Abstract
Background
Combinations of natural products with low toxicities using tumor-targeting carriers may improve cancer treatment. The combined parthenolide and ginsenoside compound K (CK) within tLyp-1 liposomes, with the aim of improving the efficacy of lung cancer treatment.
Results
In vitro studies in A549 human pulmonary adenocarcinoma cells demonstrated that parthenolide/CK tLyp-1 liposomes increased reactive oxygen species levels and induced mitochondrial apoptosis. It enters into cells via receptor-mediated uptake and micropinocytosis, followed by endosomal/lysosomal escape. In vivo studies illustrated that it produced a greater antitumor effect than combined administration of these compounds, with minimal toxicity.
Conclusion
The findings of this study indicated that combined application of natural products in nanocarriers could offer attractive therapeutic options.
Abbreviations | ||
ROS | = | intracellular reactive oxygen species |
CK | = | ginsenoside compound K |
MTT | = | 3-(4,5-dimethylthiazol-2-yl)-2,5-diphenyltetrazolium bromide |
IC50 | = | 50% inhibitory concentration |
DSPE-PEG2000 | = | 1,2-distearoyl-sn-glycero-3-phosphoethanolamine-N-[amino(polyethylene glycol)-2000] |
DiR | = | 1,1′-dioctadecyl-3,3,3′,3′-tetramethylindotricarbocyanine iodide |
C-6 | = | coumarin-6 |
CK/parthenolide PEG Liposomes | = | DSPE-PEG2000 modified parthenolide and CK liposomes |
CK/parthenolide tLyp-1 Liposomes | = | DSPE-PEG2000-tLyp-1 modified parthenolide and CK liposomes |
C-6 PEG Liposomes | = | DSPE-PEG2000 modified coumarin-6 liposomes |
C-6 tLyp-1 Liposomes | = | DSPE-PEG2000-tLyp-1 modified coumarin-6 liposomes |
DiR PEG Liposomes | = | DSPE-PEG2000 modified 1,1′-dioctadecyl-3,3,3′,3′-tetramethylindotricarbocyanine iodide liposomes |
DiR tLyp-1 Liposomes | = | DSPE-PEG2000-tLyp-1 modified 1,1′-dioctadecyl-3,3,3′,3′-tetramethylindotricarbocyanine iodide liposomes |
PBS | = | phosphate-buffered saline |
MMP | = | mitochondrial membrane potential |
JC-1 | = | 5,5′,6,6′-tetrachloro-1,1′,3,3′-tetraethyl-imidacarbocyanine |
CI | = | cooperativity index |
EPR | = | enhanced permeability and retention effect |
Background
Chemotherapy is the primary treatment option for cancer patients and is used to control cancer growth. However, although chemotherapeutics can usually produce antitumor effects, these agents do not discriminate effectively between healthy and cancerous cells. This can result in side effects such as arrest of bone marrow, gastrointestinal reactions, and phlebophlogosis; these can impact on the quality of life and limit their use.
In order to reduce the side effects of these drugs and improve their pharmacological efficacy, a series of tumor-targeted preparations such as liposomes and polymeric micelles have been developed. Liposomes are spherical structures that are composed of concentric lipid bilayers surrounding discrete aqueous spaces. These present several distinctive characteristics that allow them to improve drug solubility, carry large drug payloads, and show biocompatibility. Some of these preparations have been applied successfully in the clinic or in clinical trials, including doxorubicin liposomes (Myocet®) [Citation1], vincristine sulfate liposomes (Marqibo®) [Citation2], irinotecan liposomes (Onivyde®) [Citation3], and paclitaxel liposomes. A cytarabine/daunorubicin liposome preparation (CPX-351) was approved by the Food and Drug Administration and has been used to treat leukemia since August 2017, indicating the wide prospects for the application of combined drug and liposome preparations in tumor-targeted therapies.
Several natural antitumor products have been used clinically including paclitaxel, cantharidin, vincristine, and arsenic trioxide. Although these have all exhibited antitumor effects, serious side effects have restricted their application. Even targeted preparations of these compounds may exhibit some side effects [Citation4,Citation5]. Natural products with lower levels of toxicity have now been investigated, including parthenolide, ginsenoside compound K (CK, as shown in ), ginsenoside Rh2, ginsenoside Rg3, artemisinin, and curcumin [Citation6,Citation7]. Parthenolide (as shown in ) is the main active compound of Tanacetum parthenium, which is widely applied in Mexico and India, and has recently been employed as an antitumor agent [Citation8–15]. The antitumor effects of parthenolide involved suppression of the B-Raf/mitogen-activated protein kinase pathway [Citation16], blockade of nuclear factor-κB activation [Citation17], and inhibition of phosphoinositide 3-kinase/protein kinase B signaling [Citation18]. Panax ginseng C. A. Meyer has been used for nearly 5000 years in oriental medical traditions and more recently in western medicine [Citation19]. As the major active compound present in ginseng, CK can block glycogen synthase kinase 3β signaling [Citation20], inhibit sphingosine kinase-1 [Citation21], decrease the levels of reactive oxygen species [Citation22], and increase the cisplatin sensitivity of cancer cells when co-administered with cisplatin [Citation23].
Figure 1. (A) The chemical structures of CK and parthenolide. The effects of (B) CK or (C) parthenolide on A549 cellular proliferation. (D) The cooperativity index (CI) of parthenolide (1.5 μg/mL) combined with the indicated concentrations of CK. (E) Cells were photographed after treated with CK and parthenolide (at ratios weight = 5:1) for inhibited cellular proliferation evaluation.
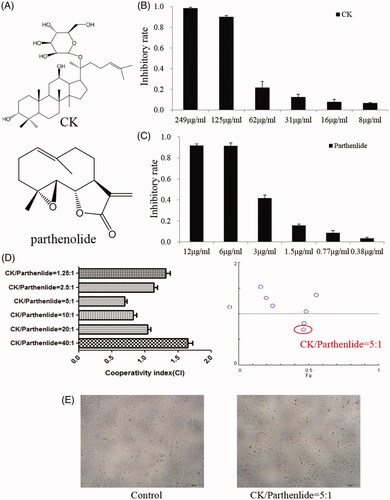
When used individually, the antitumor effects of parthenolide or CK are limited. A combination of parthenolide and CK may produce greater antitumor activity. However, the low solubility, short half-life, and poor tumor targeting may restrict the concentrations of these compounds in tumor tissues and thus limit their effects [Citation24,Citation25]. Liposomes provide a promising delivery vehicle to address these limitations and increase the antitumor effects of parthenolide and CK.
The novel tumor-homing peptide, tLyp-1 (sequence CGNKRTR), has been demonstrated to mediate tissue penetration. This could overcome the problem caused by dysfunctional tumor vessels and high interstitial pressure. When coated onto the liposome surface, this peptide could selectively target neuropilin-1 on the surface of lung cancer cells, thus facilitating the delivery of parthenolide and CK to the tumor [Citation26,Citation27].
Therefore, this study developed tLyp-1-decorated liposomes loaded with parthenolide and CK as an active tumor targeting system. The drug loading characteristics of this combination and the targeting effect of tLyp-1 liposomes were evaluated, as well as their anti-lung cancer mechanism, efficacy, and preliminary safety in tumor-bearing mice. This study aimed to provide new research ideas and an experimental basis for the use of less toxic natural products as antitumor agents, and to provide a new approach to improving cancer treatment by reducing adverse effects.
Material and methods
Materials
Ginsenoside CK and parthenolide were obtained from Xi’an XiaoCao Botanical Development Co., Ltd., China. Both PC and DP were purchased from Shanghai Advanced Vehicle Technology Pharmaceutical Co., Ltd.,China. Coumarin-6, 1,1’-dioctadecyl-3,3,3’,3’-tetramethylindotricarbocyanine iodide (DiR), and other fluorescent dyes were purchased from Shanghai Aladdin Bio-Chem Technology Co. Ltd.,China. The other solvents used were of chromatographic grade.
Cell culture and animals
A549 human pulmonary adenocarcinoma cells were purchased from Nanjing KeyGen Biotech Co., Ltd (China) obtained from American Type Culture Collection (ATCC), Manassas, VA and authenticated before receipt. The cells were cultured in Dulbecco’s modified Eagle’s medium with 10% fetal bovine serum in an incubator at 37 °C, containing 5% CO2.
Nude mice weighing 20 ± 2 g (SCXK2013-0018) were obtained from the SLAC Lab Animal Center of Shanghai (Shanghai, China). They were acclimatized for 1–2 weeks with a 12-h day/night cycle prior to examination. They had access to tap water and standard food ad libitum. Animal welfare and experimental procedures were strictly in accordance with the Guide for the Care and Use of Laboratory Animals (US National Research Council, 1996) and the related ethics regulations of our hospital. Animal protocols were reviewed and approved by the Internal Animal Care and Use Committee of Suqian Branch Jiangsu Province Hospital.
Cell viability assay
The cytotoxicity of parthenolide, CK, and liposomes against A549 cells was evaluated by 3–(4,5-dimethylthiazol-2-yl)-2,5-diphenyltetrazolium bromide (MTT) colorimetric assay. Cells were plated in a 96-well culture plate (Corning Incorporated, Corning, NY) and allowed to attach before replacing the medium with drug-containing medium, and incubating for 72 h. The medium was then removed and the MTT solution was added, followed by incubation for 4 h. The purple formazan product was solubilized using dimethyl sulfoxide and the absorbance at 540 nm was determined by a microplate reader (EL-x800; BioTek Instruments, Winooski, VT). Cells were also photographed under an inverted microscope (IX51; Olympus Corp, Tokyo, Japan). Cytotoxicity data were analyzed to determine the 50% inhibitory concentration (IC50). Synergistic effects were evaluated using CompuSyn and Chou-Talalay’s plot, as described previously [Citation28].
Preparation and characterization of liposomes
To obtain tLyp-1-decorated liposomes loaded with parthenolide and CK, a mixture of egg yolk lecithin, cholesterin, 1,2-distearoyl-sn-glycero-3-phosphoethanolamine-N-[amino(polyethylene glycol)-2000] (DSPE-PEG2000), DSPE-PEG2000-tLyp-1, CK, and parthenolide at a weight/weight ratio of 80:10:5:5:22:5 was prepared by direct hydration of a lipid film, as described previously [Citation29]. CK/parthenolide PEG liposomes were prepared using the same method, where DSPE-PEG2000 was used instead of DSPE-PEG2000-tLyp-1.
Similarly, tLyp-1 and PEG liposomes were loaded with a hydrophobic near-infrared cyanine dye, 1,1′-dioctadecyl-3,3,3′,3′-tetramethylindotricarbocyanine iodide (DiR) or coumarin-6 (C-6). These were prepared as described above, by substituting DiR or C-6 for CK/parthenolide, as appropriate.
Liposome size and morphology were determined by dynamic light scattering (BT-90; Bettersize Instruments Ltd., China), transmission electron microscopy (TEM; Tecnai G2 F20; Field Electron and Ion Company, American, Hillsboro, OR), and atomic force microscopy (SPM9700; Shimazduo, Japan).
Solubility measurements
Classical approaches for measuring solubility were based on the saturation shake-flask method. Excess amounts of CK and parthenolide were placed into 5-ml vials containing aqueous solutions. The vials were sealed and placed into a constant temperature bath (37 °C) and shaken for 24 h until equilibrium was evident. After centrifugation of the incubated suspensions at 37 °C and 15,000 rpm for 15 min, the concentrations of CK and parthenolide in the supernatant solutions were determined by a high performance liquid chromatography (HPLC) procedure. Furthermore, the CK and parthenolide concentration in the liposomes system were determined after a 10- to 100-times dilution with methanol, and then analyzed for CK and parthenolide.
Cellular uptake in vitro
The cellular uptake of tLyp-1 liposomes and PEG liposomes by A549 cells was recorded by fluorescence microscopy and confocal laser scanning microscopy. C-6, which exhibited green fluorescence, was chosen as the fluorescent probe. Firstly, A549 cells were seeded into six-well plates at a density of 4 × 105 cells/well and cultured for 12 h. PEG or tLyp-1 liposomes loaded with C-6 at the same concentrations (C-6 concentration: 300 ng/mL) were then added to the culture medium. After incubation at 37 °C for 4 h, the cells were fixed in ethanol and the nuclei were stained using Hoechst 33258 (10 μg/mL). The cells were then photographed under a fluorescent microscope (IX71) and a confocal laser scanning microscope (Zeiss LSM710, Germany).
To evaluate the effects of micropinocytosis and receptor-mediated uptake of tLyp-1 liposomes, A549 cells were pre-incubated with 50 μM amiloride (a micropinocytosis inhibitor) or 5 mg/mL tLyp-1 to inhibit receptor-mediated uptake for 1 h. C-6 tLyp-1 liposomes were then added and incubated for an additional 4 h prior to photographing the cells, as described above.
Endosomal/lysosomal escape studies
To study the endosomal/lysosomal escape behaviors of tLyp-1 liposomes, A549 cells were incubated with C-6 tLyp-1 liposomes or C-6 PEG liposomes (10 μM) for 2, 4, 8, or 12 h at 37 °C. The cells were washed three times with phosphate-buffered saline (PBS), followed by staining for 20 min at 37 °C using LysoTracker Red (25 ng/mL) for endosomes/lysosomes and Hoechst 33258 (10 μg/mL) for nuclei. Cells were then washed three times with PBS. The cellular localization was visualized by confocal laser scanning microscopy (LSM710).
In vivo and ex vivo imaging
Nude mice bearing A549 tumors were utilized to investigate the biodistribution of tLyp-1 liposomes. DiR tLyp-1 liposomes and DiR PEG liposomes were injected into the tumor-bearing nude mice via the tail vein at a dose of 5 mg/kg DiR (n = 3 for each group). After anesthetizing the mice using sevoflurane, the real-time accumulation of liposomes was monitored at 2, 4, 8, 10, and 24 h post-injection. Twenty-four hours later, the mice were immediately euthanized by cervical dislocation. The hearts, livers, spleens, lungs, kidneys, and tumors were collected. Each organ was imaged using an IVIS 200 imaging system (PerkinElmer, Hopkinton, MA).
Analyses of mitochondrial apoptosis
Apoptosis
To investigate the level of apoptosis, cells were incubated with annexin V-fluorescein isothiocyanate conjugate and propidium iodide solution (Nanjing KeyGen Biotech Co., Ltd.). The cells were then analyzed using a FACScan flow cytometer (Beckman Coulter, Brea, CA).
Mitochondrial morphology
Mitochondrial morphology was observed by TEM. Dehydrated samples were embedded in epoxy resin, sectioned, and stained with uranyl acetate and lead citrate prior to examination under the TEM.
Intracellular reactive oxygen species
Intracellular reactive oxygen species (ROS), which are considered to play an important role in mitochondrial apoptosis, were investigated by measuring oxidation of 2′,7′-dichlorodihydrofluorescein diacetate to the fluorescent compound, 2′,7′-dichlorofluorescein. The level of fluorescence in the cells was analyzed using a FACScan flow cytometer.
Mitochondrial membrane potential
Mitochondrial membrane potential (MMP) was measured using a 5,5′,6,6′-tetrachloro-1,1′,3,3′-tetraethyl-imidacarbocyanine (JC-1) MMP Detection Kit (Nanjing KeyGen Biotech Co., Ltd.) and by confocal laser scanning microscopy (LSM710). For the JC-1 flow cytometry assay, treated cells were incubated with JC-1 for 30 min at 37 °C and then washed twice with PBS prior to analysis. Similarly, cells were washed twice with buffer solution without trypsinization prior to confocal laser scanning microscopy, where the shift from red to green fluorescence represented depolarization.
Intracellular free calcium
To analyze mitochondrial matrix calcium (Ca2+), cells were treated with a fluorescent Ca2+-specific probe, Fluo-3 acetoxymethyl ester, for 1 h at 37 °C. This probe is converted to Fluo-3 within cells; Ca2+ binding to Fluo-3 generates a green fluorescent signal. This was observed by confocal laser scanning microscopy.
Cytochrome c
Cells were grown on coverslips and incubated with the test formulations for 72 h, before incubation with an anti-cytochrome c antibody (1:100) for 2 h followed by incubation with a fluor488-conjugated secondary antibody (1:100) for 1 h. Nuclei were stained using 4',6-diamidino-2-phenylindole for 5 min in dark prior to image capture by confocal laser scanning microscopy.
In vivo antitumor efficacy
When the A549 tumors reached a volume of 50 mm3 in the nude mice, the test formulations were administered intravenously every three days (four injections in total). These treatments were: CK (37.5 mg/kg); parthenolide (7.5 mg/kg); a combination of CK (37.5 mg/kg) and parthenolide (7.5 mg/kg); CK/parthenolide PEG liposomes (37.5 mg/kg CK and 7.5 mg/kg parthenolide); CK/parthenolide tLyp-1 liposomes (37.5 mg/kg CK and 7.5 mg/kg parthenolide); saline (negative control); and cisplatin (1 mg/kg; positive control). During the treatment, the tumor sizes and body weights were monitored. Tumor volume was computed as (tumor length) × (tumor width)2/2. After the 15th day, the mice were sacrificed and the hearts, livers, spleens, lungs, kidneys, and tumors were harvested for histological analysis of toxicity using hematoxylin and eosin staining.
Statistical analysis
The results were reported as the mean ± the standard deviation. Student’s t-test or one-way analysis of variance was used to determine statistical significance. A p value of <.05 was considered statistically significant.
Results
In vitro synergistic cytotoxic effects of CK and parthenolide
MTT assays were used to study the effects of CK and parthenolide on the proliferation of the A549 cell line. These cells were exposed to multiple concentrations of either CK or parthenolide alone for 72 h. As shown in both of these compounds produced concentration-dependent inhibition of cellular proliferation. Parthenolide exhibited a greater effect than CK and therefore had a lower IC50 value. Based on these results, we chose 1.5 μg/mL parthenolide as a ‘‘low’’ concentration to be used in combination treatments. shows the effect of 1.5 μg/mL parthenolide combined with various concentrations of CK (1.83, 3.75, 7.5, 15, 30, and 60 μg/mL). The cooperativity index (CI) was 1.309, 1.109, 0.753, 0.813, 1.040, and 1.636 for these treatments, respectively. The CI was calculated using the Chou-Talalay method for the evaluation of the synergism (CI < 1) or antagonism (CI > 1) of each drug combination [Citation30]. The results indicated that the CK:parthenolide ratio of 5:1 produced a synergistic effect (CI = 0.753). The photographs of cells exposed to this 5:1 ratio of CK:parthenolide confirmed this finding, as shown in . Thus, given the synergy and cytotoxicity observed in the A549 cell line, this 5:1 ratio of CK:parthenolide (by weight) was selected for liposome formulation.
Liposome characterization
The dynamic light scattering results indicated that the sizes of CK/parthenolide tLyp-1 liposomes and CK/parthenolide PEG liposomes were 188 and 155 nm, respectively. This indicated that the tLyp-1 coating may alter the liposome size (). TEM and atomic force microscopy revealed the near spherical nature of the liposomes, consistent with the literature (). However, the sizes of the CK/parthenolide tLyp-1 liposomes and CK/parthenolide PEG liposomes determined by TEM and atomic force microscopy were both smaller than the sizes measured by dynamic light scattering; this may be due to the effect of hydration in the dynamic light scattering experiment, which could result in larger sizes than those observed using the dry conditions employed for TEM and atomic force microscopy.
Figure 2. (A) Sizes of the liposome preparations. (B,C) Morphology of the liposome preparations, observed by TEM and atomic force microscopy of the liposome preparations. (D) Uptake and (E) internalization of the liposome preparations by A549 cells.
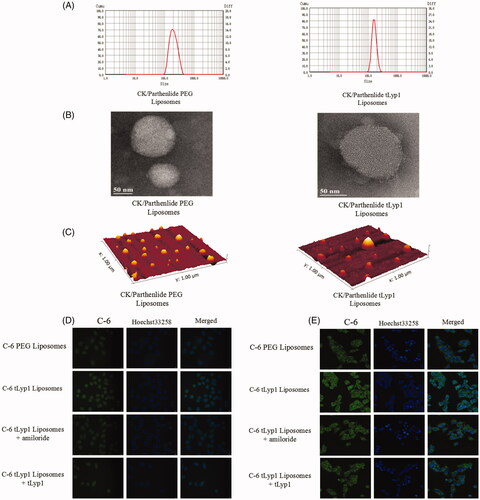
The solubility of CK and parthenolide was increased by their inclusion in tLyp-1 or PEG liposomes. For tLyp-1 liposomes, the solubility of CK and parthenolide increased by 51.4- and 11.7-fold, with entrapment efficiencies of 83.4 and 70.7%, and drug-loading efficiencies of 14.8 and 2.9%, respectively. However, these values were all lower than those observed in PEG liposomes loaded with CK or parthenolide. This may be due to the lower PEG ratio in tLyp-1-coated liposomes, which affected drug solubilization.
Cellular uptake
Liposome uptake and internalization by A549 cells were photographed (). tLyp-1 liposomes appeared to show greater uptake than PEG liposomes. shows that C-6 tLyp-1 liposomes were present in the cytoplasm, rather than in the nucleus.
The mechanisms involved in tLyp-1 liposome internalization by A549 cells were evaluated. The cellular uptake of tLyp-1 liposomes decreased markedly in the presence of amiloride or tLyp-1. This could infer that tLyp-1 liposomes may initially target the tLyp-1 receptor on the surface of cells, followed by internalization via micropinocytosis [Citation31].
Intracellular endosomal/lysosomal escape behavior
After uptake by micropinocytosis, the internalized molecules enter the endosomal/lysosomal system. To investigate whether the liposomes subsequently escaped from this system, we stained C-6 liposome-exposed cells with Hoechst 33258 (blue; nucleus) and Lysotracker (red; endosomal/lysosomal system). As shown in large area of yellow fluorescence was observed in the merged images at 2 and 4 h; this indicated an overlap of red (lysosome) and green (C-6) fluorescence, showing that the PEG and tLyp-1 liposomes were within the endolysosomes. After 12 h, C-6 delivered by PEG liposomes was still stranded in the endolysosomes, as indicated by yellow fluorescence. However, C-6 delivered by tLyp-1 liposomes for incubation periods of ≥8 h showed successful escape from endolysosomes. This study indicated that the tLyp-1 liposomes escaped from lysosomal compartments in a time-dependent manner.
In vivo and ex vivo imaging
To investigate tLyp-1-mediated tumor targeting, in vivo imaging was employed (). The results indicated that PEG liposomes accumulated at the tumor site, probably via the enhanced permeability and retention effect (EPR) effect because PEG is taken up by the reticuloendothelial system. However, stronger fluorescence intensities were observed in the region of the tumor in animals treated with tLyp-1 liposomes.
Following the last image acquisition at 24 h post injection, the mice were sacrificed and the major organs were collected for ex vivo imaging. The fluorescent signals were higher in the liver and spleen than in the other organs examined in animals treated with both liposome types. These analyses also revealed greater accumulation of tLyp-1 liposomes in the tumor, as compared with PEG liposomes. Taken together, the in vivo and ex vivo findings demonstrated that a combination of the EPR effect and tLyp-1-mediated uptake produced a more powerful targeting effect and increased accumulation in the tumor region.
Mitochondrial apoptosis
Apoptosis in A549 cells
As shown in , A549 cells exposed to CK or parthenolide showed low apoptosis rates of 8.2 and 11.8%, respectively. More apoptotic cells were observed following exposure to a combination of CK and parthenolide (p < .05), indicating a synergistic antitumor effect (apoptosis rate was 34.7%). As compared with the combination group, the apoptotic rate was significantly higher (56.7%) in cells exposed to CK/parthenolide tLyp-1 liposomes (p < .05). More encouragingly, exposure to CK/parthenolide tLyp-1 liposomes produced better levels of apoptosis as was observed in cells exposed to cisplatin (p < .05).
ROS-dependent apoptosis
To evaluate whether CK/parthenolide tLyp-1 liposomes induced apoptosis through intracellular reactive oxygen species, we investigated ROS levels. As shown in , treatment with CK and parthenolide for 72 h caused a significant increase in ROS levels (24.6%), as compared with CK (3.7%) or parthenolide (5.8%) alone. Cells exposed to CK/parthenolide tLyp-1 liposomes showed an even greater increase in ROS levels as 28.7% (p < .05). These results were consistent with the apoptosis results described above.
Mitochondrial ultrastructure
We examined changes in mitochondrial morphology by TEM in order to evaluate this apoptotic pathway (). In the control group, the mitochondria appeared to have normal morphology, with no evidence of swelling, outer membrane breaks, or intracristal dilation. In the cells exposed to CK or parthenolide, there were fewer mitochondria and they appeared swollen. In contrast, a marked mitochondrial ultrastructural change was observed after treatment with low doses of combined CK and parthenolide. The most severe mitochondrial ultrastructural change was observed in the group exposed to CK/parthenolide tLyp-1 liposomes. The most marked morphological change was a significant swelling of the mitochondrial matrix.
Mitochondrial membrane potential
Excessive ROS and morphological change often impair the MMP [Citation32]. We therefore analyzed the MMP using JC-1.
Confocal laser scanning microscopy analyses () showed that untreated cells showed homogeneous red fluorescence, with a low level of green fluorescence. After exposure to CK or parthenolide, red fluorescence gradually changed to green fluorescence, indicating a decrease in the MMP. A549 cells treated with a combination of CK and parthenolide exhibited significant green fluorescence, and even more green fluorescence was observed in cells treated with CK/parthenolide tLyp-1 liposomes. This result was consistent with the quantitative flow cytometry data described above and these findings strongly supported our previous observation that CK/parthenolide tLyp-1 liposomes could provide effective delivery of their cargo to A549 cells.
To make a further study, MMP was quantified by flow cytometry. When treated with CK or parthenolide (), the proportion of green fluorescence detected by flow cytometry was 7.6and 6.7%, respectively. The green fluorescence rate was 35.4% in cells exposed to a combination of CK and parthenolide, indicating a significant decrease in MMP (p < .05). Moreover, cells exposed to tLyp-1 liposomes loaded with CK and parthenolide exhibited an even larger change in MMP, which was 37.0% greater than that observed in the combination group (p < .05). And there was no significant difference with cisplatin group.
Location of cytochrome c
The subcellular location of cytochrome c was investigated using confocal microscopy. Upon treatment with CK/parthenolide tLyp-1 liposomes, cytochrome c was released into the cytoplasm and surrounded the nucleus (). This is a typical characteristic of apoptosis and this clustering was not seen in the untreated control cells. Both CK and parthenolide could induce the cytochrome c releasing. When treated with CK and parthenolide, the cytochrome c in cytoplasm was significantly increased. After loaded CK and parthenolide in tLyp-1 liposomes, the distribution of cytochrome c was made a further promotion.
Effects on Ca2+
Although A549 cells treated with CK or parthenolide showed an increase in the Ca2+ level, there was significantly higher in the combination group (). In the MMP study, CK/parthenolide tLyp-1 liposomes produced the largest decrease in the MMP, reflecting an increased permeability of the mitochondrial membrane. Therefore, this may also allow Ca2+ efflux, as indicated by the green fluorescence observed in . Our results showed that tLyp-1 liposomes promoted the uptake of CK and parthenolide, producing a better effect than cisplatin.
Taken together, these findings indicated that CK/parthenolide tLyp-1 liposomes could specifically induce mitochondrial dysfunction in A549 cancer cells, impair ROS generation, decrease the MMP, and cause abnormal perinuclear clustering of cytochrome c and Ca2+.
Wound-healing assay
Meanwhile, the images of the wound healing assay shown in clearly indicated that both CK and parthenolide exerted the significant inhibition of A549 cell migration. The inhibition ratio was 7and 12.2%, respectively. Co-delivery CK and parthenolide in tLyp-1 liposomes may obtain the higher inhibition ratio (47.66%) with no exception of the cisplatin group (p < .05).
In vivo antitumor efficacy
The in vivo antitumor efficacy of each test formulation was evaluated in A549 tumor-bearing mice. As shown in , animals treated with successive intravenous injections of cisplatin or CK/parthenolide showed significant inhibition of tumor growth (p < .05). CK/parthenolide tLyp-1 liposomes produced stronger tumor inhibition than the combination treatment alone, indicating that liposomes functionalized by tLyp-1 showed an improved tumor-targeting capability (p < .05).
Figure 6. In vivo antitumor efficacy in mice with subcutaneous A549 tumors. (A) Tumor volume over time after treatment. (B) Photographs of mice containing subcutaneous A549 tumors at the end of the experiment. (C) Change in mouse body-weight over time. (D) Images of hematoxylin and eosin-stained sections of the indicated tissues.
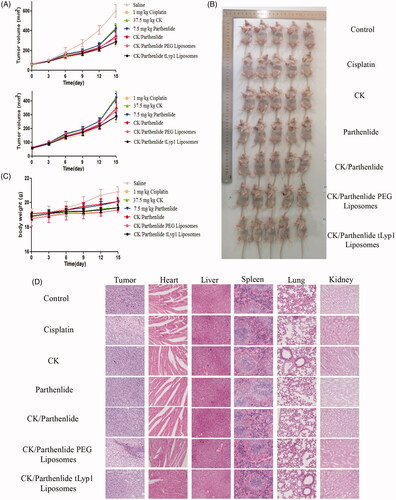
Furthermore, mouse body weights were measured to evaluate treatment-induced toxicity (). Compared to the saline group, although there was significant weight change in the treatment groups, it may because of the tumor volumes increased significantly. With the exception of the saline group, no significant reductions in body weight were observed, indicating the low toxicity of this natural product-based treatment.
As shown in , histological analyses of the mouse tumors using hematoxylin and eosin staining showed the greatest tumor shrinkage in mice treated with CK/parthenolide tLyp-1 liposomes, as compared to that observed in animals treated with other formulations. This provided further in vivo evidence of the superior therapeutic efficacy of this preparation. Microscopic analysis was also used to identify potential adverse effects. A lower toxicity in heart, liver, spleen, lung, and kidney tissues was observed after treatment with CK/parthenolide tLyp-1 liposomes as compared with cisplatin group. These findings suggested that the synergistic effects of CK and parthenolide in tLyp-1 liposomes could effectively inhibit tumor growth and reduce side effects, indicating a promising strategy for antitumor therapy.
Discussion
Natural products have afforded a rich source of compounds that have found many applications in the fields of medicine, pharmacy, and biochemistry. Well-known natural products continue to be employed for cancer treatment. Therapy using edible phytochemicals is now considered to provide an inexpensive, readily applicable, acceptable, and accessible approach to cancer control and management. However, despite the significant advances in natural products, their lower antitumor efficacy, as compared with synthetic compounds, cannot be ignored.
Combinations of traditional Chinese medicines have been widely used to treat various diseases, including cancer [Citation33–36]. The main advantages of this approach are the ability to reduce the dose and toxicity of the individual compounds, and to minimize or delay the induction of drug resistance [Citation30]. Drug combinations can also produce synergistic therapeutic effects.
In the present study, we evaluated the combined effects of CK and parthenolide as antitumor agents and found that they acted synergistically, reducing the effective dose of each compound. However, in clinical practice, while drug combination therapy via sequential administration is recommended in standard cancer chemotherapy guidelines, simultaneous administration is not [Citation37]. This is because simultaneous administration of combination therapy is difficult. To achieved regulatory approval and be clinically beneficial for patients, combining medicines in liposomes could mitigate these problems and further enhance the application of natural product combination therapies.
The use of liposomes carrying natural drug combinations could maximize the synergistic antitumor effects and minimize the systemic side-effects of treatment by overcoming multiple biological barriers and facilitating specific delivery to the target sites at tumor cells. All the components of the tLyp-1 liposomes employed in the present study are endogenous and some have been approved for clinical use in humans. This approach provides controlled temporal and spatial delivery of multiple compatible medicines simultaneously, thus coordinating drug synergy against cancer cells. The tLyP-1 peptide ligand was chosen to actively recognize tumor cells overexpressing neuropilin receptors, as well as to enable deep and uniform tumor tissue penetration in order to reach more cancer cells [Citation38]. The hydrophilic PEG shell of the tLyP-1 liposomes helps to improve their serum stability and to avoid rapid non-specific elimination by the reticular endothelial system, thus prolonging their blood circulation time. In addition, this delivery system also exhibits many other advantages, such as an increase in drug solubilization, cellular uptake via endocytosis, endosomal escape, and destabilization of cancer cell mitochondria to induce apoptosis. It is also noteworthy that this study identified tumor selectivity, which plays a key role in reducing the side effects on normal tissues. This is particularly important and warrants consideration in the further development of new strategies for efficient cancer treatment using combinations of low-toxicity natural products.
Ethical approval
This study was performed with the “Guide for the protection and use of experimental animals” and was approved by the Committee of Ethics of Armed Police Logistics College.
Conclusion
We showed that parthenolide and CK exhibited synergistic effects. Loading compounds into tLyp-1 liposomes increased tumor targeting. Therefore, combined application of parthenolide and CK in actively targeted tLyp-1 liposomes produced an increased antitumor effect, with minimal adverse effects. The findings of this study indicate that further investigations of natural antitumor products in actively targeted carriers are warranted.
Data availability
All data generated or analyzed during this study are included in the article.
Disclosure statement
No potential conflict of interest was reported by the authors.
Additional information
Funding
References
- Mu LM, Ju RJ, Liu R, et al. Dual-functional drug liposomes in treatment of resistant cancers. Adv Drug Deliv Rev. 2017;115:46–56.
- Barenholz Y. Doxil®-the first FDA-approved nano-drug: lessons learned. J Control Release. 2012;160:117–134.
- Rodriguez MA, Pytlik R, Kozak T, et al. Vincristine sulfate liposomes injection (Marqibo) in heavily pretreated patients with refractory aggressive non-Hodgkin lymphoma: report of the pivotal phase 2 study. Cancer. 2009;115:3475–3482.
- Coleman RE, Biganzoli L, Canney P, et al. A randomised phase II study of two different schedules of pegylated liposomal doxorubicin in metastatic breast cancer (EORTC-10993). Eur J Cancer. 2006;42:882–887.
- Perez-Soler R, Shin DM, Siddik ZH, et al. Phase I clinical and pharmacological study of liposome-entrapped NDDP administered intrapleurally in patients with malignant pleural effusions. Clin Cancer Res. 1997;3:373–379.
- Hartwell JL. Plants used against cancer: a survey. Lawrence, MA: Quarterman; 1982.
- Tan G, Gyllenhaal C, Soejarto DD. Biodiversity as a source of anticancer drugs. Curr Drug Targets. 2006;7:265–277.
- Ghantous A, Sinjab A, Herceg Z, et al. Parthenolide: from plant shoots to cancer roots. Drug Discov Today. 2013;18:894–905.
- Yu HJ, Jung JY, Jeong JH, et al. Induction of apoptosis by parthenolide in human oral cancer cell lines and tumor xenografts. Oral Oncol. 2015;51:602–609.
- Liu W, Wang X, Sun J, et al. Parthenolide suppresses pancreatic cell growth by autophagy-mediated apoptosis. Onco Targets Ther. 2017;10:453–461.
- Yang C, Yang QO, Kong QJ, et al. Parthenolide induces reactive oxygen species-mediated autophagic cell death in human osteosarcoma cells. Cell Physiol Biochem. 2016;40:146–154.
- Zahedpanah M, Shaiegan M, Ghaffari SH, et al. Parthenolide induces apoptosis in committed progenitor AML cell line U937 via reduction in osteopontin. Rep Biochem Mol Biol. 2016;4:82–88.
- Carlisi D, Lauricella M, D'Anneo A, et al. The synergistic effect of SAHA and parthenolide in MDA-MB231 breast cancer cells. J Cell Physiol. 2015;230:1276–1289.
- Duan D, Zhang J, Yao J, et al. Targeting thioredoxin reductase by parthenolide contributes to inducing apoptosis of HeLa cells. J Biol Chem. 2016;291:10021–10031.
- Liu YC, Kim SL, Park YR, et al. Parthenolide promotes apoptotic cell death and inhibits the migration and invasion of SW620 cells. Intest Res. 2017;15:174–181.
- Lin M, Bi H, Yan Y, et al. Parthenolide suppresses non-small cell lung cancer GLC-82 cells growth via B-Raf/MAPK/Erk pathway. Oncotarget. 2017;8:23436–23447.
- Kim SL, Kim SH, Park YR, et al. Combined parthenolide and balsalazide have enhanced antitumor efficacy through blockade of NF-κB activation. Mol Cancer Res. 2017;15:141–151.
- Jeyamohan S, Moorthy RK, Kannan MK, et al. Parthenolide induces apoptosis and autophagy through the suppression of PI3K/Akt signaling pathway in cervical cancer. Biotechnol Lett. 2016;38:1251–1260.
- Kim YJ, Zhang D, Yang DC. Biosynthesis and biotechnological production of ginsenosides. Biotechnol Adv. 2015;33:717–735.
- Kwak CW, Son YM, Gu MJ, et al. A bacterial metabolite, compound K, induces programmed necrosis in MCF-7 cells via GSK3β. J Microbiol Biotechnol. 2015;25:1170–1176.
- Shin KO, Seo CH, Cho HH, et al. Ginsenoside compound K inhibits angiogenesis via regulation of sphingosine kinase-1 in human umbilical vein endothelial cells. Arch Pharm Res. 2014;37:1183–1192.
- Kim AD, Kang KA, Kim HS, et al. A ginseng metabolite, compound K, induces autophagy and apoptosis via generation of reactive oxygen species and activation of JNK in human colon cancer cells. Cell Death Dis. 2013;4:e750.
- Zhang K, Li Y. Effects of ginsenoside compound K combined with cisplatin on the proliferation, apoptosis and epithelial mesenchymal transition in MCF-7 cells of human breast cancer. Pharm Biol. 2016;54:561–568.
- Igami K, Ozawa M, Inoue S, et al. The formation of an inclusion complex between a metabolite of ginsenoside, compound K and γ-cyclodextrin and its dissolution characteristics. J Pharm Pharmacol. 2016;68:646–654.
- Zhao AQ, Zhao JH, Zhang SQ, et al. Determination of parthenolide in rat plasma by UPLC-MS/MS and its application to a pharmacokinetic study. J Pharm Biomed Anal. 2016;119:99–103.
- Wang W, Li M, Zhang Z, et al. Design, synthesis and evaluation of multi-functional tLyP-1-hyaluronic acid-paclitaxel conjugate endowed with broad anticancer scope. Carbohydr Polym. 2017;156:97–107.
- Jiang D, Gao X, Kang T, et al. Actively targeting D-α-tocopheryl polyethylene glycol 1000 succinate-poly(lactic acid) nanoparticles as vesicles for chemo-photodynamic combination therapy of doxorubicin-resistant breast cancer. Nanoscale. 2016;8:3100–3118.
- Jin X, Yang Q, Zhang Y. Synergistic apoptotic effects of apigenin TPGS liposomes and tyroservatide: implications for effective treatment of lung cancer. Int J Nanomed. 2017;12:5109–5118.
- Jin X, Li M, Yin L, et al. Tyroservatide-TPGS-paclitaxel liposomes: tyroservatide as a targeting ligand for improving breast cancer treatment. Nanomedicine. 2017;13:1105–1115.
- Chou TC. Theoretical basis, experimental design, and computerized simulation of synergism and antagonism in drug combination studies. Pharmacol Rev. 2006;58:621–681.
- Khalil IA, Kogure K, Futaki S, et al. High density of octaarginine stimulates macropinocytosis leading to efficient intracellular trafficking for gene expression. J Biol Chem. 2006;281:3544e51.
- Wu H, Liu S, Gong J, et al. A novel synthetic derivative of α-tocopheryl succinate, sensitizes human gastric cancer to doxorubicin-induced apoptosis via ROS-dependent mitochondrial dysfunction. Cancer Lett. 2017;393:22–32.
- Ulrich-Merzenich GS. Combination screening of synthetic drugs and plant derived natural product potential and challenges for drug development. Synergy. 2014;1:59–69.
- Rastegar R, Akbari Javar H, Khoobi M, et al. Evaluation of a novel biocompatible magnetic nanomedicine based on beta-cyclodextrin, loaded doxorubicin-curcumin for overcoming chemoresistance in breast cancer. Artif Cells Nanomed Biotechnol. 2018;24:1–10.
- Pashaei-Asl F, Pashaei-Asl R, Khodadadi K, et al. Enhancement of anticancer activity by silibinin and paclitaxel combination on the ovarian cancer. Artif Cells Nanomed Biotechnol. 2018;46:1483–1487.
- Hemati M, Haghiralsadat F, Yazdian F, et al. Development and characterization of a novel cationic PEGylated niosome-encapsulated forms of doxorubicin, quercetin and siRNA for the treatment of cancer by using combination therapy. Artif Cells Nanomed Biotechnol. 2018;22:1–17.
- Cardoso F, Bedard PL, Winer EP, et al. International guidelines for management of metastatic breast cancer: combination vs. sequential single-agent chemotherapy. J Natl Cancer Inst. 2009;101:1174–1181.
- Teesalu T, Sugahara KN, Kotamraju VR, et al. C-end rule peptides mediate neuropilin-1-dependent cell, vascular, and tissue penetration. Proc Natl Acad Sci USA. 2009;106:16157–16162.