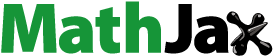
Abstract
The coprecipitation–cross-linking–dissolution (CCD) technique for protein submicron particle fabrication was improved by omitting one preparation step using the macromolecular cross-linker, periodate-oxidized dextran (Odex, M.W. of 40 and 70 kDa). The coprecipitation and cross-linking of haemoglobin (Hb) were combined in one single step since the cross-linker is incorporated into the inorganic template, MnCO3, together with the protein. After removal of the MnCO3 templates by EDTA, the amount of entrapped Hb was 60 to 70% of the initial amount. This technique provides deformable Hb submicron particles (HbMP) with narrow size distribution between 800 and 1000 nm, uniform morphology and negative zeta-potential. More than 40% of Hb in the particles was able to carry oxygen over a storage period of 90 days. The results suggest that our new protein submicron particle fabrication technique minimizes the fabrication time and is very efficient and cost-effective.
Introduction
In the last decades, protein particles have been proposed for a number of biomedical applications such as drug delivery systems and haemoglobin (Hb)-based oxygen carriers [Citation1]. Many techniques are available for the fabrication of protein nano- and microparticles including spray drying, emulsification, layer-by-layer assembly, solvent displacement and polymersome formation [Citation2]. However, each of these techniques has serious drawbacks which impair its function and/or biocompatibility. For example, spray drying or lyophilization techniques deliver protein particles in dry powder formulation but their polydispersity is usually high [Citation3,Citation4] and entrapment efficiency is typically poor in protein particles prepared by solvent displacement [Citation5]. Apart from that, tertiary and quaternary structures of proteins need to be preserved to maintain protein function that is hard to fulfil by many of these conventional methods due to exposure to high temperature, high ionic strength, non-physiological pH, organic solvents or hydrophobic interfaces.
Previously, we described a micro- and sub-microparticle fabrication method called coprecipitation–cross-linking–dissolution (CCD), which consists of three main steps. First, protein and inorganic template are precipitated together followed by a cross-linking step where glutaraldehyde (GA) was used to cross-link the proteins. Finally, EDTA was used to remove the inorganic template to obtain protein microparticles [Citation6–8]. This technique is favourable to fabricate in mild condition and provides pure protein particles with uniform morphology and narrow size distribution. However, the cross-linking of protein with GA needs to be taken into consideration.
The GA can react with several functional groups of proteins, such as amine, thiol, phenol and imidazole, and is very effective as a protein cross-linker because the most reactive amino acid side chains are nucleophiles [Citation9]. It, therefore, has been widely used to cross-link proteins and other biopolymers for medical applications. However, there are many reports concerning the undesired properties of GA-cross-linked biomaterials such as autofluorescence as well as its toxicity [Citation10,Citation11]. For that reason, “biocompatible” cross-linkers are now being investigated as alternatives for GA to reduce the risks of side effects.
Polysaccharides are natural biopolymers which have a great number of reactive groups on their molecular chains, such as hydroxyl, carboxyl and amino groups, which can make them be easily modified chemically and biochemically. They are highly safe, non-toxic, stable, biodegradable and biocompatible. Besides, polysaccharides have abundant resources in nature and low cost in their processing. There are various sources from animal (chitosan, chondroitin), plant (e.g. pectin, cellulose), alga (e.g. alginate) and microorganisms (e.g. dextran). The polysaccharide is desirably oxidized to extent sufficient to provide the aldehyde groups capable of promoting rapid cross-linking of the polymers. For that reason, there are several usages of polysaccharide in the biomedical application as a biopolymer cross-linker; for instance, oxidized hydroxyethyl starch [Citation12], oxidized raffinose (O-raffinose) [Citation13–15] and oxidized dextran (Odex) [Citation16,Citation17] were used as a cross-linker for Hb conjugation.
Dextran is a glucose homopolymer, produced by the bacteria called Leuconostoc mesenteroides. It is consisting essentially of α-1,6 linked D-glucopyranose residues with a few per cent of α-1,2-, α-1,3- or α-1,4-linked side chains. Dextran is selected in many biomedical applications because it is slowly degraded by human enzymes compared to other polysaccharides (e.g. glycogen with α-1,4 linkages) and cleaved by microbial dextranases in the gastrointestinal tract. It has been previously used as plasma expander and was investigated as a macromolecular carrier for the delivery of drugs and proteins, to increase the durability of therapeutic agents in the blood circulation and to decrease the in vivo immunogenicity of proteins and enzymes [Citation18,Citation19]. It is also an excellent candidate as a bio-cross-linker because of its chemical properties such as high water solubility, high stability, high content of hydroxyl groups, which are suitable for derivatization and can be subsequently used for chemical cross-linking of proteins [Citation20]. Oxidation of dextran by periodate ions is a common dextran functionalizing modification reaction which yields a purified product with simple dialysis method. Glucose residue of dextran has vicinal diol which houses two oxidizable bonds. The oxidation of those bonds forms an aldehyde in C3, which is susceptible to periodate oxidation on account of the presence of the hydroxyl groups in C2 and C4 and subsequently leads to a double oxidation of the same residue. This leads to the opening of the glucose ring and formation of double aldehyde groups, which may react with amino groups creating Schiff’s base (Maillard reaction). The dextran oxidation reaction may perform under mild conditions with high efficiency but no catalyst is needed [Citation21]. For this reason, oxidized dextran (Odex) has recently been investigated as an “alternative biocompatible hydrophilic cross-linker” for biopolymers, that is hydrogel formation, tissue engineering and drug delivering particles [Citation22–24]. Nevertheless, to the best of our knowledge, Odex was not used for the fabrication of micro- or nano-protein particles.
Therefore, the aim of this study was to develop a novel biopolymer microparticle fabrication method based on the CCD technique by applying Odex as a cross-linker for Hb. Here, the coprecipitation and the cross-linking steps are combined in one single step for the reason that the cross-linker, Odex, is coprecipitated together with the protein. The physiochemical properties of the resulting Odex-cross-linked Hb particles (Odex-HbMP) were investigated by several techniques including scanning electron microscopy (SEM), atomic force microscopy (AFM) and dynamic light scattering, zeta-potential measurements, spectrophotometry. Additionally, the functionality of the Hb entrapped in the Odex-HbMP as well as the stability of Odex-HbMP in a salt solution and their deformability was determined.
Materials and methods
Materials
Dextran (from Leuconostoc mesenteroides, M.W. 40, 70 kDa) was purchased from AppliChem GmbH (Darmstadt, Germany). Human serum albumin (HSA) solution was purchased from Grifols GmbH (Frankfurt, Germany). Ethylenediaminetetraacetic acid (EDTA), potassium ferricyanide (K3[Fe(CN)6]), glycine, manganese chloride, sodium borohydride, sodium carbonate, sodium chloride, sodium dithionite, sodium hydroxide and sodium (meta) periodate were purchased from Sigma-Aldrich (Darmstadt, Germany).
Preparation of haemoglobin
Haemoglobin was extracted from bovine red blood cells by hypotonic haemolysis as previously described [Citation25,Citation26]. Briefly, fresh bovine whole blood (EDTA-anticoagulated, obtained from Biophyll GmbH, Dietersburg, Germany) was centrifuged at 2500 g for 15 min at 4 °C. The resulting packed red blood cells were washed three times with 0.9% NaCl (B. Braun Melsungen AG, Melsungen, Germany). Haemolysis was performed by adding five volumes of lysing NaCl solution (100 mOsmol/kg). The solution was stirred at 4 °C overnight and then centrifuged for 3 h at 5500 g. The supernatant was filtered by means of tangential flow filtration (TFF) using the KrosFlo Research II TFF system (Spectrum Europe B.V., the Netherlands) similar to the process described earlier (23). One 500 kDa mPES hollow fibre filter module (MiniKros Sampler, Spectrum) was used with a permeate flow rate of 30 to 25 ml/min and a transmembrane pressure (TMP) of 0.3 to 0.5 bar. The filtered Hb was stored as a stock solution at −30 °C until use.
Synthesis and characterization of oxidized dextran (Odex)
Sodium periodate (6.6 g, 30.9 mmoL) was added into 50 ml of 10% of dextran (30.9 mmoL glucose subunits) in water, shielded from light, with constant stirring at room temperature. The reaction was allowed to proceed for 1 h [Citation27]. Then, the reaction mixture containing the oxidized dextran (Odex) was transferred to a cellulose dialysis tube (MWCO of 12,000: Carl Roth GmbH, Karlsruhe, Germany) and dialyzed against water. Finally, the Odex solution was lyophilized by the freeze-drying method and the obtained dried powder was maintained at room temperature for further usage [Citation28].
The aldehyde group content of Odex was determined using the hydroxylamine hydrochloride titration method, and non-oxidized dextran samples were used as a control [Citation23,Citation28]. Briefly, 0.1 g of lyophilized oxidized dextran was dissolved in 25 ml of 0.25 N hydroxylamine hydrochloride-methyl orange solution pH 4.0. The mixtures were then titrated with standardized 0.1 N NaOH solution until the red-to-yellow endpoint was achieved by the equivalent of the colour of the non-oxidized dextran titrated solution. Thereby, the number of aldehyde groups per 100 glucose subunits was calculated with the following formula:
(1)
(1)
where N is the concentration of standardized sodium hydroxide, V is the volume of NaOH solution used in the titration, MW is the molecular weight of dextran, wt is the weight of dextran and S is the number of glucose subunits in dextran.
Preparation of Odex-HbMP
Haemoglobin particles (HbMP) were fabricated by a modified protocol based on the CCD technique as previously described by Bäumler et al. [Citation6–8,Citation29,Citation30]. In brief, equal volumes of solution 1 consisting of 0.25 M of MnCl2 and 10 to 50 mg/mL of Hb were rapidly mixed with solution 2 containing 0.25 M of Na2CO3 and 5 to 40 mg/mL of Odex in a beaker under vigorous stirring using a mechanical stirrer (model IKA RW 20 DZM equipped with a 35-mm-diameter rotor) at room temperature. In this step, the Hb and the cross-linker Odex were precipitated together into the inorganic MnCO3 allowing the cross-linking reaction to perform in the formulated submicron particles. After incubation for 30 s, 5 mg/mL of HSA was added to the suspension and incubated for 5 min under stirring as a result of the adsorption of HSA on the surface of particles. After HSA incubation, the resulting suspension particles were proceeded to the dissolution of the MnCO3 template by 0.25 M EDTA/0.05 M glycine for 30 min and the reduction by 0.4 mg/mL of NaBH4. Finally, the obtained Odex-cross-linked Hb particles (Odex-HbMP) were centrifuged, washed three times (6000 g for 5 min) and suspended in sterile 0.9% NaCl.
The amount of entrapped protein was determined as the difference between the total amount of applied protein (Pt) and the remaining amount of protein in the supernatant (Pf) after coprecipitation and washing steps. Subsequently, the entrapment efficiency (EE%) was calculated according to the following equation:
(2)
(2)
The Hb concentration in the Odex-HbMP was determined by alkaline haematin detergent (AHD)-575 method. The optical density of haematin was measured at 575 nm.
Particles characterization
Scanning electron microscopy
For SEM imaging, samples were prepared by applying a drop of particles suspension onto glass slide followed by drying overnight. After sputtering with gold, measurements were conducted at an operation voltage of 3 keV using Gemini Leo 1550 instrument (Oberkochen, Germany) and ImageJ 1.44p software (Wayne Rasband, National Institute of Mental Health, Bethesda, MD).
Cryo-SEM
The Odex-HbMP suspension was frozen in a copper sandwich holder by submersion in liquid propane at −180 °C in a Cryoplunge (Gatan, USA) and subsequently freeze-fractured (Alto 2500 cryo preparation chamber) at −180 °C, freeze-etched at −98 °C for 54 s and finally sputtered with platinum at −130 °C. After this procedure, the sample was transferred into an ultrahigh-resolution field-emission scanning electron microscopy (SEM) system (Hitachi, S-4800). The SEM micrographs were obtained at a stage temperature of −130 °C and an accelerating voltage of 5 kV.
Particle size and zeta-potential
The hydrodynamic diameter (Z-average diameter) and the zeta-potential of HbMP were measured by dynamic light scattering using a Zetasizer Nano ZS instrument (Malvern Instruments Ltd., Malvern, UK) in PBS solution.
Haemoglobin content in Odex-HbMP
For Hb determination, modified AHD-575 method was employed. 2% (v/v) Odex-HbMP suspension was mixed with pronase to achieve a final concentration of 0.5 mg/ml pronase (Roche Diagnostics GmbH, Mannheim, Germany). The particles were digested by pronase with the purpose to terminate the binding of Odex and Hb in order to reduce the noise scattering from of particles. The pronase–HbMP suspension was then incubated for 30 min at 45 °C. Afterwards, AHD reagent was added at the volume ratio of 1:1 to the pronase–HbMP mixture for hemin conversion. Absorption spectra of the particles were recorded using a UV-VIS spectrophotometer at 575 nm (Hitachi U2800, Hitachi High-Technologies Corporation).
Determination of oxygen carrying capacity of Odex-HbMP
The Hb solution was diluted into different concentrations (0.75–6 mg/mL). 1 ml of each concentration was filled into a 2-ml glass vial containing a magnetic stirrer. After an equilibration time of 5 min, a miniaturized optical needle type oxygen sensor (oxygen microsensor NTH-PSt7, PreSens – Precision Sensing GmbH, Regensburg, Germany), which was connected to a portable oxygen meter with data logging (Microx 4, PreSens – Precision Sensing GmbH, Germany), was inserted into the stirred sample. The concentration of dissolved oxygen was recorded at the starting point. Then, 50 µL of 10% potassium ferricyanide (K3[Fe(CN)6]) dissolved in water was added, and the change in concentration of dissolved oxygen was measured and recorded every second. After reaching a stable value, the measurement was stopped. An increase of dissolved oxygen concentration after adding ferricyanide is dependent on the Hb concentration manner. The change in pO2 is determined by subtracting the pO2 from the beginning and the end of the measurement. The released oxygen was previously bound to Hb and defined as a functional Hb. A calibration curve demonstrated the ratio of Hb concentration versus pO2. For Odex-HbMP, the particle volume concentration was measured by the haematocrit (Hettich Mikro 22R; at 20,000 g for 10 min) and then adjusted to 2%. The measurement of 2% of Odex-HbMP was performed by using the same procedure as described for standard Hb. The functional Hb in the particles compared with a determined calibration curve [Citation31,Citation32]. Finally, the percentage of functional Hb to total Hb was calculated using the identical sample.
Particles deformability
Particle compression and dilation behaviour were measured by using an automatic measuring system based on an analytic centrifuge with an integrated optoelectronic sensor (LUMiFuge, LUM GmbH, Berlin, Germany). The integrated transmitted light intensity during the centrifugation at different accelerations allows quantification of the suspension stability by means of an instability index:
(3)
(3)
where clarification is the integral of transmission along the cuvette during the centrifugation. The maximal possible clarification is obtained at the highest possible centrifugation force until no changes in the profile will be detected.
In total, 10% haematocrit of particles was placed into the cuvette. The centrifugal force was applied to particles as a cycle to observe the compression and relaxation of particles [Citation33]. Particles were first equilibrated at the beginning with a centrifugal force of 5 g for 10 min. Then, the centrifugal force up to 2300 g was applied to compress particles for 4 h. Finally, the centrifugal force was reduced to 5 g again for 4 h to observe the relaxation of particles. The profiles are simultaneously registered along the cuvette by the STEP technology as a function of time and of the applied centrifugal force and analyzed using the software SEPVIEW 6.
Atomic force microscopy
Atomic force microscopy images were obtained using a Nanoscope III Multimode AFM (Digital Instrument Inc., Santa Barbara, CA) in taping mode as described earlier [Citation34]. Briefly, Odex-HbMP were applied into polyethyleneimine (25 kDa, 1 mM)-pretreated mica substrate. After allowing the particles to attach, the substrate was rinsed with deionized water and dried under a gentle stream of nitrogen. The particles were first scanned in the dry state, then a drop of deionized water was added, and the particles were scanned again in the wet state. The height of each particle in the dry and wet state was measured applying the Nanoscope software as the height difference between the support and the highest point of the particle surface profile.
Storage stability of Odex-HbMP
The storage stability of Odex-HbMP in 0.9% NaCl was determined at day 0, 30 and 90 after fabrication. The individual aliquots were kept at 4 °C. Then, the size distribution, the Hb content and the amount of functional Hb of the stored Odex-HbMP were evaluated.
Statistical analysis
The results were presented as means ± standard deviation (SD). The data were compared using ANOVA-like test. GraphPad Prism 6 software (GraphPad, La Jolla, CA) was employed for graphs and statistical analyses. p-Values < .05 were considered statistically significant.
Results
Synthesis of oxidized dextran and characterization
Oxidized dextran was successfully prepared using periodate oxidation reaction. The actual number of aldehyde groups of periodate-oxidized dextran was determined by hydroxylamine hydrochloride titration method. The results showed that aldehydes amount was constant at approximately 157 ± 9 and 152 ± 6 aldehyde groups per 100 glucose subunits for oxidized dextran MW of 40,000 (40T) and dextran MW 70,000 (70T), respectively.
Preparation of Odex-HbMP
Bovine Hb was selected to demonstrate this novel fabrication procedure as shown in . At constant protein concentration of 0.5%, the concentration of 40T- and 70T-Odex during coprecipitation was optimized from 0.25, 0.5, 1.0 and 2.0%, respectively. At low concentrations of Odex, 0.25 and 0.5%, particles were not obtained. The final volume concentration of the Odex-HbMP suspension obtained with 1.0% Odex was lower than that of the Odex-HbMP suspension prepared with 2.0% Odex. Therefore, Odex concentration of 2% was considered as optimal for the fabrication of Odex-HbMP. Odex-HbMP fabricated with 40T-Odex and 70T-Odex are named 40T-HbMP and 70T-HbMP, respectively.
Figure 1. Scheme of particle preparation through the one-pot fabrication technique. Two mixtures of inorganic solutions, MnCl2 containing haemoglobin and Na2CO3 with Odex, are mixed together. Here, MnCO3 particles are formed with entrapped biopolymers; meanwhile, the Hb is cross-linked by Odex in the particle. HSA was absorbed into particle surface. The MnCO3 template is then dissolved and the pure biopolymer particles remain in the solution.
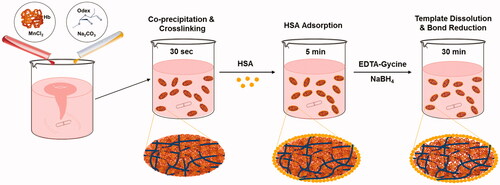
shows the Hb entrapment efficiency of our technique using different initial Hb concentrations and 2% Odex for cross-linking. It can be seen that the entrapped protein remains constant with increasing initial Hb concentration. Hence, the initial Hb concentration of 5 mg/mL the maximal entrapment capacity of the MnCO3 template is reached (). Therefore, Odex-HbMP prepared with 5 mg/mL and 2% Odex were used for all further experiments.
Figure 2. (A) Hb entrapment efficiency of MnCO3 template. (B) The absolute amount of entrapped protein by different initial Hb concentration. Hb content of Hb-MnCO3 was independent of the initial protein amount and it was not able to increase by increasing the initial Hb concentration. Data are presented as mean ± SD (n = 5).
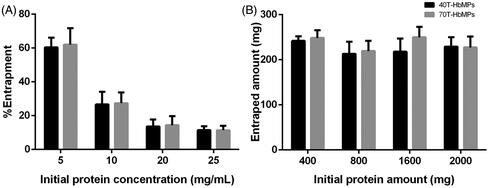
Particles characterization
Morphology, particles size and zeta-potential
The scanning electron micrographs of the Odex-HbMP prepared from 2% Odex and 0.5% Hb in show clearly a peanut-like shape with shortest and longest diameter of about 700 and 1000 nm. Additionally, cryo-SEM micrographs demonstrated that their surface in aqueous environment appears smooth.
Figure 3. SEM images of (A,B) 40T-HbMP and (D,E) 70T-HbMP show a peanut-like shape. Cryo-SEM images of (C) 40T-HbMP and (F) 70T-HbMP show a smooth surface of the particles.
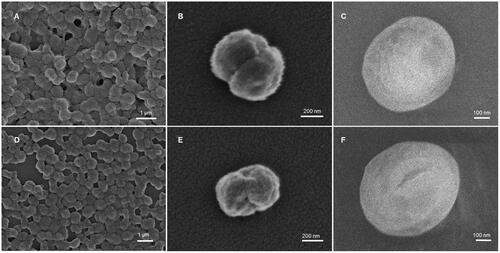
The size of the Odex-HbMP measured by dynamic light scattering was around 800–1000 nm () which corresponds to the observations by SEM. Additionally, Odex-HbMP suspend in PBS (conductivity 18–20 mS/cm) showed a negative zeta-potential () of approximately −9 mV. No significant differences in the physicochemical properties of Odex-HbMP cross-linked by 40T- or 70T-Odex could be found.
Deformability of Odex-HbMP
The deformability of the Odex-HbMP cross-linked with both 40T- and 70T-Odex was studied using an analytical centrifuge with integrated optoelectric sensor system. If the particles are exposed to the high centrifugal force at 2300 g, rapidly packing is obtained which results in a constant thickness of the sediment layer. This becomes visible in the high instability index (). After exposure to a low centrifugal force at 5 g, the particles are decompressed, which increases the thickness of the sediment layer and results in a decreased instability index. This phenomenon demonstrates the deformability of these particles.
Figure 5. Deformability of Odex-cross-linked particles. (A) Compression and dilation behaviour of particles, the profile from the analytical centrifuge. (B) AFM images of 40T-HbMP in dry as well as wet state. (C) The width of the particles is defined as the horizontal distance and (D) the thickness of the particles is given as the vertical distance between the mica support and the top of the particle. Dried HbMP swelled after water was added. Data are presented as mean ± SD (n = 5), p < .05.
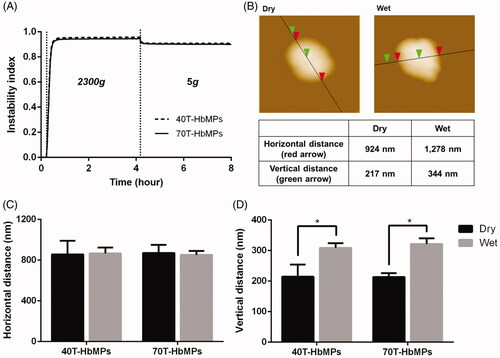
To confirm the deformability of the Odex-HbMP, AFM scans in the dry and wet state were performed. Example of these scans is shown in . represents the measured size of the particles at the long axis and present their thickness measured as the vertical distance between the mica support and the highest point of the height profiles. The mean thickness determined from scans of particles in the dry state of 40T- and 70T-HbMP was 214 ± 40 and 213 ± 12 nm and in the wet state 308 ± 15 and 321 ± 18 nm, respectively. It can be clearly seen that the particles are swollen in the wet state.
Haemoglobin content and oxygen carrying capacity of Odex-HbMP
The concentration of total Hb entrapped in Odex-HbMP was 28–30 mg/mL as calculated for a suspension with a Hct of 20%. The values for 40T-HbMP and 70T-HbMP did not significantly differ from each other (28.8 ± 2.2 and 30.4 ± 2.1, respectively). The amount of functional Hb was 11.59 ± 0.6 and 12.53 ± 0.9 mg/mL, respectively
The ability of oxygenation and deoxygenation of the Odex-HbMP was confirmed using UV-VIS spectrophotometric analysis as shown in . Odex-HbMP have similar spectra and spectral changes compared to free Hb in the oxygenated and deoxygenated state.
Stability of Odex-HbMP
The stability of Odex-HbMP was characterized by their size, the Hb content and the fraction of functional Hb as shown in . It is demonstrated that there was no obvious change in size and in the amount of functional Hb in saline solution at 4 °C during the period of 90 days.
Discussion
Our new one-pot fabrication technique for protein microparticles is based on the CCD procedure, which exploits the ability of insoluble carbonates template to capture proteins and other macromolecules that are present in the aqueous solution during precipitation [Citation30].
The proteins are precipitated and cross-linked in “one step” because the macromolecular cross-linker (Odex) precipitates and is entrapped in the particles together with the proteins (). Odex is promising as a highly effective macromolecular cross-linker because the carbonyl groups of the sugar open chains are capable of reacting with the free –NH2 groups on the protein; the relatively fast formation of Schiff base results in creating a cross-linked network [Citation24,Citation27,Citation35,Citation36].
In such a way, one preparation step and several respective washing steps are omitted, which significantly reduces the preparation time. MnCO3 and bovine Hb were selected as a model to demonstrate this novel fabrication procedure. Haemoglobin content in Odex-HbMP was estimated through photometric and quantitative measurement by a photometric method using the modified pronase-AHD method. The method is the combination of pronase enzyme and AHD-575 together. In comparison with HbMP-700 [Citation6,Citation29] prepared by CCD technique using GA as a cross-linker, Odex-HbMP contain a comparable amount of Hb. The entrapped Hb amount could not be enhanced by increasing the initial concentration of the protein. Additionally, the entrapped protein by MnCO3 using a one-pot procedure had no significant difference in the entrapment efficiency of the CCD procedure as earlier reported by Xiong et al. [Citation7,Citation29]. In comparison with other fabrication methods, the new one-pot procedure provides easily prepared particles with high protein content under ambient condition and no organic solvents or catalysts are needed. The reagents are neither toxic and nor expensive. Henceforward, our new technique is fast, simple, efficient and cost-effective.
The Odex-protein cross-linking process occurs simultaneously during the precipitation. This is achieved by the large molecular size of Odex and its substantial excess in the reaction. Odex can rapidly cover the protein surface avoiding protein–protein interaction () [Citation37]. The Odex concentration during coprecipitation influences the stability of Hb. At the Odex concentration of 2%, HbMP exhibits a higher amount of functional Hb. The reason could be that the dextran moiety hinders the steric expansion of Hb that would inhibit the denaturation rate of Hb. Additionally, Zhang et al. reported earlier that dextran was able to decrease the autoxidation rate of Hb and could structurally stabilize Hb to avoid the oxidation process of the haem-containing protein and its denaturation [Citation38,Citation39]. Cross-linking of Hb by GA has many drawbacks such as reduction of redox potential as well as increase of its autoxidation rate [Citation40]. This could be the reason for the different amount of functional Hb in GA-HbMP and Odex-HbMP (40% in Odex-HbMP and 30% in GA-HbMP). This value was stable during storage for Odex-HbMP but not for GA-HbMP where the functional Hb decreased rapidly with the storage time. We measured the amount of released oxygen and calculated from these numbers the amount of functional Hb. Therefore, we cannot directly conclude that the remaining 60% of the measured total Hb is MetHb. It could also be possible that the oxygen release from the particles is partly hindered and the amount of MetHb is lower than 60%. Direct measurements of the MetHb content in the particles are in progress and will give a better insight.
Particle size is a significant feature in determining the performance of particles in biological applications because it influences the biological properties, such as cellular uptake, circulating half-life and biodistribution. Particles fabricated by our new “one-pot” procedure fit to the criteria for the biomedical usages [Citation41]. This procedure provided the uniform morphology, monodisperse, size range between 800 and 1000 nm. The Odex particles have negative zeta-potential, suggestive that the particles have a tendency to repel each other, rendering the colloid stable.
The results of analytical centrifugation clearly showed the deformability of Odex-cross-linked particles, and they were compressed when applied high centrifugal force. However, they were dilated when the force was reduced. The deformability of particles was also confirmed by scanning several Odex-HbMP by AFM in dry and wet states. An increase in thickness gives some evidence for a sponge-like structure of the particles with a high water content. Upon drying, the particles shrink but recover their thickness quickly when water is added. The deformability of particles is very important for the intravenous administration because they need to circulate through the bloodstream and pass through microvessels.
In terms of protein functionality, the UV-VIS spectrophotometric analysis in exhibited the ability of Odex-HbMP to bind and release oxygen in comparison with stroma-free Hb. Oxygenated Odex-HbMP demonstrated three absorption peaks at 414, 542 and 576 nm, which correspond to the oxygenated Hb (OxyHb). After deoxygenation of the Odex-HbMP using 1 mg/mL sodium dithionite (SDT), a red shift of the 414 nm peak (the Soret peak) to 432 nm was observed. A peak at 556 nm was detected instead while the peaks at 542 and 576 nm disappeared. The absorption spectra of Odex-HbMP showed the characteristic shape of oxygenated/deoxygenated Hb and confirmed the functionality of Odex-HbMP. The shift to higher absorption values of Odex-HbMP spectra compared to the Hb solution is due to the scattering of Odex-HbMP.
The stability of particles is one of the most important factors influencing their medical and pharmaceutical applications. We could show that there was no significant change of the particle size, size distribution as well as of the total Hb content and the fraction of functional Hb of the Odex-HbMP during at least 3 months storage. This information indicated the long shelf life of our new particles which is a huge benefit.
Conclusion
We have successfully established a novel, simple, efficient and cost-effective method for biopolymer microparticle fabrication modified from CCD technique using Odex as a biocompatible cross-linker. Monodisperse HbMP could be prepared by coprecipitation of Hb with the MnCO3 template and cross-linking with Odex in one step. After removing MnCO3 template with EDTA, the resulting Odex-HbMP showed homogeneous morphology with a size of about 850–1000 nm, negative zeta-potential, high deformability, high entrapment efficiency of Hb and a high fraction of functional Hb. The Odex-HbMP maintained their ability to be oxygenated/deoxygenated and they are stable at least for 90 days. The results suggest that Odex has a potential to be used as a promising alternative cross-linker for the preparation of submicron particles. We eagerly anticipate a potential of our new technique for a wide range of biomedical applications soon.
Disclosure statement
No potential conflict of interest was reported by the authors.
Additional information
Funding
References
- Zhang Y, Chan HF, Leong KW. Advanced materials and processing for drug delivery: the past and the future. Adv Drug Deliv Rev. 2013;65:104–120.
- Teekamp N, Duque LF, Frijlink HW, et al. Production methods and stabilization strategies for polymer-based nanoparticles and microparticles for parenteral delivery of peptides and proteins. Expert Opin Drug Deliv. 2015;12:1311–1331.
- Keshani S, Daud WRW, Nourouzi MM, et al. Spray drying: an overview on wall deposition, process and modeling. J Food Eng. 2015;146:152–162.
- Vehring R. Pharmaceutical particle engineering via spray drying. Pharm Res. 2008;25:999–1022.
- Gao X, Zhang X, Wu Z, et al. Synthesis and physicochemical characterization of a novel amphiphilic polylactic acid-hyperbranched polyglycerol conjugate for protein delivery. J Control Release. 2009;140:141–147.
- Bäumler H, Xiong Y, Liu ZZ, et al. Novel hemoglobin particles-promising new-generation hemoglobin-based oxygen carriers. Artif Organs. 2014;38:708–714.
- Xiong Y, Steffen A, Andreas K, et al. Hemoglobin-based oxygen carrier microparticles: synthesis, properties, and in vitro and in vivo investigations. Biomacromolecules. 2012;13:3292–3300.
- Ba¨umler H, Georgieva R. Coupled enzyme reactions in multicompartment microparticles. Biomacromolecules. 2010;11:1480–1487.
- Habeeb AFSA, Hiramoto R. Reaction of proteins with glutaraldehyde. Arch Biochem Biophys. 1968;126:16–26.
- Lai J-Y. Interrelationship between cross-linking structure, molecular stability, and cytocompatibility of amniotic membranes cross-linked with glutaraldehyde of varying concentrations. RSC Adv. 2014;4:18871–18880.
- Reddy N, Reddy R, Jiang Q. Crosslinking biopolymers for biomedical applications. Trends Biotechnol. 2015;33:362–369.
- Sommermeyer K, Eichner W, inventor. Haemoglobin-hydroxyethyl starch conjugates as oxygen carriers. United States patent US 6,083,909, Assignee: Fresenius AG, Oberusel, Germany; 2000
- Eike JH, Palmer AF. Oxidized mono-, di-, tri-, and polysaccharides as potential hemoglobin cross-linking reagents for the synthesis of high oxygen affinity artificial blood substitutes. Biotechnol Prog. 2004;20:953–962.
- Scatena R, Giardina B. O-raffinose-polymerised haemoglobin. A biochemical and pharmacological profile of an oxygen carrier. Expert Opin Biol Ther. 2001;1:121–127.
- Boykins RA, Buehler PW, Jia Y, et al. O-raffinose crosslinked hemoglobin lacks site-specific chemistry in the central cavity: structural and functional consequences of beta93Cys modification. Proteins Struct Funct Genet. 2005;59:840–855.
- Bonneaux F, Labrude P, Dellacherie E. Preparation and oxygen binding properties of soluble covalent hemoglobin-dextran conjugates. Experientia. 1981;37(8):884–886.
- Bonneaux F, Dellacherie E, Labrude P, et al. Hemoglobin-dialdehyde dextran conjugates: improvement of their oxygen-binding properties with anionic groups [Internet]. J Protein Chem. 1996;15:461.
- McCahon R, Hardman J. Pharmacology of plasma expanders [Internet]. In: Nick P, Mick S, editors. Anaesthesia and intensive care medicine. Vol. 18. Netherlands: Elsevier Amsterdam; 2017. p. 418–420.
- Mehvar R. Dextrans for targeted and sustained delivery of therapeutic and imaging agents. J Control Release. 2000;69:1–25.
- Maia J, Evangelista MB, Gil H, et al. Dextran-based materials for biomedical applications. Carbohydr Appl Med. 2014;661:31–53.
- Maia J, Carvalho RA, Coelho JFJ, et al. Insight on the periodate oxidation of dextran and its structural vicissitudes. Polymer (Guildf). 2011;52:258–265.
- Wasiak I, Kulikowska A, Janczewska M, et al. Dextran nanoparticle synthesis and properties. PLoS One 2016. 11(1): e0146237. doi:10.1371/journal.pone.0146237
- Lisman A, Butruk B, Wasiak I, et al. Dextran/albumin hydrogel sealant for Dacron(R) vascular prosthesis. J Biomater Appl. 2014;28:1386–1396.
- Cortesi R, Esposito E, Osti M, et al. Dextran cross-linked gelatin microspheres as a drug delivery system. Eur J Pharm Biopharm. 1999;47:153–160.
- Palmer AF, Sun G, Harris DR. Tangential flow filtration of hemoglobin. Biotechnol Prog. 2009;25:189–199.
- Haney CR, Buehler PW, Gulati A. Purification and chemical modifications of hemoglobin in developing hemoglobin based oxygen carriers. Adv Drug Deliv Rev. 2000;40:153–169.
- Berillo D, Elowsson L, Kirsebom H. Oxidized dextran as crosslinker for chitosan cryogel scaffolds and formation of polyelectrolyte complexes between chitosan and gelatin. Macromol Biosci. 2012;12:1090–1099.
- Muangsiri W, Kirsch LE. The protein-binding and drug release properties of macromolecular conjugates containing daptomycin and dextran. Int J Pharm. 2006;315:30–43.
- Xiong Y, Liu ZZ, Georgieva R, et al. Nonvasoconstrictive hemoglobin particles as oxygen carriers. ACS Nano. 2013;7:7454–7461.
- Xiong Y, Georgieva R, Steffen A, et al. Structure and properties of hybrid biopolymer particles fabricated by co-precipitation cross-linking dissolution procedure. J Colloid Interface Sci. 2018;514:156–164.
- Haldane J. The ferricyanide method of determining the oxygen capacity of blood. J Physiol (Lond). 1900;25:295–302.
- Cook SF. The action of potassium cyanide and potassium ferricyanide on certain respiratory pigments. J Gen Physiol. 1928;11:339–348.
- Bäumler H, Hamberger L, Zaslansky P, et al. Non-destructive mechanical testing of allograft bone-implants by analytic centrifugation. Exp Mech. 2016;56:1653–1660.
- Kao I, Xiong Y, Steffen A, et al. Preclinical in vitro safety investigations of submicron sized hemoglobin based oxygen carrier HbMP-700. Artif Organs. 2018;42:549–559.
- Gómez-Mascaraque LG, Méndez JA, Fernández-Gutiérrez M, et al. Oxidized dextrins as alternative crosslinking agents for polysaccharides: application to hydrogels of agarose–chitosan. Acta Biomater. 2014;10:798–811.
- Ribeiro MP, Morgado PI, Miguel SP, et al. Dextran-based hydrogel containing chitosan microparticles loaded with growth factors to be used in wound healing. Mater Sci Eng C. 2013;33:2958–2966.
- Fuentes M, Segura RL, Abian O, et al. Determination of protein-protein interactions through aldehyde-dextran intermolecular cross-linking. Proteomics. 2004;4:2602–2607.
- Wang Y, Zhang S, Zhang J, et al. Structural, functional and physiochemical properties of dextran-bovine hemoglobin conjugate as a hemoglobin-based oxygen carrier. Process Biochem. 2017;60:67–73.
- Zhang J, Wang Y, You G-X, et al. Conjugation with 20 kDa dextran decreases the autoxidation rate of bovine hemoglobin. Artif Cells Nanomed Biotechnol. 2018;46:1436–1438.
- Guillochon D, Vijayalakshmi MW, Thiam-Sow A, et al. Effect of glutaraldehyde on hemoglobin: functional aspects and Mössbauer parameters. Biochem Cell Biol. 1986;64:29–37.
- Mayer A, Vadon M, Rinner B, et al. The role of nanoparticle size in hemocompatibility. Toxicology. 2009;258:139–147.