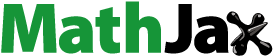
Abstract
Neuroprotection in ischaemic stroke prevents neuronal injury and subsequent death. Our earlier work revealed the neuroprotective effect of ethylacetate fraction (EF) obtained from Allium cepa outer scales in a mouse model of cerebral ischaemia–reperfusion (I–R) injury. The present study was designed to develop and optimize a liposomal delivery system for EF, along with its biological assessment. Thin film hydration method was used for the preparation of liposomal formulation. The prepared liposomes were optimized with respect to particle size, size distribution and encapsulation efficiency (EE) and characterised on the basis of zeta potential, in vitro release, morphology (TEM) and physical stability. The biological activity of the optimized liposomal formulation (EF-L; 8.5 mg/kg, intra-nasal) was evaluated after induction of cerebral injury in the experimental animals. Neuroprotective effects were assessed in terms of improvement of cognitive/sensorimotor functions and reduction of cerebral infarct size and brain oxidative stress. EF-L (particle size of 204.93 ± 7.96 nm; EE of 88.02 ± 2.09%; zeta potential of –20.8 ± 1.24 mV) showed controlled release pattern; spherical shape and were physically stable for 60 days at 4 °C. Intra-nasal administration of EF-L produced significant neuroprotection in mice at 1/10th the oral dose. Thus, EF-L may be developed as a neuroprotective formulation.
Introduction
Ischaemic stroke, a condition in which blood supply to the brain is reduced, is one of the leading causes of physical disability and mortality worldwide [Citation1]. Tissue plasminogen activator (t-PA) is the only FDA approved drug for the treatment of ischaemic stroke but it has a narrow therapeutic window of 3 h [Citation2]. Ironically, reperfusion results in secondary impairment called as ischaemia–reperfusion (I–R) injury, which exacerbates the ischaemic injury by causing massive generation of free radicals leading to neuronal damage and thus, functional deficit [Citation3]. Hence, finding an effective pharmacological drug for the treatment of I–R injury is of utmost importance.
Researchers have always considered nature a significant source of drugs [Citation4]. Herbal drugs containing flavonoids are gaining attention due to their wide spectrum of biological activities and are being explored for possible neuroprotective capacity [Citation5]. Allium cepa (Onion) is cultivated worldwide and used traditionally, as well as in the modern system of medicine to treat various disorders including diabetes, cancer, hypertension, jaundice and brain disorders [Citation6–9]. The biological activities of onion were attributed to the presence of an appreciable amount of flavonoids possessing antioxidant effects [Citation10]. There is an increasing attention on the antioxidant content of onions because regular consumption is associated with reduced risk of cardiovascular and neurodegenerative disorders, many forms of cancer and cataract formation [Citation9].
To overcome the complexities, such as poor BBB permeability, unwanted distribution and rapid metabolism of drugs, associated with drug delivery of anti-stroke agents, administration of drugs with nanocarriers through intra-nasal (IN) route were thought of as an alternative [Citation11]. Therapeutic agents are typically encapsulated, entrapped, adsorbed or chemically attached to the nanoparticle surface for enhancing their efficacy at the target site. Based on this hypothesis certain nanocarriers like liposomes, nanotubes, hydrogels, dendrimers, polymeric particles, inorganic/solid particles, micelles and quantum dots have been developed for effective delivery of the therapeutics such as Pittsburgh compound B [Citation12,Citation13], connexin 43 [Citation14], tamoxifen [Citation15], doxorubicin [Citation16], riluzole [Citation17], quercetin [Citation18] to the brain for the treatment of various brain disorders [Citation19]. In recent years, liposomes have been explored as carriers of therapeutic drugs, imaging agents and genes, in particular for treatment and/or diagnosis of neurological diseases [Citation20]. In this way, liposomes have emerged as promising carriers for CNS delivery.
Liposomes are nano-sized vesicles consisting of an outer lipid layer encapsulating the inner aqueous core [Citation21]. Thus, they have an added advantage of incorporating and delivering both lipid and water soluble drugs [Citation22]. Because of their ability to cross blood–brain barrier, liposomes can augment the efficacy of neuroprotectants by enhancing the bioavailability of encapsulated actives to the brain [Citation23]. Recently, liposomal delivery systems of Withania somnifera, fasudil and tacrolimus have been developed for the treatment of stroke [Citation24–26]. Furthermore, IN route delivers the therapeutics directly to CNS through olfactory lobes and trigeminal nerve pathways [Citation27]. It is well established that IN delivery of drugs in animals and humans is effective in treating neurological disorders including epilepsy, depression, migraine, stroke and Alzheimer’s [Citation28,Citation29]. Thus, a combination of IN delivery route with nanotechnology-based carrier system (liposomes) may unbolt positive horizons for the treatment of cerebral I–R injury.
In our previous study, marked neuroprotective effects of ethylacetate faction (EF) separated from A. cepa outer scales hydromethanol extract (ACE) against cerebral injury in mice were observed [Citation30]. In continuation with the previous report, the present study was aimed to design and optimize an efficient delivery system and route (liposome through intra-nasal route) of EF to confer neuroprotection against I–R-induced cerebral injury in mice at a lower dose.
Material and methods
Chemicals
Reduced glutathione (GSH), 5,5'-dithiobis-(2-nitrobenzoic acid) (DTNB), 1,1,3,3-tetraethoxypropane and quercetin were obtained from Sigma (St. Louis, MO). Soy phosphatidylcholine (soy lecithin) and cholesterol were purchased from Himedia Pvt Ltd. (Mumbai, India). For chromatographic studies, HPLC grade solvents were used. All other chemicals were of analytical grade.
Preparation of ethylacetate fraction from A. cepa outer scales
Allium cepa var. NHRDF Red bulbs were procured from a cultivated source, National Horticulture Research and Development Foundation (NHRDF), Bathinda, Punjab, India in November 2013 (voucher number NHRDF/SC/BTI/2013 14/458). The variety is released by NHRDF and notified by Government of India (Vide notification number SO-2035 dated 28/10/2006). The ethylacetate fraction (EF) from A. cepa outer scales was prepared as described in . The percentage yield of EF was 42.50% (w/w) with respect to dried A. cepa outer scale hydromethanol extract (ACE).
HPLC method development and validation for estimation of quercetin
In our previous study quercetin was found as a major compound present in the bioactive EF [Citation30]. Therefore, in the present study, an HPLC method for the estimation of quercetin was developed, which was used during the development and optimization of the EF-loaded liposomal formulation. The samples obtained from encapsulation efficiency and in vitro release studies were quantitatively analyzed for quercetin concentration using an HPLC system (Waters, Milford, MA) equipped with binary pump (1525 Waters), UV–Vis detector (Waters 2487) and reversed phase C18 column (Spherisorb ODS2, 4.6 × 250 mm, 5 μ). Empower software version 2 (Waters) was used for data collection and integration. A stock solution of quercetin (1 mg/ml) was diluted to obtain different concentrations and 20 µl of each concentration was manually injected onto column after filtered through a 0.45-μm nylon membrane. Appropriate separation of quercetin peak was achieved by isocratic elution with mobile phase methanol and water containing 0.1% O-phosphoric acid (70:30 v/v) at a flow rate of 1 ml/min at room temperature. The runtime was 20 min for each injection. The analysis was carried out at 366 nm (λmax). This method was validated for linearity (1–10 µg/ml), intra-day and inter-day precision, limit of quantification (0.9 µg), limit of detection (0.3 µg) and accuracy (98.76%). The linearity equation (y = 106004x–15731) was then used to determine the unknown quercetin concentration in various samples.
Development of liposomal delivery system
Preparation of blank liposomes
Thin film hydration method was used for the preparation of liposomes [Citation26]. In brief, phosphatidylcholine and cholesterol were dissolved in different ratios (50 + 50, 60 + 40, 70 + 30, 80 + 20 and 90 + 10 on %w/w basis), separately, with 5 ml mixture of chloroform and methanol (2:3 v/v) in 250 ml round bottom flask. The final lipid concentration in liposomal formulation was 5% w/v [Citation31]. The organic solvent was removed under vacuum using a rotary evaporator to obtain a thin film. For complete removal of organic solvent, the film was allowed to dry for 60 min. Later, the film was hydrated by agitating with phosphate buffer having pH similar to a nasal fluid, i.e. pH 6.3 (10 ml). This mixture was allowed to hydrate for 2 h at room temperature followed by bath sonication for 3 min to get small multi-lamellar blank liposomes.
Preparation and optimization of EF-loaded liposomes
To prepare drug-loaded liposomes, EF was added along with the different ratio of phosphatidylcholine and cholesterol and process used to prepared blank liposome was repeated. The concentration of EF in the liposomal formulation was initially fixed to 10% of total lipid mass and the best formulation was determined on the basis of particle size, polydispersity index (PDI), and encapsulation efficiency (EE). In order to determine the maximum amount of EF that can be loaded into liposomal vesicles, different formulations were prepared with increasing amount of EF (up to 25% w/w of total lipid mass) to the best formulation. The formulation with maximum EE was choose as final optimized formulation (EF-L) whose zeta potential, TEM, in vitro release, physical stability and neuroprotective effects were determined.
Characterization of prepared liposomes
Particle size and size distribution
The blank and EF-loaded liposomes were subjected to measurement of particle size and PDI by a laser diffraction particle size analyzer (Zeta-sizer, Nano ZS model; Malvern Instruments UK, Milford, MA). Each sample was measured in triplicate and Z average (d. nm) and the poly-dispersity index (PDI) was determined.
Entrapment efficiency (EE)
To determine the EE of various formulations, the unentrapped drug was separated by the dialysis method [Citation31,Citation32]. The liposomal dispersion (2 ml) was encased in cellophane membrane (molecular weight cut-off 12,000–14,000; HiMedia, Mumbai, India) tied at both the ends and was placed in a beaker containing 50 ml phosphate buffer pH 6.3 (receptor fluid). Samples (2 ml) were withdrawn from the receptor phase at time intervals and replaced with fresh buffer. The drug content in samples was determined with respect to quercetin (major quantifiable marker in EF) using a validated HPLC method. The experiment was stopped when constant drug concentration values were obtained in subsequent withdrawals from the receiver phase [Citation31]. The amount of drug entrapped in the liposomal formulation was determined by HPLC assay after lysing the dialyzed formulation with 0.1% (v/v) triton-X 100. The percentage EE was calculated using the formula:
where Ct is the concentration of total drug and Cr is the concentration of drug in lysed solution.
Zeta potential
Zeta potential of EF-L was determined in triplicate by laser Doppler anemometry using a Zeta-sizer (Nano ZS model; Malvern Instruments UK, Milford, MA).
Transmission electron microscopy (TEM) study
The morphology of liposomes in EF-L was examined using TEM studies. The sample was negatively stained with a phosphotungstic acid solution and observed under an electron microscope (Hitachi TEM system, Milford, MA).
In vitro release study
The release of EF (with respect to quercetin) from EF-L was carried out using the dialysis bag method [Citation31,Citation33]. EF-L (2 ml) and EF suspension (2 mg EF in 2 ml phosphate buffer pH 6.3) was encased, separately, in cellophane membrane (molecular weight cut-off 12,000–14,000; HiMedia) tied at both ends and immersed in 20 ml receptor medium comprising of artificial nasal fluid (ANF) containing 1% sodium lauryl sulphate (SLS) [Citation34]. Samples (2 ml) were taken from receptor compartment at a time interval of 0, 0.25, 0.5, 0.75, 1, 2, 4, 6, 8, 10, and 12 h and the same volume was immediately replaced with ANF containing 1% SLS to maintain sink conditions. The drug content was determined with respect to quercetin in each sample, in triplicate, using HPLC assay and cumulative release percentage was calculated. The release data were fitted to various kinetic models such as zero order, first order, Higuchi’s equation, Korsmeyer–Peppas and Hixson–Crowell models.
Stability studies
EF-L was stored in a covered transparent vial at 4 °C for 60 days. Samples were withdrawn periodically and physical stability was assessed by determining any change in physical appearances like a variation of colour, sedimentation and aggregation, particle size, size distribution and zeta potential on 0, 30th and 60th day [Citation26].
Evaluation of neuroprotective effects of EF-L
Animals
Swiss Albino mice, either sex, weighing 25–30 g (3–4 week old) were obtained from Lala Lajpat Rai University of Veterinary and Animal Sciences, Hisar, Haryana, India. Animals were kept under controlled environmental conditions (temperature 25 ± 2 °C with 12 h light–dark cycle) and had free access to food and water. Experimental studies were performed between 8:00 and 14:00. The experimental protocol was reviewed and approved by Institutional Animal Ethics Committee (107/GO/ReBi/S/99/CPCSEA/2017–38) and is in accordance with the guidelines of the Committee for the Purpose of Control and Supervision of Experiments on Animals (CPCSEA), Ministry of Environment and Forest, Government of India.
Induction of cerebral I–R injury
Bilateral common carotid artery occlusion (BCCAO) method, as described by Singh et al. [Citation35], was used to induce cerebral I–R injury in mice. In brief, animals were anaesthetised with chloral hydrate (350 mg/kg, i.p.) and placed in the supine position. A midline incision was made in the neck region and the left and right carotid arteries were exposed. Both arteries were freed from surrounding tissues. Ischaemia was induced for 15 min by occluding both arteries with cotton thread. After ischaemia thread was removed and skin was sutured in layers. The sutures were cleaned with disinfectant and sprayed with Neosporin antibiotic. Animals were returned to their home cage after the surgical procedure and were allowed to recover for 24 h (reperfusion). BCCAO followed by 24 h reperfusion shall be termed as BCCAO hereafter. For sham control animals, the same surgical procedure was followed but ischaemia was not induced, i.e. arteries were not occluded.
The animals were divided into following groups:
Group I: Sham control (n = 6)
Group II: Animals administered vehicle after BCCAO (BCCAO + vehicle; n = 6; p.o.)
Group III: Animals administered EF after BCCAO (BCCAO + EF 85 mg/kg; n = 6; p.o.)
Group IV: Animals administered BL after BCCAO (BCCAO + BL; n = 6)
Group V: Animals administered EF-L after BCCAO (BCCAO + EF-L 8.5 mg/kg; n = 6)
Vehicle (0.5% w/v carboxymethylcellulose sodium in distilled water) or EF was administered to animals for 7 days once daily after BCCAO through oral route with a maximum volume of 10 ml/kg. Blank liposome (BL) or EF-L was administered to animals for 7 days once daily through the nasal route with a maximum volume of 15 µl each nostril [Citation36].
Effect of various treatments on cognitive functions
The effect of various treatments on cognitive functions in mice after BCCAO was evaluated using the Morris Water Maze (MWM) test from day 3 to day 7 of the study period [Citation37]. In brief, a platform was hidden in a circular pool of water (divided into 4 equal quadrants) and animals were trained (4 trainings per day) from day 3 to day 6 to find the hidden platform. The time taken by an animal to find the hidden platform, called escape latency time (ELT), was recorded and considered an index of acquisition. On day 7, the platform was removed and animals were again allowed to find the hidden platform. The time spent in the target quadrant (TSTQ) on day 7 was considered as an index of retention [Citation35].
Effect of various treatments on neurological functions
The effect of various treatments on neurological functions after BCCAO was evaluated on day 7 using the neurological severity score (NSS) [Citation35]. NSS (ranging from 0 to 15) assesses balance, motor and reflex parameters in a composite manner (). A higher NSS indicates a greater neurological deficit.
Table 1. Tests performed and their scores in NSS.
Effect of various treatments on cerebral infarct size
After completion of behavioural studies, animals were sacrificed by cervical dislocation under mild anaesthesia. The brains were separated and frozen at –5 °C. Coronal sections (1 mm thickness) of brain samples were made and stained with 2,3,5-triphenyltetrazolium chloride (TTC) as described by Singh et al. [Citation35]. The stained slices were photographed and infarcted area (yellow area) was calculated with Image J software (NIH Image 1.61; National Institute of Health; Bethesda, MD).
Effect of various treatments on brain biochemical parameters
Animals were decapitated at the end of behavioural studies to remove brains. A homogenate (10% w/v in phosphate buffer pH 7.4) was prepared and centrifuged at 14,500 rpm for 15 min at 4 °C. The total protein content [Citation38], reduced glutathione (GSH) levels [Citation39], superoxide dismutase (SOD) activity [Citation40] and TBARS levels [Citation41] were determined in the clear supernatant.
Statistical analysis
Data were presented as mean ± SD. The results of the MWM test were statistically analyzed using two-way analysis of variance (ANOVA) followed by Tukey’s test as post hoc analysis. Data from biochemical estimations were compared by one-way ANOVA followed by Tukey’s test as post hoc analysis. A value of p < .05 was considered statistically significant.
Results and discussion
Combination of lipid-based drug delivery system (liposome) and its administration through IN route offers several advantages, the most significant being the enhanced delivery of active principles to the brain [Citation42]. Higher the availability in the brain better is the therapeutic effect of the drug. With this aim, the present study was envisaged to design and optimize the delivery system (liposome) and route (IN) for efficient delivery of ethylacetate fraction (EF) separated from ACE to be used in the event of cerebral I–R injury.
Selection of lipid composition
Phosphatidylcholine (an unsaturated lipid chain) is an ideal primary component of the liposomal system, which forms the vesicle for the entrapment of drug [Citation22]. Addition of cholesterol imparts the vesicle rigidity by filling in the free spaces formed due to the kink in the unsaturated lipid chain. Therefore, the selection of the right amount of both phosphatidylcholine and cholesterol is essential as inappropriate amounts result in reduced membrane permeability and drug release, which is a disadvantage in drug targeting [Citation21]. In the present study, various batches of blank liposomes were prepared by employing different compositions of phosphatidylcholine and cholesterol using film hydration method ().
Table 2. Particle size and PDI of blank liposomes.
Incorporation of an equal amount of phosphatidylcholine and cholesterol, i.e. 50% resulted in clumping and ridging of the film causing failure of uniform film formation.
With the increase in phosphatidylcholine concentration and a decrease in cholesterol concentration in the formulation, improvement in film formation was observed. Possibly due to uniform film formation, smaller particle size and PDI was noted when 70% phosphatidylcholine and 30% cholesterol were used to prepare blank liposomal formulation (B3).
With further increase in phosphatidylcholine amount and a decrease of cholesterol concentration up to 9:1, breakage in film formation was observed and thus, a film formed was not continuous and higher particle size was recorded (). Amount of cholesterol determines the membrane stability, fluidity and flexibility. Probably due to low cholesterol content, the vesicles formed were not stable and formulation B5 had a higher average particle size (292.06 nm) and PDI (0.394).
Film formed with 30% cholesterol and 70% phosphatidylcholine resulted in the formation of the continuous uniform film, which had desired fluidity as it was easily dispersed in hydration media. Moreover, blank liposomes obtained with formulation named B3 had a lowest average particle size (179.53 nm) with acceptable PDI (0.257), which confirmed the homogeneity of the system ().
Preparation and optimization of EF-loaded liposomes
For optimization of EF-loaded liposomes, different formulations were prepared by varying lipid composition with 10% EF and their particle size, PDI and EE were determined (). Previous studies have shown the development of optimized liposomal formulation with the addition of 10% herbal extracts to total lipid content [Citation26,Citation32]. Considering these studies, 10% EF was used to initially prepare the liposomal formulation in the present study. As observed in blank liposomes, on varying lipid content similar pattern of change in particle size was observed in EF-loaded liposomal formulations (). The particle size of all EF-loaded liposomal formulations was found to be higher than corresponding blank liposomes ( and ). Such an effect confirmed the incorporation of EF into the vesicle system. The increase of particle size after EF addition might also be due to the fact that EF contained diverse chemical compounds, which are mostly lipophilic (e.g. quercetin) that localised in the lipid bilayer thus increasing the size of liposomes. Similar results have also been observed in a previous study showing an increase in liposome size with extract incorporation [Citation32].
Table 3. Particle size, PDI and encapsulation efficiency of drug-loaded liposomes.
Further increase in EE was observed with increase in phosphatidylcholine amount and maximum EE (78.80 ± 2.52%) was observed with a B3 formulation having 70% phosphatidylcholine and 30% cholesterol with small particle size and acceptable PDI (). This could be because the addition of cholesterol in this ratio provides optimum rigidity to the bilayer. Further increase in phosphatidylcholine and a decrease in cholesterol contents reduced the EE of the system. It has been documented that optimum cholesterol level is required to maintain the membrane rigidity which prevents drug leakage [Citation26]. The decrease in EE in formulation B4 (72.91 ± 6.51%) and B5 (59.30 ± 5.59%) in the present study may be attributed to drug leakage from the system because of the presence of less amount of cholesterol.
As B3 showed acceptable particle size, PDA and EE, therefore, it was selected for further optimization.
To evaluate the effect of drug content on EE of the liposomal system, amount of EF in the best formulation (B3) was increased up to 25% (w/w) of total lipid content. Addition of drug up to 20% of lipid content showed increased EE with a maximum EE of 88.02 ± 2.09%. Further increase in drug content reduced the EE of the system (). This can be explained by the fact that lipid bilayer could only incorporate a certain amount of drug after which the stable liposomal bilayer starts sedimenting immediately on the formation.
Table 4. Effect of drug content on particle size, PDI and entrapment efficiency of liposome formulation.
From the above findings, it was concluded that B3 with 20% EF was best in terms of particle size, PDI and EE. Hence, it was chosen as the final optimized formulation (EF-L) and used for further investigations
Determination of zeta potential
The zeta potential of EF-L was found to be –20.8 ± 1.24 mV which confirmed the formation of anionic liposomes. It is documented that higher zeta potential is associated with better physical stability of formulation as it causes intra-particle repulsion and reduces the chances of coalescence [Citation43,Citation44]. Hence, the formulation optimized in the present study was stable.
In vitro release study
The cumulative release of EF (determined with respect to quercetin) from non-formulated and EF-L in an artificial nasal fluid containing 1% sodium lauryl sulphate was studied by in vitro dialysis bag method. The release of quercetin from EF-L was significantly higher (p < .05) compared with non-formulated EF from 0.5 to 12 h. The maximum percentage cumulative release of quercetin from EF-L was 71.70% at 12 h. The release was observed as two phases (). Initially, a rapid release phase was observed during first 1 h, which could be related to the release of drug adsorbed to the surface of the liposomes. The second phase was a slow release demonstrating typically sustained and prolonged drug release behaviour. The decrease in the release may be attributed to the good interaction between EF and phospholipid carrier, which caused its slow release from liposomal formulation [Citation45]. In addition, liposomes serve as a rate-limiting membrane barrier thus providing a controlled system. The results are in agreement with earlier reports suggesting the biphasic nature of liposomal dispersion [Citation44,Citation46]. In contrast, EF suspension (non-formulated) showed immediate release behaviour.
Figure 2. Per cent cumulative release profile of quercetin from optimized EF-loaded liposomal formulation (EF-L) and non-formulated EF (EF) using in vitro dialysis membrane method.
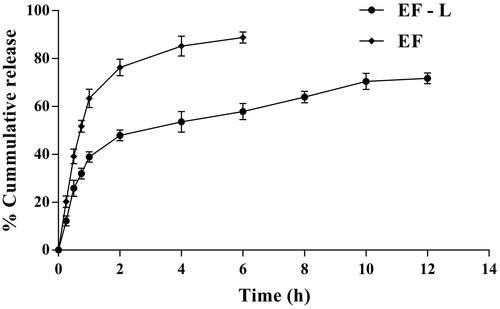
The release data were kinetically treated and evaluated based on the correlation coefficient (r2) values obtained. The results () showed higher r2 value for Higuchi’s model compared with other models, suggesting that the drug transport occurred mainly by diffusion-controlled mechanism [Citation47]. To explore the mechanism of drug release, results of the in vitro release data were further fitted to the Korsmeyer–Peppas equation. The release exponent (n value) was found to be 0.367. This implies that the release of quercetin from EF-L was based on Fickian diffusion [Citation12].
Table 5. Kinetic parameters for EF release from the liposomal formulation.
Because of the diffusion release exponent value, n = 0.236, it can be concluded that mechanism of drug release in Korsmeyer–Peppas model is based on the phenomenon of diffusion [Citation48].
TEM analysis
The morphology of EF-L was confirmed by TEM, which verified the spherical shape of liposomes ().
Stability studies of EF-L
Evaluation of stability for a formulation is an important parameter when developing it as a commercial product. The physical stability of EF-L was assured over a period of 60 days at 4 °C by determining physical appearances like a change of colour, sedimentation and aggregation, particle size, PDI and zeta potential. Results showed no change of colour, sedimentation and aggregation in the optimized formulation over a period of 60 days (). in addition, no statistically significant change in particle size, PDI and zeta potential was observed, which indicated that optimized formulation was stable over the study period ().
Table 6. Physical stability parameters for EF-L.
Evaluation of neuroprotective effects of EF-L
Neuroprotective effects of EF-L administered through nasal route were determined by evaluating cognitive and sensorimotor functions, cerebral infarct size and brain oxidative stress against BCCAO-induced cerebral injury in mice. In the present study, BCCAO method was used to induce cerebral injury because it is well documented that this mimics clinical conditions of ischaemic stroke and impairs normal cognitive and sensorimotor functions [Citation6,Citation49].
Effect of EF-L treatment on cognitive functions
It is reported that hippocampal neurons die in 2–4 days after I–R insult leading to cognitive impairment in animals [Citation50–52]. MWM test is a well-established model to evaluate the spatial memory (cognitive functions) of animals [Citation53]. Thus, in the present study cognitive functions of various groups were evaluated using the MWM test from day 3 to day 7 of the study period. All the groups showed statistically similar day 3 ELT possibly because animals were naïve to the apparatus and had no previous training. From day 3 to day 6 a sharp decline (statistically significant) in ELT was observed in sham group, which showed the normal learning ability of animals. In addition, day 7 TSTQ (Q4) was found to be significantly higher than other quadrants (Q1, Q2 and Q3) in sham group confirming normal cognitive functions of mice ( and ). In a vehicle-treated group, significantly higher day 6 ELT and lower TSTQ was observed compared with sham animals indicating cognitive impairment effects of BCCAO in animals. These results are supported by previous reports showing BCCAO induced cognitive impairment in animals [Citation35,Citation54]. Treatment of animals with EF-L by IN route after BCCAO exhibited significantly lower day 6 ELT and higher TSTQ in comparison with the vehicle-treated group showing beneficial effects of EF-L against BCCAO in mice. Furthermore, IN administration of blank liposomes (BL) did not show a significant change in day 6 ELT and TSTQ in MWM test in comparison with the vehicle-treated group, which confirmed that cognitive improvement effects of EF-L were due to the presence of EF not due to the phospholipids of the formulation ( and ). In comparison with EF treated group, animals administered with EF-L showed statistically similar improvement in cognitive functions in the MWM test. This suggested that same cognition improvement effects against I–R injury could be observed with EF at a lower dose when administered as liposomes through IN route.
Figure 4. Effect of optimised liposomal formulation on time spent in the target quadrant (TSTQ) of mice after I–R-induced cerebral injury using MWM test. Data were presented as mean ± SD and analyzed by two-way ANOVA followed by Tukey’s test as post hoc analysis. ap < .05 vs. time spent in Q1, Q2, Q3 quadrants of the sham group; bp < .05 vs. TSTQ (Q4) of the sham group; cp < .05 vs. TSTQ (Q4) of BCCAO + Vehicle group; dp < .05 vs. TSTQ (Q4) of BCCAO + BL group. EF: ethyl acetate fraction; BL: blank liposomes; LF: EF-loaded optimized liposomal formulation. The doses are in mg/kg.
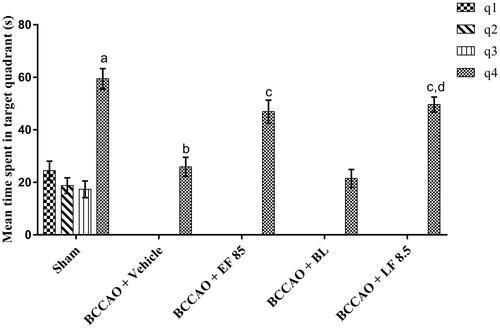
Table 7. Effect of optimised liposomal formulation on ELT of mice in MWM after I–R-induced cerebral injury.
Effect of EF-L treatment on sensorimotor functions
Cerebral I–R injury is documented to cause motor and sensory reflex disturbances in animals [Citation55]. In harmony with these reports, higher NSS was observed, in the present investigation, in the vehicle-treated group compared with the sham group, which showed BCCAO-induced sensorimotor disturbances in animals. The NSS was found to be significantly reduced when animals were treated with EF-L through IN route for 7 days in comparison with vehicle-treated animals indicating beneficial effects of EF-L against BCCAO (). Furthermore, animals treated with blank liposomes (BL) did not show a significant change in NSS in comparison with vehicle-treated group. This confirmed that the improvement in sensorimotor functions of animals with EF-L treatment after BCCAO was exclusively due to encapsulated EF in liposomes. In addition, in comparison with EF treated group, animals administered with EF-L showed statistically similar improvement in sensorimotor functions suggesting that the beneficial effects of EF could be observed at a lower dose when administered as liposomes through IN route.
Figure 5. Effect of optimized liposomal formulation on neurological severity score after I–R-induced cerebral injury in mice. Data were presented as mean ± SD and analyzed by one-way ANOVA followed by Tukey’s test as post hoc analysis. ap < .05 vs. sham group; bp < .05 vs. BCCAO + vehicle group; cp < .05 vs. BCCAO + BL group. EF: ethyl acetate fraction; BL: blank liposomes; LF: EF-loaded optimized liposomal formulation. The doses are in mg/kg.
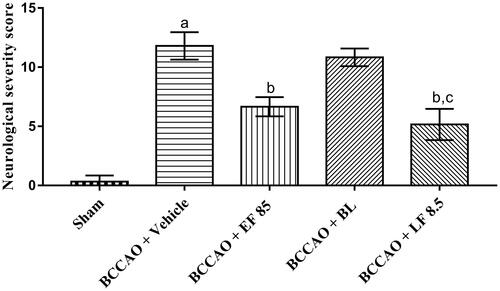
Effect of EF-L treatment on cerebral infarct size
I–R injury has been reported to induce neuronal cell death of diffused nature, spread throughout the brain [Citation42]. The extent of ischaemic cell death in the brain is established by infarct size estimation done using TTC staining [Citation56]. In the present study, cerebral infarct size was found to be higher in the vehicle-treated group in comparison with the sham group which indicated the neurodegenerative effects of BCCAO in animals. Treatment of animals with EF-L for 7 days through IN route showed a marked reduction of cerebral infarct size in comparison with vehicle-treated animals suggesting cerebroprotective effects of EF (). Furthermore, no significant difference was observed between EF and EF-L treated groups which confirmed the marked efficacy of EF at a lower dose when administered as liposomal formulation through IN route.
Figure 6. Effect of optimized liposomal formulation on cerebral infarct size after I–R-induced cerebral injury in mice. Data were presented as mean ± SD and analyzed by one way ANOVA followed by Tukey’s test as post hoc analysis. ap < .05 vs. sham group; bp < .05 vs. BCCAO + vehicle group; cp < .05 vs. BCCAO + BL group. EF: ethyl acetate fraction; BL: blank liposomes; LF: EF-loaded optimized liposomal formulation. The doses are in mg/kg.
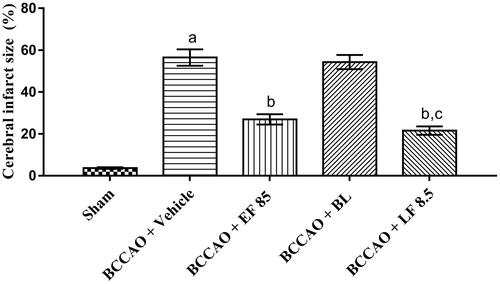
Effect of EF-L treatment on brain biochemical parameters
The pathophysiology of I–R-induced neuronal injury involves a series of complex processes of which oxidative stress has been identified as a major cause of cerebral damage [Citation57]. I–R injury is well documented to produce oxidative stress-mediated neurodegeneration by depleting endogenous antioxidants like reduced glutathione (GSH) and superoxide dismutase (SOD) activity and increasing lipid peroxidation [Citation45,Citation54,Citation58]. In line with these reports, the present study showed a significant decrease in GSH levels and SOD activity and increase in TBARS levels (index of lipid peroxidation) in the vehicle-treated group in comparison with the sham group. Treatment of animals with EF-L through IN route significantly ameliorated the BCCAO mediated decrease in GSH levels and SOD activity and increase in TBAR levels indicating the antioxidant effects of EF-L. Furthermore, animals treated with blank liposomes (BL) did not show a significant change in brain oxidative stress parameters in comparison with the vehicle-treated group, which confirmed that the antioxidant effects of EF-L were due to incorporated EF (). In addition, no significant difference was observed in brain biochemical parameters of EF and EF-L treated group, which showed that EF could exert marked antioxidant based neuroprotective effects at a lower dose when administered through IN route as a liposomal formulation.
Table 8. Effect of optimized liposomal formulation on brain biochemical parameters against I–R-induced cerebral injury.
Conclusions
In the current scenario pertaining to the management of stroke, rightful delivery of anti-stroke agents assumes great significance. Therefore, an efficient delivery system like liposome was prepared for ethyl acetate fraction (EF) obtained from A. cepa outer scale extract which demonstrated significant neuroprotective effects against I–R injury when administered intra-nasally at a dose one-tenth of the orally administered EF. After further pre-clinical and clinical studies, these liposomes may prove beneficial for the treatment of patients who have had an incident of global cerebral I–R injury as they facilitate neurorehabilitation and prevent neurodegeneration.
Disclosure statement
No potential conflict of interest was reported by the authors.
Additional information
Funding
References
- Ojaghihaghighi S, Vahdati SS, Mikaeilpour A, et al. Comparison of neurological clinical manifestation in patients with hemorrhagic and ischemic stroke. World J Emerg Med. 2017;8:34–38.
- Cheng NT, Kim AS. Intravenous thrombolysis for acute ischemic stroke within 3 hours versus between 3 and 4.5 hours of symptom onset. Neurohospitalist. 2015;5:101–109.
- Nour M, Scalzo F, Liebeskind DS. Ischemia-reperfusion injury in stroke. Interv Neurol. 2013;1:185–199.
- Uddin R, Kim HH, Lee JH, et al. Neuroprotective effects of medicinal plants. Excli J. 2013;12:541–545.
- Kumar S, Pandey AK. Chemistry and biological activities of flavonoids: an overview. Sci World J. 2013;2013:162750.
- Shri R, Bora KS. Neuroprotective effect of methanolic extracts of Allium cepa on ischemia and reperfusion-induced cerebral injury. Fitoterapia. 2008;79:86–96.
- Sakai Y, Murakami T, Yamamoto Y. Antihypertensive effects of onion on NO synthase inhibitor-induced hypertensive rats and spontaneously hypertensive rats. Biosci Biotechnol Biochem. 2003;67:1305–1311.
- Bystrická J, Musilová J, Vollmannová A, et al. Bioactive components of onion (Allium cepa L.) – a review. Acta Alimentaria. 2013;42:11–22.
- Arshad MS, Sohaib M, Nadeem M, et al. Status and trends of nutraceuticals from onion and onion by-products: a critical review. Cogent Food Agric. 2017;3:1280254.
- Shon MY, Choi SD, Kahng GG, et al. Antimutagenic, antioxidant and free radical scavenging activity of ethyl acetate extracts from white, yellow and red onions. Food Chem Toxicol. 2004;42:659–666.
- Thompson BJ, Ronaldson PT. Drug delivery to the ischemic brain. Adv Pharmacol. 2014;71:165–202.
- Costa PM, Wang JT, Morfin J, et al. Functionalised carbon nanotubes enhance brain delivery of amyloid-targeting pittsburgh compound b (pib)-derived ligands. Nanotheranostics. 2018;2:168–183.
- Vashist A, Kaushik A, Vashist A, et al. Recent trends on hydrogel based drug delivery systems for infectious diseases. Biomater Sci. 2016;4:1535–1553.
- Baklaushev VP, Nukolov NN, Khalansky AS, et al. Treatment of glioma by cisplatin-loaded nanogels conjugated with monoclonal antibodies against Cx43 and BSAT1. Drug Deliv. 2015;22:276–285.
- Li Y, He H, Jia X, et al. A dual-targeting nanocarrier based on poly(amidoamine) dendrimers conjugated with transferrin and tamoxifen for treating brain gliomas. Biomaterials. 2012;33:3899–3908.
- Gil ES, Li J, Xiao H, et al. Quaternary ammonium-cyclodextrin nanoparticles for enhancing doxorubicin permeability across the in vitro blood-brain barrier. Biomacromolecules. 2009;10:505–516.
- Bondì ML, Craparo EF, Giammona G, et al. Brain-targeted solid lipid nanoparticles containing riluzole: preparation, characterization and biodistribution. Nanomedicine. 2010;5:25–32.
- Ahmad N, Ahmad R, Naqvi AA, et al. Intranasal delivery of quercetin-loaded mucoadhesive nanoemulsion for treatment of cerebral ischaemia. Artif Cells Nanomed Biotechnol. 2018;46:717–729.
- Li X, Tsibouklis J, Weng T, et al. Nano carriers for drug transport across the blood-brain barrier. J Drug Target. 2017;25:17–28.
- Vieira DB, Gamarra LF. Getting into the brain: liposome-based strategies for effective drug delivery across the blood–brain barrier. Int J Nanomedicine. 2016;11:5381–5414.
- Pattni BS, Chupin VV, Torchilin VP. New developments in liposomal drug delivery. Chem Rev. 2015;115:10938–10966.
- Akbarzadeh A, Rezaei-Sadabady R, Davaran S, et al. Liposome: classification, preparation, and applications. Nanoscale Res Lett. 2013;8:102.
- Mignet N, Seguin J, Chabot GG. Bioavailability of polyphenol liposomes: a challenge ahead. Pharmaceutics. 2013;5:457–471.
- Fukuta T, Ishii T, Asai T, et al. Treatment of stroke with liposomal neuroprotective agents under cerebral ischemia conditions. Eur J Pharm Biopharm. 2015;97:1–7.
- Fukuta T, Asai T, Sato A, et al. Neuroprotection against cerebral ischemia/reperfusion injury by intravenous administration of liposomal fasudil. Int J Pharm. 2016;506:129–137.
- Ahmad H, Khandelwal K, Samuel SS, et al. Neuro-protective potential of a vesicular system of a standardized extract of a new chemotype of Withania somnifera Dunal (NMITLI118RT+) against cerebral stroke in rats. Drug Deliv. 2016;23:2630–2641.
- Lu CT, Zhao YZ, Wong HL, et al. Current approaches to enhance CNS delivery of drugs across the brain barriers. Int J Nanomedicine. 2014;9:2241–2257.
- Hanson LR, Frey WH. Intranasal delivery bypasses the blood-brain barrier to target therapeutic agents to the central nervous system and treat neurodegenerative disease. BMC Neurosci. 2008;9:S5.
- Tayebati SK, Nwankwo IE, Amenta F. Intranasal drug delivery to the central nervous system: present status and future outlook. Curr Pharm Des. 2013;19:510–526.
- Singh V. Development of a herbal formulation for the management of ischemia reperfusion induced cerebral injury [Unpublished dissertation]. Patiala (Punjab, India): Punjabi University; 2018.
- Utreja P, Jain S, Tiwary AK. Localized delivery of paclitaxel using elastic liposomes: formulation development and evaluation. Drug Deliv. 2011;18:367–376.
- Castangia I, Caddeo C, Manca ML, et al. Delivery of liquorice extract by liposomes and hyalurosomes to protect the skin against oxidative stress injuries. Carbohydr Polym. 2015;134:657–663.
- Washington C. Evaluation of the non-sink dialysis method for the measurement of drug release from colloids effects of drug partition. Int J Pharm. 1989;56:71–74.
- Sood S, Jain K, Gowthamarajan K. Optimization of curcumin nanoemulsion for intranasal delivery using design of experiment and its toxicity assessment. Colloids Surf B Biointerfaces. 2014;113:330–337.
- Singh V, Krishan P, Singh N, et al. Amelioration of ischemia-reperfusion induced functional and biochemical deficit in mice by Ocimum kilimandscharicum leaf extract. Biomed Pharmacother. 2017;85:556–563.
- Hanson LR, Fine JM, Svitak AL, et al. Intranasal administration of CNS therapeutics to awake mice. J Vis Exp. 2013;74:e4440.
- Morris R. Developments of a water-maze procedure for studying spatial learning in the rat. J Neurosci Methods. 1984;11:47–60.
- Lowry OH, Rosebrough NJ, Farr AL, et al. Protein measurement with the Folin phenol reagent. J Biol Chem. 1951;193:265–275.
- Beutler E, Duron O, Kelly BM. Improved method for the determination of blood glutathione. J Lab Clin Med. 1963;61:882–888.
- Kakkar P, Das B, Viswanathan PN. A modified spectrophotometric assay of superoxide dismutase. Indian J Biochem Biophys. 1984;21:130–132.
- Ohkawa H, Ohishi N, Yagi K. Assay for lipid peroxides in animal tissues by thiobarbituric acid reaction. Anal Biochem. 1979;95:351–358.
- Khan AR, Liu M, Khan MW, et al. Progress in brain targeting drug delivery system by nasal route. J Control Release. 2017;268:364–389.
- Heurtault B, Saulnier P, Pech B, et al. Physico-chemical stability of colloidal lipid particles. Biomaterials. 2003;24:4283–4300.
- Al Asmari AK, Ullah Z, Tariq M, et al. Preparation, characterization, and in vivo evaluation of intranasally administered liposomal formulation of donepezil. Drug Des Devel Ther. 2016;10:205–215.
- Aisha AF, Majid AM, Ismail Z. Preparation and characterization of nano liposomes of Orthosiphon stamineus ethanolic extract in soybean phospholipids. BMC Biotechnol. 2014;14:23.
- Zheng X, Shao X, Zhang C, et al. Intranasal H102 peptide-loaded liposomes for brain delivery to treat Alzheimer's disease. Pharm Res. 2015;32:3837–3849.
- Dash S, Murthy PN, Nath L, et al. Kinetic modeling on drug release from controlled drug delivery systems. Acta Pol Pharm. 2010;67:217–223.
- Costa P, Lobo JMS. Modeling and comparison of dissolution profiles. Eur J Pharm Sci. 2001;13:123–133.
- Neumar RW. Molecular mechanisms of ischemic neuronal injury. Ann Emerg Med. 2000;36:483–506.
- Pulsinelli WA, Brierley JB, Plum F. Temporal profile of neuronal damage in a model of transient forebrain ischemia. Ann Neurol. 1982;11:491–498.
- Auer RN, Jensen ML, Whishaw IQ. Neurobehavioral deficit due to ischemic brain damage limited to half of the CA1 sector of the hippocampus. J Neurosci. 1989;9:1641–1647.
- Kiryk A, Pluta R, Figiel I, et al. Transient brain ischemia due to cardiac arrest causes irreversible long-lasting cognitive injury. Behav Brain Res. 2011;219:1–7.
- Vorhees CV, Williams MT. Morris water maze: procedures for assessing spatial and related forms of learning and memory. Nat Protoc. 2006;1:848–858.
- Surapaneni S, Prakash T, Ansari M, et al. Study on cerebroprotective actions of Clerodendron glandulosum leaves extract against long term bilateral common carotid artery occlusion in rats. Biomed Pharmacother. 2016;80:87–94.
- Rogers DC, Campbell CA, Stretton JL, et al. Correlation between motor impairment and infarct volume after permanent and transient middle cerebral artery occlusion in the rat. Stroke. 1997;28:2060–2066.
- Joshi CN, Jain SK, Murthy PS. An optimized triphenyltetrazolium chloride method for identification of cerebral infarcts. Brain Res Brain Res Protoc. 2004;13:11–17.
- Chen H, Yoshioka H, Kim GS, et al. Oxidative stress in ischemic brain damage: mechanisms of cell death and potential molecular targets for neuroprotection. Antioxid Redox Signal. 2011;14:1505–1517.
- Murin R, Drgova A, Kaplan P, et al. Ischemia/reperfusion-induced oxidative stress causes structural changes of brain membrane proteins and lipids. Gen Physiol Biophys. 2001;20:431–438.