV free wall. To evaluate the effects of tanshinone IIA-NP on mice without infarction, sham-operated mice were designated to the matching groups 4 weeks after ligation of the left coronary artery.
Echocardiography
Mice were anesthetized with 2% isoflurane/oxygen inhalation and placed in supine position on a constant temperature pad. LV function were assessed by a Vevo 770 high-resolution digital ultrasound imaging system (Primetech Inc) and 18 MHz scanning head (MS 200) with 2-dimensional guided M-mode echocardiographic measurements at baseline and 2 days, and 4 weeks after ligation [Citation13]. LV dimension involving end-systolic diameter (ESD) and end-diastolic diameter (EDD) were gained by M-mode tracings and subsequently, LV ejection fraction (EF) and LV fractional shortening (FS) were determined [Citation14]. An average of 10 consecutive cardiac cycles was intended for each evaluation and was made 3 times in a blinded manner.
Immunoblotting analysis
Homogenate of LV myocardium sample was analyzed using immunoblotting. Briefly, frozen samples were homogenized in ice-cold lysis buffer and proteins (40 μg) per lane were separated on 8–15% SDS-polyacrylamide gels and then transferred to PVDF membranes. The protein lysates were probed with primary antibodies diluted in 5% BSA overnight at 4 °C. The blots were rinsed and subsequently incubated in horseradish peroxidase-conjugated secondary antibodies in 5% BSA for 1 h and developed using a chemiluminescent substrate. Densitometric quantifications were carried out via Image J software (version 1.44, Bethesda, Maryland, USA).
Quantitative real-time RT-PCR analysis
Reverse transcription of RNA was performed using SuperScript™ II Reverse Transcriptase with random primers following the manufacturer’s protocol. Real-time PCR was carried out via a Prism 7900HT (Applied Biosystems, Foster City, CA). The cycling conditions were: 95 °C for 10 min, (95 °C for 15 s, 60 °C, 72 °C for 1 min) for 40 cycles, followed by dissociation curve analysis. Changes in mRNA expression was normalized to 18 s mRNA levels and compared statically (ΔΔCt method) using the ABI Prism SDS 2.1 software (Foster City, USA).
Immunohistochemical analysis
Four weeks following the ligation procedure, myocardium specimens were fixed in 10% buffered-formalin overnight and embedded in paraffin or frozen with optimal cutting temperature compound. 5-μm thick sections were immunohistostained using indicated antibodies. Digital images of 5–10 fields in the specimens were observed, and the number of NF-κB p65, IL-1β, IL-6, MCP-1, and IL-18 positive cells were determined using a 40× objective under Nikon Eclipse E1000 microscope (Nikon Instruments, Tokyo, Japan).
Apoptotic nuclei were labeled by TUNEL staining using a commercial detection kit (Takara Bio, Inc., Shiga, Japan). The number of TUNEL-positive myocytes was counted and divided by total nuclei identified by DAPI staining in the same specimen.
Statistical analysis
Data are presented as the mean ± SEM. The statistical analyses of differences were determined using the Student’s t-test or ANOVA tests. The statistical tests were conducted at the 0.05 significance level using the statistical package Prism Software version 7.0 (GraphPad Software, San Diego California USA).
Results
Characterization of tanshinone IIA-NP
The synthesis of mPEG-PLA-TPGS copolymer was performed described in Supplementary Figure1. The structure of synthesized mPEG-PLA-TPGS chemical conjugate was confirmed by infrared spectroscopy and 1 H NMR spectrum. Using infrared spectroscopy, it was evident that the peaks of amide bands I and II of copolymer appeared at 1643 cm−1and 1566 cm−1, respectively (). 1 H NMR spectrum showed that proton characteristic peaks of amide bandat 8.16 ppm (). Both infrared spectroscopy and 1HNMR spectrum indicated the success conjugation of mPEG-PLA and TPGS.
Figure 1. The characterization of tanshinone IIA-NP. (A) The FTIR spectra showed that the peaks of amide bands I and II of copolymer appeared at 1643 cm−1and 1566 cm−1, respectively. (B) 1H NMR spectrum demonstrated that the proton characteristic peaks of amide band sat 8.16 ppm. (C) The size distribution spectrum of tanshinone IIA-NP was between 100 and 200 nm. (D) The zeta potential was –7.12 ± 0.07 mV. (E) Representative transmission electron micrographs of tanshinone IIA-NP. (F) The vitro release of tanII A tanshinone IIA-NP in pH =7.2 PBS at 37 °C.
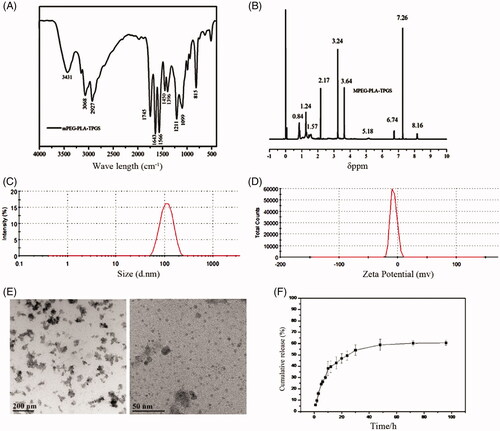
The physical characterizations of tanshinone IIA-loaded nanoparticles are shown in Supplementary Table 1. The average particle sizes of tanshinone IIA-NP is between 100 and 200 nm with acceptable polydispersity indexes (PDI <0.25) (). The zeta potential was −7.12 ± 0.07 mV ().
TEM images illustrated that tanshinone IIA-NP had homogeneous cotton-like shapes with average diameters in good agreement with DLS measurements. Tanshinone IIA-NP consisted of many small nanoparticles (). The encapsulation efficiency (EE) and drug loading capacity (DL) of tanshinone IIA-NP were 61.30 ± 1.96% and 1.48 ± 0.12%, respectively (Supplementary Table 1).
In vitro release data showed that tanshinone IIA-NP displayed a release pattern in deionized water with ∼60% tanshinone IIA (Supplementary Figure 2) released from tanshinone IIA-loaded copolymer nanoparticle during 96 h incubation (). Furthermore, tanshinone IIA-NP was shown not to influence the cell viability in vitro (Supplementary Figure 3).
Figure 2. Effects of tanshinone IIA-NP on cardiac function after MI. Representative photographs of M-mode echocardiography and quantitative analysis of LV dimension and systolic function in mice exposed to shamoperation or coronary artery ligation with or without tanshinone IIA-NP treatment. (n = 12). LVEDD: LV end-diastolic diameter; LVESD: LV end-systolic diameter; FS: fractional shortening. #p < .05 versus Sham; *p < .05 versus MI.
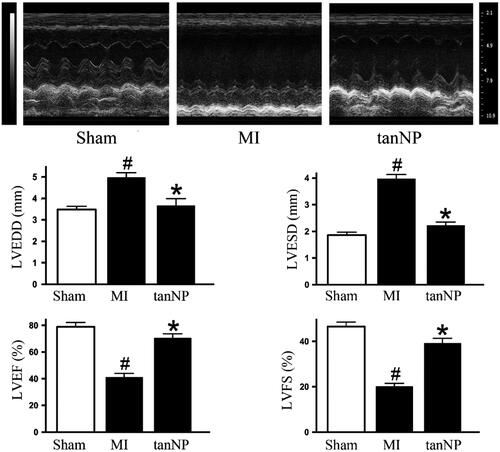
Figure 3. Effects of tanshinone IIA-NP on cardiac remodeling after MI. (A) Representative global heart photographs and the Heart weight/body weight ratio (HW/BW: mg/g) were shown (scale bar = 2 mm). (B,C): Representative images of Masson trichrome staining at 4 weeks after ligation in mice treated with or without tanshinone IIA-NP. The scar circumference (scale bar = 1 mm) and percentage of fibrotic area (scale bar = 100 µm) in circumference is shown in right panels (n = 6). #p < .05 versus Sham; *p < .05 versus MI.
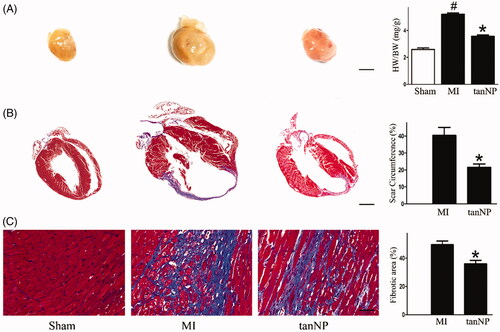
Tanshinone IIA-N preservedcardiac function after MI
To substantiate the role of tanshinone IIA-NP in cardiac function at 4 weeks following LAD ligation, echocardiogram data showed both LVEDD and LVESD significantly enlarged in MI group (both p < .05). In contrast, treatment with tanshinone IIA-NP reduced LV dilation, as shown by LVEDD and LVESD 4 weeks after MI (both p < .05, ). Tanshinone IIA-NP attenuated the deterioration in LVEF and LVFS at 4 weeks after MI ( and Supplementary Table 2). Treatment with tanshinone IIA-NP did not affect hemodynamic parameters including systolic blood pressure and heart rate (Supplementary Table 2).
Tanshinone IIA-NP improved cardiac remodeling after MI
In MI group mice at 4 weeks following LAD ligation, the heart mass index (ratio of heart weight to body weight) an extent of cardiac hypertrophy, was considerably increased compared to sham group (p < .05). However, this ratio was significantly decreased in tanshinone IIA-NP treated mice (16% decrease, p < .05, ).
In parallel, tanshinone IIA-NP treatment reduced the scar circumference (21%) and area of fibrosis (32%), supporting the limitation of LV infarct expansion (p < .05, respectively).
Next, we intravenously injected tanshinone IIA solution (1 mg/kg, diluted in DMAO) for only 5 consecutive days to evaluate the efficacy on LV remodeling after MI. Echocardiographic parameters (Supplementary Table 2) and histological analyses (Supplementary Figure 4) demonstrated no significant effect of tanshinone IIA on LV remodeling. We increased the dose of tanshinone IIA to 10 mg/kg, but there were no significant anti-remodeling effects (Supplementary Figure 4). We did not test 50 mg/kg tanshinone IIA because it was insoluble when prepared as a 200 μL solution.
Figure 4. Effects of tanshinone IIA-NP on apoptosis after MI. (A) Western blotting assay of Caspase-3, Bax and Bcl-2. Expression of β-actin served as a loading control. Representative blots from 3 independent experiments are shown. (B) TUNEL staining pictures, in which brown stained cells were TUNEL positive 4 weeks after ligation. Scale bar, 100 μm. Quantitative analysis of TUNEL-positive cells is shown in right panel (n = 6). #p < .05 versus Sham; *p < .05 versus MI.
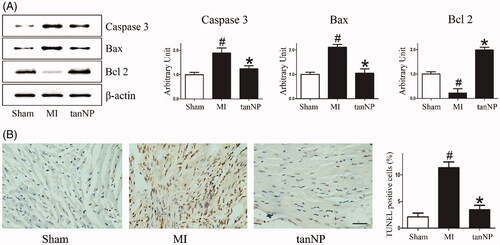
Tanshinone IIA-NP decreased cardiomyocyte apoptosis in post-infarct myocardium
Cardiomyocyte apoptosis after ischemia contributes into ventricular remodeling and subsequent systolic and diastolic dysfunction [Citation15]. To identify whether tanshinone IIA-NP protects cardiomyocytes from post-MI apoptosis, we assessed cleaved caspase3, Bax, and Bcl2 expression using immunoblotting analysis. The cleaved caspase 3 and Bax levels were about 0.63, or 0.65-fold lower (p < .05), on the contrary, Bcl-2 was about 7.8 folds increase (p < .05) in the tanshinone IIA-NP group than the MI group ().
We examined TUNEL staining in the remote area of post-infarct myocardium as a marker of apoptosis. TUNEL-positive cells were distinguished in the myocardium specimen in saline-treated animals. Treatment with tanshinone IIA-NP significantly reduced the amount of TUNEL-positive cardiomyocytes (). These findings suggest that tanshinone IIA-NP inhibited apoptotic cardiomyocyte death and adverse cardiac remodeling after MI.
Tanshinone IIA-NP reduced the expression of inflammatory factors in post-infarct heart
The inflammatory response in the ischemic myocardium has been shown to play a crucial role in the pathogenesis of cardiomyocyte apoptosis and fibrosis following MI [Citation16]. Mice subjected to LAD artery ligation demonstrated an increase in cardiac inflammatory markers: TNFα, IL-1β, IL-6, MCP1 and IL-18 mRNA levels ().
Figure 5. Effects of tanshinone IIA-NP on inflammatory factors after MI. (A) The mRNA level of inflammatory factors were analyzed by Real-time PCR in LV ischemic border zone 4 weeks after ligation. Data are shown as the ratio of mRNA level of each factors relative to 18S mRNA and expressed as the fold change relative to the mean value of sham-operated mice (n = 6). (B) ELISA assay to quantify the production of the inflammatory factors by the hearts after LAD ligation.(C) Representative immunohistochemical photographs and quantification assay of the ischemic border zone 4 weeks after LAD ligation. #p < .05 versus Sham; *p < .05 versus MI.
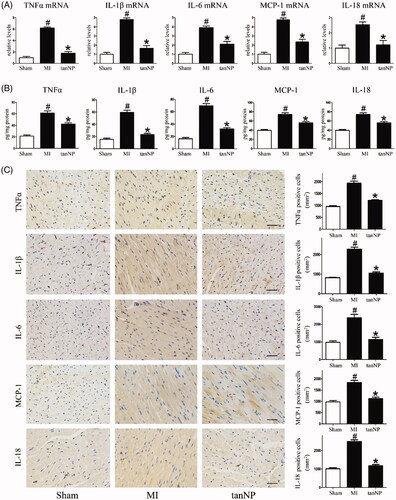
Subsequently, this inflammatory response was characterized by TNFα, IL-1β, IL-6, MCP1 and IL-18expression in myocardium as shown in the immunoblotting analysis and representative pictures immunostained for inflammatory markers (). Treatment with tanshinone IIA-NP showed a decrease in inflammatory markers post ligation (p < .05).
Tanshinone IIA-NP attenuated profibrotic markers in post-infarct heart
It has previously been suggested that ischemia mediated cardiac fibrotic processes [Citation17]. To assess the role of tanshinone IIA-NP in fibrosis post-MI, levels of TGFβ1 and SMAD3 were assessed using immunoblots. In mice treated with tanshinone IIA-NP, the activated forms of both profibrotic markers were significantly suppressed (, p < .05).
Figure 6. Effects of tanshinone IIA-NP on profibrotic markers after MI. (A) Immunoblotting analysis assessed profibrotic markers expression in ischemic border zone 4 weeks after explosion to coronary artery ligation. Quantitative analysis is shown in right panels (n = 6). (B) Representative of myocardial sections stained with profibrotic markers. Quantitative analysis was shown in right panel (n = 6). Scale bar, 100 μm. #p < .05 versus Sham; *p < .05 versus MI.
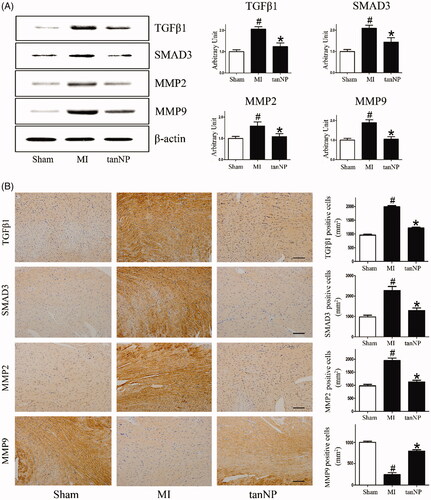
Both MMP2 and MMP9 also play important roles in cardiac rupture and remodeling after MI [Citation18]. We assessed the expression of MMP2 and MMP9 in infarcted hearts. Consist with previous reports, MMPs production were significantly increased in the infarct area, however tanshinone IIA-NP administration significantly decreased MMPs expression (< .05).
In parallel, analysis of immune-detectable TGFβ1, SMAD3, MMP2, and MMP9 with respective antibodies in myocardium further supports the evidence that tanshinone IIA-NP eliminates the profibrotic factors while up-regulating anti-fibrotic factors ().
Tanshinone IIA-NP regulated IκB/NFκB activation
We further aimed to uncover the cardioprotective mechanisms of tanshinone IIA-NP. An increasing number of experimental studies and clinical trials have testified that the inhibition of angiotensin II type 1 receptor (AR1) attenuates LV remodeling and improves symptoms of heart failure [Citation19]. Our previous work has shown that in cultured human cardiac fibroblasts tanshinone IIA inhibits angiotensin II-induced extracellular matrix remodeling via downregulation the AR1 pathway [Citation20]. We therefore questioned whether AR1 was target molecules of tanshinone IIA-NP. Indeed, treatment with tanshinone IIA-NP suppressed the upregulation of AR1at 4 weeks in mice after ligation (p < .05, Supplementary Figure 5).
Moreover, AR1 had been described to phosphorylate ERK, which degrades IκB kinase (IκB). As a consequence of IκB degradation, it promoted the function of NF-κB p65, a transcription factor that up-regulates proinflammatory and profibrotic factors [Citation21]. To further define the downstream signal effects of tanshinone IIA-NP, we focused our attention on the NF-κB pathway. Following MI, significant activation of phospho-ERK and phospho-IκB was observed, however, their levels were restored hearts treated with tanshinone IIA-NP (p < .05, Supplementary Figure 5). Degradation of IκB unbinds NF-κB dimers which in turn translocate to the nucleus and promote transcription. Nuclear levels of NF-κB p65 was significantly elevated in the MI group but reduced in tanshinone IIA-NP treated group (Supplementary Figure 5). These results supported that tanshinone IIA-NP exerts cardioprotective effects via inhibition of IκB phosphorylation and consequent NF-κB activation.
Discussion
Previous studies established that treatment with high dose of tanshinone IIA (20–50 mg/kg) improved LV function in mice with acute MI [Citation22]. However, its wide therapeutic applications have been slowed down by its intrinsic poor solubility and short half-life. Nanoparticle-mediated drug delivers stand at the forefront of nanomedicine-developing studies. Because they can overcome several shortcomings of conventional drug delivery manners, such as low water solubility and non-specific biodistribution. Recently, Jia and his colleague have prepared solid dispersions (SDs) of tanshinone IIA with silica nanoparticles, characterization and in vivo evaluation results showed that the silica nanoparticle based SDs achieved complete dissolution, increased absorption rate, maintained drug stability, and improved oral bioavailability compared to tanshinone IIA alone [Citation23]. Coincidentally, Vincent designed a new anti-cancer medicine delivery system to delivery tanshinone IIA and α-mangostin from gold/PEI/cyclodextrin nanoparticle system [Citation24]. In vitro preliminary cell viability assays against prostate cancer cell lines PC-3 and DU145 showed that encapsulation resulted in increased cytotoxicity.
In the present study, we confirmed that mPEG-PLA-TPGS nanoparticle containing 1 mg/kg tanshinone IIA attenuated post-infarct LV enlargement and maladaptive dysfunction. The attenuation of myocardial remodeling in tanshinone IIA-NP treated mice was mediated via IκB and inhibition of NF-κB pathway, leading to suppression of inflammatory responses in the post-infarct myocardium. As shown in Supplementary Table 2 and Figure 2, intravenous administration of 1 and 10 mg/kg tanshinone IIA solution for only 5 times was not effective, although 1 mg/kg tanshinone IIA-NPs showed efficacy. Importantly, the lower concentration tanshinone IIA-NP treatment did not influence the cell viability in vitro nor the myocardium immediately following coronary artery ligation. Treatment with tanshinone IIA-NP resulted in significant reduction in LV dilatation, myocardial fibrosis and infarct area making the infarct heart less susceptible to the influence of mechanical forces that exacerbate the remodeling pathological process.
Following an MI, loss of myocardium due to apoptosis is related to ventricular dilatation and progression to heart failure [Citation25]. Cardiomyocyte apoptosis has been shown to increase the infarct zone expansion and maladaptive hypertrophy, together with impairing systolic and diastolic function [Citation13]. Studies suggested that cardiomyocyte-specific NF-κB p65 deletion protects mice heart from apoptosis induced by either global ischemic reperfusion injury by Langendorff or regional ischemic reperfusion injury by coronary ligation and release [Citation26]. In support of this data, tanshinone IIA-NP treated mice showed reduced cardiac apoptosis via decreased expression of cleaved caspase-3 and Bax in addition to inhibition NF-κB p65.
The present research provides an additional mechanism that tanshinone IIA-NP generates IκB protein activation, leading to NF-κB degradation in the post-infarcted myocardium. It is acknowledged that the NF-κB pathway plays a key role in mediating inflammatory responses via promoting the expression of proinflammatory cytokines. In line with the alteration of IκB and NF-κB activity, our reports demonstrated that the level of TNFα, IL-1β, IL-6, MCP1 and IL-18 was significantly attenuated in the tanshinone IIA-NP group, which correlated with a reduction of infiltration of inflammatory cells in the post-MI myocardium. Excluding its role as an inflammatory mediator, various studies have identified NF-κB as a facilitator of myocardial injury [Citation27]. We have previously shown that NF-κB provoked adverse extracellular matrix remodeling induced by angiotensin II [Citation20]. Other studies have also shown the suppression of NF-κB pathway is a substantial therapeutic strategy for cardioprotection from ischemic injury [Citation28]. Inhibition NF-κB by cardiac-specific overexpression of IκBα, subsequently preventing the translocation of the p65/p50 dimer, has demonstrated a causal role in reducing the cardiac injury response to ischemia [Citation29]. Coincident with these reports, our present data demonstrated that tanshinone IIA-NP exerted its cardioprotective mechanisms via inhibition in IκB degradation and NF-κB p65 expression.
Additionally, the proinflammatory factors released from cardiomyocytes not only act in autocrine signaling to promote apoptosis and hypertrophy, but also in paracrine signaling to stimulate the release of profibrotic molecules from neighboring myofibroblasts and macrophages which are critical in the process of pathologic remodeling [Citation30]. Moreover, the promoter region of MMP9 gene contains a NF-κB binding site, mutations in NF-κB binding sites can reduce the induction of MMP9 [Citation31]. MMPs had been implicated in the progression of myocardium ischemic lesions that contribute to the expansion of the infarct zone contributing into aneurysm or even ventricular rupture. Thus, there is considerable interest in defining approaches to limit these processes. Here, we show tanshinone IIA-NP inhibited the expression of MMP2/9 protein. This finding reconciles with the observation whereby tanshinone IIA treatment prevented cardiac rupture in mice subjected to myocardial infarction [Citation32].
Conclusion
In summary, we demonstrated a novel delivery mechanism of a therapeutic agent, tanshinone IIA-NP, which prevented IκB phosphorylation and subsequent NF-κB activation resulting in the suppression of inflammatory responses and reduction of cardiomyocytes apoptosis and fibrotic process, contributing to the mitigation of LV remodeling and improvement of cardiac functions following MI. Inspired by this supportive evidence, we will conduct phase I/II clinical trials to assess the effectiveness of tanshinone IIA-NP on the cardiac function and incidence of major adverse cardiac events of patients following MI in not far future.
Supplementary_0415.docx
Download MS Word (1.2 MB)Acknowledgments
The authors gratefully acknowledge the contributions of all the investigators of the program.
Disclosure statement
No potential conflict of interest was reported by the authors.
Additional information
Funding
Reference
- Sanz-Ruiz R, Casado Plasencia A, Borlado LR, et al. Rationale and design of a clinical trial to evaluate the safety and efficacy of intracoronary infusion of allogeneic human cardiac stem cells in patients with acute myocardial infarction and left ventricular dysfunction: The randomized multicenter double-blind controlled CAREMI trial (cardiac stem cells in patients with acute myocardial infarction). Circ Res. 2017;121:71–80.
- Ezekowitz JA, Armstrong PW, Granger CB, et al. Predicting chronic left ventricular dysfunction 90 days after ST-segment elevation myocardial infarction: an assessment of pexelizumab in acute myocardial infarction (APEX-AMI) substudy. Am Heart J. 2010;160:272–278.
- Gao S, Liu Z, Li H, et al. Cardiovascular actions and therapeutic potential of tanshinone IIA. Atherosclerosis. 2012;220:3–10.
- Yang JX, Pan YY, Ge JH, et al. Tanshinone IIA attenuates TNF-alpha-induced expression of VCAM-1 and ICAM-1 in endothelial progenitor cells by blocking activation of NF-kappaB. Cell Physiol Biochem. 2016;40:195–206.
- Feng J, Li S, Chen H. Tanshinone IIA inhibits myocardial remodeling induced by pressure overload via suppressing oxidative stress and inflammation: possible role of silent information regulator 1. Eur J Pharmacol. 2016;791:632–639.
- Hu H, Zhai C, Qian G, et al. Protective effects of tanshinone IIA on myocardial ischemia reperfusion injury by reducing oxidative stress, HMGB1 expression, and inflammatory reaction. Pharm Biol. 2015;53:1752–1758.
- Mao S, Wang Y, Zhang M, et al. Phytoestrogen, tanshinone IIA diminishes collagen deposition and stimulates new elastogenesis in cultures of human cardiac fibroblasts. Exp Cell Res. 2014;323:189–197.
- Wang Q, Wei N, Liu X, et al. Enhancement of the bioavailability of a novel anticancer compound (acetyltanshinone IIA) by encapsulation within mPEG-PLGA nanoparticles: a study of formulation optimization, toxicity, and pharmacokinetics. Oncotarget. 2017;8:12013–12030.
- Liu QY, Zhang ZH, Jin X, et al. Enhanced dissolution and oral bioavailability of tanshinone IIA base by solid dispersion system with low-molecular-weight chitosan. J Pharm Pharmacol. 2013;65:839–846.
- Wang L, Jiang X, Xu W, et al. Complexation of tanshinone IIA with 2-hydroxypropyl-beta-cyclodextrin: effect on aqueous solubility, dissolution rate, and intestinal absorption behavior in rats. Int J Pharm. 2007;341:58–67.
- Sah AK, Vyas A, Suresh PK, et al. Application of nanocarrier-based drug delivery system in treatment of oral cancer. Artif Cells Nanomed Biotechnol. 2018;46:650–657.
- Du J, Singh H, Yi T-H. Biosynthesis of silver nanoparticles by Novosphingobium sp. THG-C3 and their antimicrobial potential. Artif Cells Nanomed Biotechnol. 2017;45:211–217.
- van Empel VP, Bertrand AT, Hofstra L, et al. Myocyte apoptosis in heart failure. Cardiovasc Res. 2005;67:21–29.
- Qi J, Yu J, Tan Y, et al. Mechanisms of Chinese medicine xinmailong's protection against heart failure in pressure-overloaded mice and cultured cardiomyocytes. Sci Rep. 2017;7:42843.
- Wu G, Yu W, Zhang M, et al. MicroRNA-145-3p suppresses proliferation and promotes apotosis and autophagy of osteosarcoma cell by targeting HDAC4. Artif Cells Nanomed Biotechnol. 2018;1–8.doi:10.1080/21691401.2018.1464459.
- Suthahar N, Meijers WC, Sillje HHW, et al. From inflammation to fibrosis-molecular and cellular mechanisms of myocardial tissue remodelling and perspectives on differential treatment opportunities. Curr Heart Fail Rep. 2017;14:235–250.
- Liu G, Ma C, Yang H, et al. Transforming growth factor β and its role in heart disease. Exp Ther Med. 2017;13:2123–2128.
- Cau SB, Guimaraes DA, Rizzi E, et al. The nuclear factor kappaB inhibitor pyrrolidine dithiocarbamate prevents cardiac remodelling and matrix metalloproteinase-2 up-regulation in renovascular hypertension. Basic Clin Pharmacol Toxicol. 2015;117:234–241.
- Hollenberg NK. A randomized trial of the angiotensin-receptor blocker valsartan in chronic heart failure. Curr Hypertens Rep. 2002;4:411.
- Mao S, Li W, Qa'aty N, et al. Tanshinone IIA inhibits angiotensin II induced extracellular matrix remodeling in human cardiac fibroblasts–Implications for treatment of pathologic cardiac remodeling. Int J Cardiol. 2016;202:110–117.
- Kang YM, Ma Y, Zheng JP, et al. Brain nuclear factor-kappa B activation contributes to neurohumoral excitation in angiotensin II-induced hypertension. Cardiovasc Res. 2009;82:503–512.
- Pan C, Lou L, Huo Y, et al. Salvianolic acid B and tanshinone IIA attenuate myocardial ischemia injury in mice by NO production through multiple pathways. Ther Adv Cardiovasc Dis. 2011;5:99–111.
- Jiang YR, Zhang ZH, Liu QY, et al. Preparation, characterization, and in vivo evaluation of tanshinone IIA solid dispersions with silica nanoparticles. Int J Nanomed. 2013;8:2285–2293.
- Qiu S, Granet R, Mbakidi JP, et al. Delivery of tanshinone IIA and alpha-mangostin from gold/PEI/cyclodextrin nanoparticle platform designed for prostate cancer chemotherapy. Bioorg Med Chem Lett. 2016;26:2503–2506.
- Takemura G, Fujiwara H. Role of apoptosis in remodeling after myocardial infarction. Pharmacol Ther. 2004;104:1–16.
- Zhang XQ, Tang R, Li L, et al. Cardiomyocyte-specific p65 NF-kappaB deletion protects the injured heart by preservation of calcium handling. Am J Physiol Heart Circ Physiol. 2013;305:H1089–H1097.
- Gordon JW, Shaw JA, Kirshenbaum LA. Multiple facets of NF-κB in the heart: to be or not to NF-κB. Circ Res. 2011;108:1122–1132.
- Camandola S, Cutler RG, Gary DS, et al. Suppression of calcium release from inositol 1,4,5-trisphosphate-sensitive stores mediates the anti-apoptotic function of nuclear factor-kappaB. J Biol Chem. 2005;280:22287–22296.
- Haudek SB, Spencer E, Bryant DD, et al. Overexpression of cardiac I-kappaBalpha prevents endotoxin-induced myocardial dysfunction. Am J Physiol Heart Circ Physiol. 2001;280:H962–H968.
- Dobaczewski M, Gonzalez-Quesada C, Frangogiannis NG. The extracellular matrix as a modulator of the inflammatory and reparative response following myocardial infarction. J Mol Cell Cardiol. 2010;48:504–511.
- Li GQ, Zhang Y, Liu D, et al. Celastrol inhibits interleukin-17A-stimulated rheumatoid fibroblast-like synoviocyte migration and invasion through suppression of NF-kappaB-mediated matrix metalloproteinase-9 expression. Int Immunopharmacol. 2012;14:422–431.
- Zhang Y, Wei L, Sun D, et al. Tanshinone IIA pretreatment protects myocardium against ischaemia/reperfusion injury through the phosphatidylinositol 3-kinase/Akt-dependent pathway in diabetic rats. Diabetes Obes Metab. 2010;12:316–322.