Abstract
The hierarchical microtextured/nanotextured topographies have been recognized to have better tissue integration properties, but the underlying mechanisms are only partially understood. Hedgehog signaling plays a pivotal role in developmental and homeostatic angiogenesis. We suppose that the Hedgehog-Gli1 signaling may play a significant role in the response of endothelial cells to microtextured/nanotextured topographies (MNTs). To confirm this hypothesis, we produced the MNTs decorated with TiO2 nanotubes of two different diameters (25 and 70 nm), and the proliferation, apoptosis, angiogenesis-related genes expression and Hedgehog signaling activity of human umbilical vein endothelial cells (HUVECs) grown onto these MNTs were measured. Our results showed that the MNTs induced significantly high expression of Sonic Hedgehog (SHH), Smoothened (SMO) and GLI1 in the HUVECs as well as high activation of Hedgehog-Gli1 signaling, compared to the smooth surface. The HUVECs grown on the MNTs showed significantly high levels of adhesion, proliferation and expression of angiogenesis-related genes, including angiopoietin-1 (ANG-1), vascular endothelial growth factor (VEGFA), vascular endothelial growth factor receptor 2 (VEGFR2) and endothelial nitric oxide synthase (ENOS); these enhancements were attenuated by siRNA-mediated depletion of SMO, which indicated a significant role of Hedgehog-Gli1 signaling in mediating the enhanced effect of the MNTs on the angiogenic potential of HUVECs. This study may contribute to the modification of biomaterial surfaces for better tissue integration and clinical performance.
Introduction
One of the key challenges in reestablishing bone is finding surface treatment technologies for an implant that can promote angiogenesis and bone revascularization by supplying oxygen, nutrients and regulatory factors to cells in bone tissue and removing metabolites. Bone revascularization in the host tissues surrounding a functionalized implant plays a pivotal role in promoting bone reconstruction and regeneration, which largely determine the successful bio-integration of dental implants or bone grafts [Citation1,Citation2]. Endothelial cells are important in these processes, forming new blood vessels via migration, proliferation and differentiation and providing a network of scaffolds between the bone and adjacent tissues. Increasing evidence has demonstrated that the surface properties of an implant greatly influence the extent of bone-implant integration [Citation3,Citation4]. The addition of micro-/nanotextured topographies (MNTs) to a biomedical implant surface can effectively promote endothelial proliferation and angiogenesis, which are crucial in improving the successful integration of implants both in vitro and in vivo [Citation5–7]. However, the precise mechanisms by which MNTs affect the physiology of vascular endothelial cells are not well understood, and this makes it difficult to optimize biomaterial topographies systematically.
The Hedgehog signaling pathway, which plays a crucial role in osteoblast function and bone healing, is also involved in developmental and homeostatic angiogenesis [Citation8–10]. The Hedgehog pathway signals via binding of three secreted Hedgehog proteins, namely, Sonic, Indian and Desert Hedgehog (SHH, IHH and DHH, respectively), to the 7-transmembrane proteins Smoothened (SMO) and Patched (PATCH), resulting in transcription of GLI-target genes [Citation11]. A recent study showed that SHH activated phosphoinositide 3-kinase signaling in mature endothelial cells and promoted capillary morphogenesis by umbilical vein endothelial cells [Citation12]. Pola et al. demonstrated that the SHH pathway promoted endothelial angiogenesis by stimulating expression of several pro-angiogenic growth factors in a GLI-dependent transcriptional manner [Citation13]. Another study indicated a significant role played by Hedgehog signaling in osteogenesis following stress fracture healing, which mediated its effects via modulation of bone formation and angiogenesis directly [Citation14]. In addition, knockout of SMO in mice resulted in severe disruption of vasculogenesis [Citation15], and the developing lungs of SHH-deficient mice lack proper vascularization [Citation16]. These lines of evidence indicated a critical role played by the Hedgehog pathway in the process of endothelial angiogenesis and vasculogenesis.
Increasing evidence has demonstrated that a series of signaling pathways are closely involved in the biological effect of topographical cues on endothelial cell behaviour [Citation17–19]. A recent study by Schernthaner et al. revealed that a significant modification of β-catenin signaling and target gene expression induced by micro-/nanotextured substrates was involved in the enhanced proliferation and phenotype of human microvascular endothelial cells [Citation18]. Hung et al. indicated that the effect of a polyurethane-gold nanocomposite on enhanced migration and proliferation of endothelial cells was mediated by activation of the FAK and PI3K/Akt signaling pathways [Citation19]. With regard to the Hedgehog signaling pathway, it has been shown to be highly sensitive to different surface topographies of biomaterials [Citation20–22]. Our recent study also demonstrated that the activation of Hedgehog-Gli1 signal transduction induced by hierarchical micro-/nanotextured topographies is involved in enhanced osteoblast proliferation and differentiation [Citation23]. Thus, it appeared reasonable for us to speculate that the Hedgehog-Gli1 signaling pathway might also play a potentially important role in mediating the response of endothelial cells to micro-/nanotextured topographical cues.
To test this hypothesis, we produced hierarchical MNTs decorated with TiO2 nanotubes by a simple and rapid method of acid-etching and anodization; then, human umbilical vein endothelial cells (HUVECs) were cultured on the MNTs to evaluate the cell adhesion, proliferation, apoptosis, angiogenesis-related genes expression and Hedgehog-Gli1 signaling activities. Furthermore, we silenced SMO, a key transmembrane conductance regulator of Hedgehog signaling, by transfection with SMO-specific siRNA to assess whether Hedgehog-Gli1 signaling was involved in the supposed downstream events including cell adhesion, proliferation, apoptosis and angiogenesis-related genes expression on MNTs.
Methods
Titanium disk preparation and surface characterization
Pure titanium discs (99.9%, 10 × 10 × 1 mm3) purchased from Mengtai Institute for Metal Materials Research (Beijing, China) were cut into titanium discs with 21 mm in diameter to fit snugly into 12-well plates. After polishing with silicon carbide sandpaper from 280 to 1000 grits and ultrasonic cleaning, the titanium disk was treated with 1 wt% hydrofluoric (HF) acid for 15 min, and then gently washed with ddH2O and dried at the room temperature. After acid-etched, the specimen was then anodized in an electrolyte containing 5 vol% of ddH2O and 95 vol% of ethylene glycol supplemented with 0.5 wt% of NH4F via a DC power supply with a platinum electrode as the cathode at 10 and 20 V to produce two typical MNTs (R10 and R20), respectively. The polished smooth titanium disc (S) without acid-etched/anodized was set as the control. The morphology and surface roughness of the specimens were inspected via using a field emission scanning electron microscopy (FE-SEM; S-4800; Hitachi, Japan) and Micro-XAM-3D noncontact surface profiler (ADE Corporation, Westwood, MA, USA) according to our previous study [Citation23]. Before cell culturing, the specimens were sterilized by cobalt-60 irradiation.
Cell culture and transfection
Human umbilical vein endothelial cells (HUVEC) were obtained from a commercial source (Shanghai Institute of Cell Biology, Shanghai, China), and were grown onto the three different surface topographies of titanium disks placed in a 12-well polystyrene plate at the density of 5 × 104/well. Cells were cultured in Dulbecco’s Modified Eagle’s Medium (Thermo Fisher Scientific, Waltham, MA, USA) contained with 10% fetal bovine serum (Thermo Fisher Scientific) and 1% penicillin/streptomycin (HyClone, Logan, UT, USA) in an incubator of 5% carbon dioxide at 37 °C. Four interference vectors LV-shRNA-GP specific to SMO were obtained from Longqian Biotech (Shanghai, China) containing the target sequences as follows: LV-shRNA-SMO 1 (SMO siRNA 1), 5′-GCGTCATCATCTTTGTCATCG-3′; LV-shRNA-SMO 2 (SMO siRNA 2), 5′-GCTACAAGAACTACCGATACC-3′; LV-shRNA-SMO 3 (SMO siRNA 3), 5′-GCTTTGTGCTCATTACCTTCA-3′; LV-shRNA-SMO 4 (SMO siRNA 4), 5′-GCTGCCACTTCTACGACTTCT-3′. The LV-shRNA-NC containing the sequence (5′-CAACAAGATGAAGAGCACCAA-3′) was set as the negative control that did not target any known genes. These LV-shRNA-GP vectors were transfected into HUVECs via using Lipofectamine 2000 (Invitrogen, USA) following the manufacturer’s instructions. After transfection for 48 h, western blot was performed to measure the protein levels of SMO in order to determine the efficiency of target gene silencing.
Cell adhesion assay
The negative control (NC) and SMO silencing (SMOsi) HUVECs were cultured onto the three different substrates of titanium discs with a density of 5 × 104/well. The attachment of cells was initially assessed by measuring the amount of cells attached to the titanium substrates (400 × over an area of 1020 × 800 μm2) under a fluorescence microscope after 1-h of incubation. Briefly, the non-adherent cells were dislodged from the titanium substrates by gently washing with phosphate-buffered saline (PBS) solution. After 30 min of fixation with 4% paraformaldehyde, the adhered cells were rinsed with PBS for three times and their nuclei was then stained with 4',6-diamidino-2-phenylindole (DAPI; Beyotime, Shanghai, China) for 5 min, ultimately seen under a microscope.
Cell proliferation assay
HUVECs were seeded onto the three different substrates of titanium discs at a density of 5 × 104/well for 3 and 5 days of incubation to evaluate the proliferation activities of cells using 3-(4,5-dimethylthiazol-2yl)-2,5-diphenyltetrazolium bromide (MTT; Sigma-Aldrich, St Louis, MO, USA) method. The NC and SMOsi HUVECs were also seeded onto the different titanium substrates with a density of 5 × 104/well for a total period of 5 days. At each prescribed time point, the MTT assay was conducted following the manufacturer’s protocol, and the amount of formazan product was measured by a spectrophotometer at 490 nm.
Annexin V apoptosis assay
The cell culture was the same as the cell proliferation assay. The apoptosis of HUVECs induced by the different titanium surfaces after 5 days of incubation was detected via using the ANXA5/AnnexinV-FITC Apoptosis Detection Kit (Beyotime institute of biotechnology, Shanghai, China) following the manufacturer’s instructions. Briefly, cells were collected and washed with PBS twice and resuspended in 195 μL binding buffer. Then, 10 μL propidium iodide (PI) and 5 μL ANXA5-FITC stock solution were added for 15 min of incubation at 37 °C, protected from light. Then, the apoptosis of cells was immediately analyzed by FACS.
Quantitative real-time PCR (RT-PCR)
The cell culture was the same as the cell proliferation assay. For quantitative RT-PCR analysis of the mRNA expressions of SHH, SMO, Gli1, angiopoietin-1 (ANG-1), vascular endothelial growth factor (VEGFA), vascular endothelial growth factor receptor 2 (VEGFR2), basic fibroblast growth factor (BFGF), endothelial nitric oxide synthase (ENOS), von Willebrand factor (VWF) and platelet-endothelial cell adhesion molecule 1 (PECAM-1) genes, TRIzol reagent (Sangon Biotech, Shanghai, China) was used to isolate total RNA from HUVECs. Then, a First Strand cDNA Synthesis Kit (Thermo Fisher Scientific) was conducted to convert the RNA into cDNA. Quantitative RT-PCR was performed to calculate the expressions of these genes using the SYBR Green RT-PCR Kit (Takara). The human GAPDH gene was set as a control in the quantitative RT-PCR analysis. The primers sequences used in this study were designed by Sangon Biotech with Primer Premier 5.0 program (Applied Biosystems) as presented in . Amplification was performed in a LightCycler480 sequence detector system (Roche Applied Science, Laval, Quebec, Canada) with 40 cycles of: 5 s at 95 °C, 20 s at 58 °C and 1 min at 72 °C. The mRNA expressions of these genes were calculate using the 2−△△CT method with values normalized to GAPDH expression.
Table 1. Primer sequences used in quantitative real-time PCR.
Western blot analysis
The NC and SMOsi HUVECs were seeded onto the three different substrates of titanium discs with a density of 5 × 104/well and cultured for 5 days. Cells were collected and lysed in RIPA lysis buffer for the total proteins extraction. After separated by 10% sodium dodecyl sulfate-polyacrylamide gel electrophoresis (SDS-PAGE), the proteins were then transferred into a polyvinylidene difluoride (PVDF) membrane (Millipore, Bedford, MA, USA). Immunoblotting was performed overnight at 4 °C by using antibodies as follows: anti-Shh (ab53281; Abcam, Cambridge, UK), anti-Smo (sc-13943; Santa Cruz Biotechnology, Santa Cruz, CA, USA), anti-Gli1 (#2534; CST, Danvers, MA, USA) and anti-β-actin antibody (Santa Cruz Biotechnology). After incubation with the HRP-linked secondary antibody, blots were visualized using an enhanced chemiluminescence (ECL) detection kit (Millipore) according to the manufacturer’s instructions.
Statistical analyses
Statistical analysis was conducted by using SPSS version 19.0 (IBM, NY, USA) and GraphPad Prism 4.0 (GraphPad Software Inc., San Diego, CA, USA). The measurement data was presented as the mean ± standard error of the mean (SEM) from at least three independent experiments. Differences between groups were analyzed by one-way analysis of variance combined with Student–Newman–Keuls post hoc test, or Student’s t-test. A p values of less than .05 was considered statistically significant.
Results
Surface characterization of MNTs
FE-SEM characterization of the different titanium surfaces is presented in . The titanium discs were 21 mm in diameter and 1 mm in thickness. Low-magnification images showed that the smooth surface was relatively flat while MNTs appeared as 10–40 μm pits and 1–5 μm micropits. At high magnification, two different classes of nanotube with 25 and 70 nm diameters were uniformly distributed on the micron-sized surface. Micro-XAM-3D micrographs with two scanning areas of 1.8 × 1.4 mm and 450 × 350 μm and the surface roughness parameters of the samples are illustrated in . The MNTs exhibited significantly higher Ra and Rq than the smooth surface (all p < .001). Nevertheless, no significant difference was found in these two parameters between the R10 and R20 groups.
Figure 1. Representative FE-SEM pictures showing the morphology of the smooth (S) and hierarchical micro-/nanotextured surfaces (R10 and R20). (A) General observation of the fabricated samples; (B) The nanotubes with 25 nm and 70 nm in diameter were distributed relatively uniformly onto the microstructured surface.
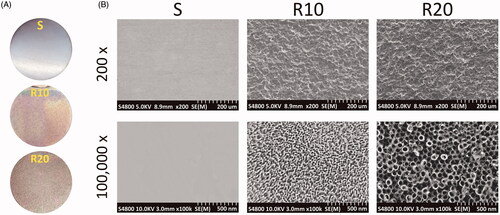
Figure 2. Surface roughness analysis of the fabricated samples. (A) Representative pictures of the smooth (S) and hierarchical micro-/nanotextured surfaces (R10 and R20) taken by a Micro-XAM-3D noncontact surface profiler. The scanning areas were 1.8 mm ×1.4 mm and 450 μm × 350 μm, respectively; (B) Comparisons of the roughness parameter (Ra, the average roughness; Rq, root mean square roughness) of different surfaces. *p < .05; **p < .01; ***p < .001.
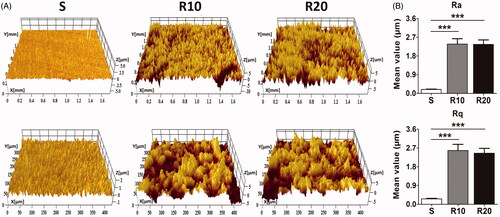
Initial adhesion of HUVECs on MNTs
The extent of initial cell adhesion onto the different titanium surfaces was assessed by measuring the number of cell nuclei stained with DAPI after 1 h of culturing. As shown in , the MNTs (R10 and R20) exhibited a significantly higher number of adherent cells than the smooth surface (p < .05). The cell number on the surface of the R10 group was similar to that of the R20 group without a significant difference (p > .05).
Figure 3. Effect of MNTs on the early adhesion ability of HUVECs. (A) The number of HUVECs nuclei stained by DAPI were evaluated under a fluorescence microscope onto the smooth (S) and hierarchical micro-/nanotextured surfaces (R10 and R20); (B) Comparisons of the adherent cell numbers on the smooth and hierarchical micro-/nanotextured surfaces (counts performed over an area of 1020 × 800 μm2). *p < .05; **p < .01; ***p < .001.
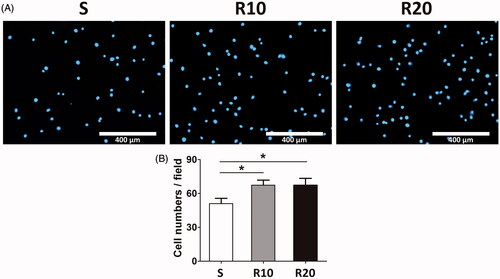
The proliferation and apoptosis of HUVECs on MNTs
To evaluate the effect of MNTs on the proliferation and apoptosis of HUVECs, cells were cultured onto the three different titanium surfaces for 3 and 5 days, and then MTT and Annexin V apoptosis assays were performed. Our results showed that the proliferation of HUVECs increased in all three groups as the cultivation time progressed (). The cells cultured onto the MNTs displayed significantly higher activity of proliferation than the smooth group at both time points (p < .05). In contrast, the smooth titanium surface induced significantly higher apoptosis rates of HUVECs than the MNTs (p < .05, ). No statistically significant differences in cell proliferation and apoptosis were found between the R10 and R20 groups (Both p > .05).
Figure 4. Effects of the MNTs on the proliferation and apoptosis of HUVECs. (A, B) The proliferation of HUVECs seeded onto the smooth (S) and hierarchical micro-/nanotextured surfaces (R10 and R20) for 3 and 5 days was detected by using a MTT assay; (C, D) Evaluation of apoptosis of HUVECs seeded onto the S, R10 and R20 surfaces for 5 days by using annexin-V- FITC/PI staining and fow cytometry. *p < .05; **p < .01; ***p < .001.
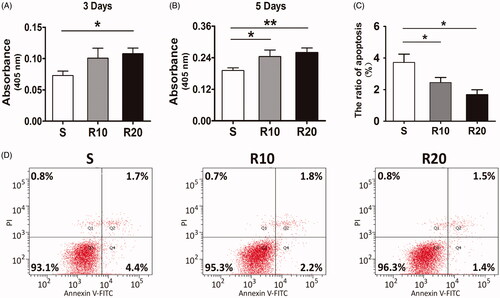
Expression of angiogenesis-associated genes in HUVECs on MNTs
Angiogenesis-associated gene expression, including that of ANG-1, VEGFA, VEGFR2, BFGF, ENOS, VWF and PECAM-1, was measured to evaluate the effect of MNTs on the angiogenic potential of HUVECs. As presented in , the expression of VEGFA, ENOS and PECAM-1 was significantly upregulated in HUVECs grown on the MNTs compared to that in cells grown on the smooth surface at 3 and 5 days of incubation, with the R20 group showing the highest levels of expression. With regard to ANG-1 and VEGRF2, significantly increased expression induced by MNTs was found only at day 5. No significant differences in the expression levels of BFGF and VWF were found among the three different titanium surfaces. Taken together, the MNTs effectively promote angiogenesis-related genes expression in HUVECs, and R20 induced the highest.
Figure 5. Effect of MNTs on the expression of angiogenic-associated genes. (A) ANG-1, (B) VEGFA, (C) VEGFR2, (D) BFGF, (E) ENOS, (F) VWF and (G) PECAM-1 expression levels in HUVECs seeded onto the smooth (S) and hierarchical micro-/nanotextured surfaces (R10 and R20) for 3 and 5 days were measured by quantitative real-time PCR. *p < .05; **p < .01; ***p < .001.
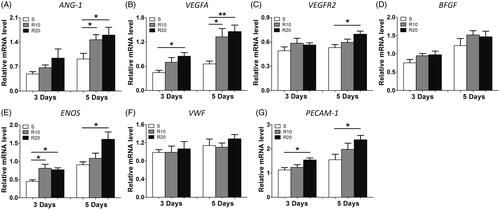
The activity of Hedgehog-Gli1 signaling in HUVECs on MNTs
Gene expression of SHH, SMO and Gli1 were measured by quantitative RT-PCR analysis to examine the effect of MNTs on the activation of Hedgehog-Gli1 signaling. As presented in , the mRNA expression levels of SHH, SMO and GLI1 increased over time in cells on the three different titanium surfaces. On day 3, no significant difference in the mRNA expression of SHH was observed among the samples; however, HUVECs cultured onto the MNTs had significantly higher SHH levels than cells cultured onto the S surface at 5 days. The mRNA expression levels of SMO and Gli1 were significantly increased by the MNTs on days 3 and 5, especially R20. To further validate the results above, Western blot analysis was performed to detect the levels of these proteins involved in Hedgehog-Gli1 signaling after incubation for 5 days. The R20 significantly induced a higher protein level of SHH than did the S group (). With regard to SMO, the protein level was dramatically upregulated by MNTs, especially R20 (). In addition, a similar trend was observed from the protein level of Gli1 (). Overall, the MNTs activated Hedgehog-Gli1 signaling and R20 had the greatest effect.
Figure 6. Hedgehog signaling activation in HUVECs onto the MNTs. The mRNA expression of SHH (A), SMO (B) and Gli1 (C) in HUVECs seeded onto the smooth (S) and micro-/nanotextured surfaces (R10 and R20) for 3 and 5 days were measured by quantitative real-time PCR; Western blot analysis of SHH (D), SMO (E) and Gli1 (F) protein levels in HUVECs after 5 days of incubation onto the S, R10 and R20 surfaces. *p < .05; **p < .01; ***p < .001.
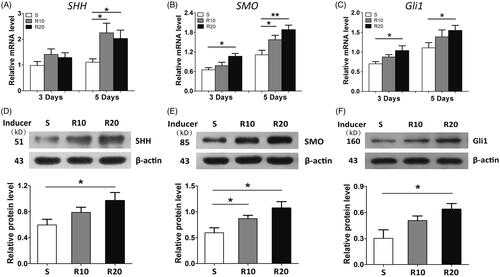
Role of Hedgehog-Gli1 signaling in adhesion, proliferation and apoptosis of HUVECs on MNTs
We downregulated the expression of SMO, a key transmembrane conductance regulator of Hedgehog-Gli1 signaling, by transfection with SMO-specific siRNA (LV-shRNA-SMO) to assess whether Hedgehog-Gli1 signaling promotes cell adhesion, proliferation and inhibition of apoptosis by MNTs. The siRNA-depletion efficiency was calculated by a Western blot assay after incubation for 2 days. As shown in , LV-shRNA-SMO 4 successfully decreased the highest protein levels of SMO by 64.5%, and it was used to silence gene expression of SMO for the following experiments. The initial adhesion of HUVECs was obviously influenced by surface properties, while it was not significantly affected by SMOsi (). SMO silencing by transfection with LV-shRNA-SMO4 obviously decreased the proliferation activities of HUVECs after 5 days of incubation, especially the cells cultured onto the MNTs (). In contrast, SMOsi resulted in promoting apoptosis of HUVECs grown on the MNTs to a similar level as those grown on the smooth titanium surface (). These results indicate a role for Hedgehog-Gli1 signaling in mediating the enhanced proliferation and inhibited apoptosis of HUVECs by MNTs.
Figure 7. Transfection and knockdown efficiency of SMO-specific siRNA (LV-shRNA-SMO) in HUVECs. (A) HUVECs under light microscope and fluorescence microscope after transfection (×100); (B) HUVECs were transfected with LV-shRNA specifically targeting SMO or LV-shRNA-NC, and siRNA-depletion efficiency was calculated by Western blot assay. *p < .05; **p < .01; ***p < .001.
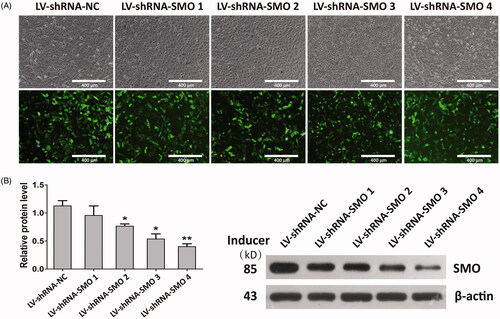
Figure 8. Effect of SMO silencing on the early adhesion ability of HUVECs seeded onto the smooth (S) and hierarchical micro-/nanotextured surfaces (R10 and R20). (A) The number of NC and SMOsi HUVECs nuclei stained by DAPI were evaluated under a fluorescence microscope onto the S, R10 and R20 surfaces; (B) Comparisons of the adherent cell numbers on the S, R10 and R20 surfaces (counts performed over an area of 1020 × 800 μm2). *p < .05; **p < .01; ***p < .001.
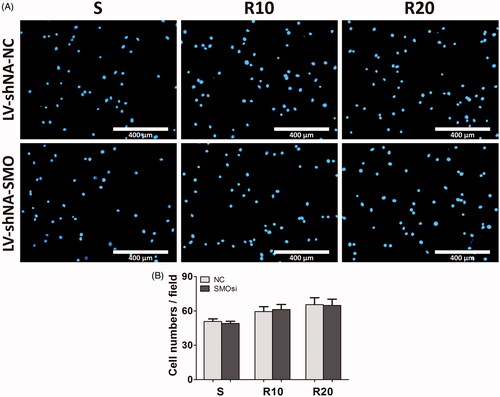
Figure 9. Effect of SMO silencing on the proliferation and apoptosis of HUVECs seeded onto the smooth (S) and hierarchical micro-/nanotextured surfaces (R10 and R20). (A) The proliferation of NC and SMOsi HUVECs seeded onto the S, R10 and R20 surfaces after 5 days of incubation was calculated by MTT assay; (B, C) Evaluation of apoptosis of NC and SMOsi HUVECs cultured onto the samples for 5 days by using annexin-V- FITC/PI staining and fow cytometry. *p < .05; **p < .01; ***p < .001.
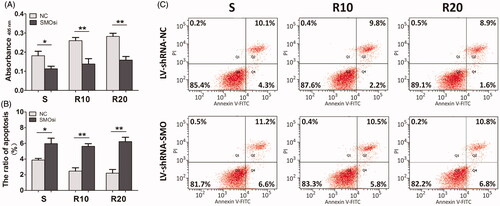
Role of Hedgehog-Gli1 signaling in HUVEC angiogenesis on MNTs
We further evaluated the effect of SMOsi on the expression of angiogenesis-related genes in HUVECs seeded onto the smooth (S) and hierarchical micro-/nanotextured surfaces (R10 and R20) after 5 days of incubation. As presented in , SMOsi significantly decreased the expression levels of ANG-1 and VEGFA genes on the MNTs, while not on the smooth titanium surface. With regard to the VEGFR2 and ENOS genes, significant downregulation by SMOsi was found only on R20 and not on S or R10. However, the expression levels of BFGF, VWF and PECAM-1 were not affected by SMOsi on the three different titanium surfaces. In general, Hedgehog-Gli1 signaling mediated the enhanced expression of angiogenesis-related genes in HUVECs enabled by the MNTs, which suggests a significant role for Hedgehog-Gli1 signaling in the effect of MNTs on the angiogenic potential of HUVECs.
Figure 10. Effect of SMO silencing on the expression of angiogenic-associated genes in HUVECs seeded onto the smooth (S) and hierarchical micro-/nanotextured surfaces (R10 and R20) after 5 days of incubation. (A) ANG-1, (B) VEGFA, (C) VEGFR2, (D) BFGF, (E) ENOS, (F) VWF and (G) PECAM-1 expression levels in NC and SMOsi HUVECs cultured onto the S, R10 and R20 surfaces were detected by quantitative real-time PCR. *p < .05; **p < .01; ***p < .001.
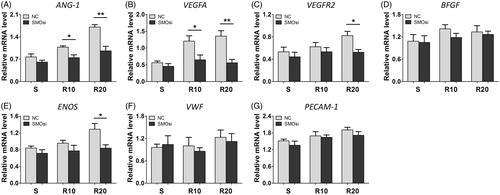
Discussion
Previous studies have demonstrated that hierarchical micro-/nanotextured topographies (MNTs) play a significant role in endothelial cell recognition of bioactive materials and biological micro-environments used for intravascular stent, bone graft and dental implantation [Citation5–7]. However, the underlying mechanism involved in the response of endothelial cells to surface topographies remains unclear. In this study, we found that MNTs promoted HUVEC angiogenesis in terms of enhancing adhesion and proliferation of HUVECs, inhibiting cell apoptosis and upregulating the expression of angiogenesis-associated genes. This enhanced effect was related to the upregulation of SHH, SMO and Gli1 expression. SMO silencing significantly attenuated the activation of Hedgehog-Gli1 signaling and the consequent enhanced events of cell proliferation, antiapoptosis and angiogenesis by MNTs, indicating a crucial role for Hedgehog-Gli1 signaling involved in the biological effects of MNTs on the angiogenic potential of HUVECs.
Highly efficient and rapid bone vascularization is essential for optimal bio-integration of dental implants or bone grafts [Citation24,Citation25]. Recently, various nano- and microstructured surface modification techniques have been developed to achieve desirable biological integration of bone implants and better clinical performance [Citation26,Citation27]. Several lines of evidence have demonstrated that the addition of MNTs to a biomedical implant surface contribute to promoting adhesion, proliferation and viability of endothelial cells [Citation6,Citation28,Citation29]. Consistently, our simple and rapid method of acid-etched and anodization successfully produced MNTs decorated with two different TiO2 nanotubes (25 and 70 nm in diameter); the HUVECs grown onto these MNTs showed significantly higher activities of adhesion and proliferation, and lower levels of apoptosis. Furthermore, the expression of several angiogenesis-related genes, including VEGFA, VEGFR2, ANG-1, BFGF, PECAM-1, VWF and ENOS, was measured to evaluate the effect of MNTs on the angiogenic differentiation of HUVECs. VEGFA, its receptor VEGFR2, ANG-1, BFGF and PECAM-1 have been identified as crucial factors in angiogenesis regulation by promoting endothelial cell functions such as migration, proliferation and tube-forming activity [Citation30–33]. VWF and ENOS are functional markers for endothelial cells and have essential roles in modulation of endothelial cell growth and angiogenesis [Citation32,Citation33]. Our data showed that the expression of the angiogenesis- related genes ANG-1, VEGFA, VEGRF2, ENOS and PECAM-1 were significantly increased in HUVECs grown on the MNTs, especially R20, which was consistent with previous studies. Santos et al. have reported that HUVECs grown on microtextured/nanotextured scaffolds exhibited significantly higher expression of vimentin and PECAM-1 and a marked angiogenic potential [Citation34]. Another study indicated that TiO2 nanofibrous topographies induced significantly higher expression of various angiogenesis-related genes and enhanced formation of capillary-like tube networks of HUVECs [Citation35]. Thus, this presently available evidence demonstrates that MNTs effectively promote the angiogenic potential of HUVECs, with R20 the most effective.
Surface modification of biomaterials not only modulates cell behaviour directly by regulating protein adsorption and cell focal adhesion but also influences the cell phenotype and overall biological response to the implant indirectly via altering the intracellular signaling pathways [Citation36,Citation37]. Hedgehog signaling plays a pivotal role in both angiogenesis and osteogenesis [Citation8–10]. In this respect, Hedgehog-Gli1 signaling has become focused in biomaterial studies and has been observed to be involved in the biological effects of biomaterial topographies [Citation20–22]. The authors have shown previously that Hedgehog-Gli1 signaling in MG63 cells grown onto our MNTs decorated with TiO2 nanotubes was markedly activated and that it mediated enhanced cell proliferation and osteoblastic differentiation [Citation23]. Thus, in this study these MNTs were further evaluated to determine whether Hedgehog-Gli1 signaling was involved in the response of endothelial cells to the surface topographies. The expression levels of SHH, SMO and Gli1 were significantly increased in HUVECs grown onto the MNTs, which validated the activation of Hedgehog-Gli1 signaling by the MNTs. These MNTs may provide cells with an extracellular stimulus that can change the structures of the interphase chromosomes inside the nucleus via passing through the cytoskeletal components to the nucleoskeleton, subsequently leading to differences in the gene expression of Hedgehog-Gli1 signaling [Citation38,Citation39]. However, the specific underlying mechanisms need to be further investigated.
Hedgehog-Gli1 signaling has been reported to have pivotal effects on endothelial cell viability and apoptosis, which are essential for endothelial tube formation during bone vascularization [Citation40,Citation41]. Asai et al. have shown that SHH directly promotes the migration, adhesion, proliferation and even tube formation of endothelial progenitor cells [Citation42]. Other studies have indicated that SHH application protects against apoptosis in endothelial cells, while the SMO-specific inhibitor enhances TNF-α-induced apoptosis in HUVECs [Citation43,Citation44]. Regarding Hedgehog-Gli1 signaling activation in HUVECs induced by the MNTs, we paid further attention to Hedgehog-Gli1 signaling and investigated its potential roles in mediating biological effects of the biomaterial topographies. Our data showed that SMO silencing significantly attenuated the enhanced proliferation and inhibited apoptosis by the MNTs, which elucidated a protective effect of Hedgehog-Gli1 signaling in endothelial cell viability and apoptosis on the MNTs. However, no significant difference in the cell adhesion of HUVECs was found on the S, R10 and R20 groups after silencing SMO.
Hedgehog signaling activates vasculogenesis not only directly by altering transcription of various angiogenesis-related genes but also indirectly by regulating several downstream pathways such as VEGF, Notch and BMP [Citation15,Citation45,Citation46]. For verification, the association between Hedgehog-Gli1 signaling and angiogenesis-related gene expression in HUVECs on biomaterial surfaces was further investigated. Our data showed that the upregulated expression of ANG-1, VEGFA, VEGFR2 and ENOS on the MNTs was significantly attenuated by SMOsi, which indicated that these angiogenesis-related genes might be located downstream of and regulated by Hedgehog-Gli1 signaling on the MNTs. These results were consistent with previous reports. A recent study showed that SHH application significantly upregulated the expression of several angiogenesis-related factors including ANG-1, VEGFA and FGF in cardiac microvascular endothelial cells, as well as the production of SMO, Patch-1 and GLI1 proteins in Hedgehog-Gli1 signaling [Citation44]. Other studies reported that VEGFA and ENOS were downstream targets of Hedgehog signaling [Citation47,Citation48]. The blockage of Hedgehog-Gli1 signaling via a specific inhibitor of SMO or a siRNA specific to Patched strongly downregulated the expression of ENOS, VEGFA, VEGFR2 and VWF [Citation49,Citation50]. However, the expression levels of BFGF, VWF and PECAM-1 were not significantly affected by SMOsi on the three different titanium surfaces. Overall, these results provided an explicit underlying mechanism that Hedgehog-Gli1 signaling was involved in mediating the enhanced effect of the MNTs on the proliferation, angiogenesis-related genes expression and angiogenic potential of HUVECs. More biological studies with other topographies are required to validate our tentative conclusions.
Conclusions
In the present study, a simple and rapid method of acid-etching and anodization produced the MNTs decorated with TiO2 nanotubes of two different diameters. HUVECs grown onto these MNTs showed significantly higher activities of adhesion and proliferation, expression levels of several angiogenesis-related genes and lower levels of apoptosis; these enhanced effects of MNTs on the in vitro angiogenic potential of HUVECs were mediated by Hedgehog-Gli1 signaling activation. Our results provide an advanced understanding about the role of Hedgehog-Gli1 signaling involved in the biological effects of surface topographies on bone vascularization and reconstruction, which may contribute to the modification of biomaterial surfaces with better tissue integration and clinical performance.
Disclosure statement
No potential conflict of interest was reported by the authors.
Additional information
Funding
References
- Zhang Y, Andrukhov O, Berner S, et al. Osteogenic properties of hydrophilic and hydrophobic titanium surfaces evaluated with osteoblast-like cells (MG63) in coculture with human umbilical vein endothelial cells (HUVEC). Dent Mater. 2010;26:1043–1051.
- Unger RE, Sartoris A, Peters K, et al. Tissue-like self-assembly in cocultures of endothelial cells and osteoblasts and the formation of microcapillary-like structures on three-dimensional porous biomaterials. Biomaterials. 2007;28:3965–3976.
- Park J, Bauer S, Schmuki P, et al. Narrow window in nanoscale dependent activation of endothelial cell growth and differentiation on TiO2 nanotube surfaces. Nano Lett. 2009;9:3157–3164.
- Saghiri MA, Asatourian A, Garcia-Godoy F, et al. The role of angiogenesis in implant dentistry part I: review of titanium alloys, surface characteristics and treatments. Med Oral. 2016;21:e.514–525.
- Greiner AM, Sales A, Chen H, et al. Nano- and microstructured materials for in vitro studies of the physiology of vascular cells. Beilstein J Nanotechnol. 2016;7:1620–1641.
- Moffa M, Sciancalepore AG, Passione LG, et al. Combined nano- and micro-scale topographic cues for engineered vascular constructs by electrospinning and imprinted micro-patterns. Small. 2014;10:2439–2450.
- Ranjan A, Webster TJ. Increased endothelial cell adhesion and elongation on micron-patterned nano-rough poly(dimethylsiloxane) films. Nanotechnology. 2009;20:305102.
- Yang J, Andre P, Ye L, et al. The Hedgehog signalling pathway in bone formation. Int J Oral Sci. 2015;7:73–79.
- Byrd N, Grabel L. Hedgehog signaling in murine vasculogenesis and angiogenesis. Trends Cardiovasc Med. 2004;14:308–313.
- Vokes SA, Yatskievych TA, Heimark RL, et al. Hedgehog signaling is essential for endothelial tube formation during vasculogenesis. Development. 2004;131:4371–4380.
- Varjosalo M, Taipale J. Hedgehog: functions and mechanisms. Genes Dev. 2008;22:2454–2472.
- Kanda S, Mochizuki Y, Suematsu T, et al. Sonic hedgehog induces capillary morphogenesis by endothelial cells through phosphoinositide 3-kinase. J Biol Chem. 2003;278:8244–8249.
- Pola R, Ling LE, Silver M, et al. The morphogen Sonic hedgehog is an indirect angiogenic agent upregulating two families of angiogenic growth factors. Nat Med. 2001;7:706–711.
- Kazmers NH, McKenzie JA, Shen TS, et al. Hedgehog signaling mediates woven bone formation and vascularization during stress fracture healing. Bone. 2015;81:524–532.
- Astorga J, Carlsson P. Hedgehog induction of murine vasculogenesis is mediated by Foxf1 and Bmp4. Development. 2007;134:3753–3761.
- Pepicelli CV, Lewis PM, McMahon AP. Sonic hedgehog regulates branching morphogenesis in the mammalian lung. Curr Biol. 1998;8:1083–1086.
- Schernthaner M, Leitinger G, Wolinski H, et al. Enhanced Ca(2+)entry and tyrosine phosphorylation mediate nanostructure-induced endothelial proliferation. J Nanomater. 2013;2013:1.
- Schernthaner M, Reisinger B, Wolinski H, et al. Nanopatterned polymer substrates promote endothelial proliferation by initiation of beta-catenin transcriptional signaling. Acta Biomater. 2012;8:2953–2962.
- Hung HS, Wu CC, Chien S, et al. The behavior of endothelial cells on polyurethane nanocomposites and the associated signaling pathways. Biomaterials. 2009;30:1502–1511.
- Kim SY, Yoo JY, Ohe JY, et al. Differential expression of osteo-modulatory molecules in periodontal ligament stem cells in response to modified titanium surfaces. Biomed Res Int. 2014;2014:1.
- Regard JB, Malhotra D, Gvozdenovic-Jeremic J, et al. Activation of Hedgehog signaling by loss of GNAS causes heterotopic ossification. Nat Med. 2013;19:1505–1512.
- Miron RJ, Shuang Y, Bosshardt DD, et al. Osteogenic gene array of osteoblasts cultured on a novel osteoinductive biphasic calcium phosphate bone grafting material. Clin Oral Invest. 2017;21:801–808.
- Lin Y, Huang Y, He J, et al. Role of Hedgehog-Gli1 signaling in the enhanced proliferation and differentiation of MG63 cells enabled by hierarchical micro-/nanotextured topography. Int J Nanomed. 2017;12:3267–3280.
- Ponche A, Bigerelle M, Anselme K. Relative influence of surface topography and surface chemistry on cell response to bone implant materials. Part 1: physico-chemical effects. Proc Inst Mech Eng H. 2010;224:1471–1486.
- Mercado-Pagan AE, Stahl AM, Shanjani Y, et al. Vascularization in bone tissue engineering constructs. Ann Biomed Eng. 2015;43:718–729.
- Nguyen LH, Annabi N, Nikkhah M, et al. Vascularized bone tissue engineering: approaches for potential improvement. Tissue Eng B Rev. 2012;18:363–382.
- Chen LJ, Kaji H. Modeling angiogenesis with micro- and nanotechnology. Lab Chip. 2017;17:4186–4219.
- Peng L, Eltgroth ML, LaTempa TJ, et al. The effect of TiO2 nanotubes on endothelial function and smooth muscle proliferation. Biomaterials. 2009;30:1268–1272.
- Holmes B, Bulusu K, Plesniak M, et al. A synergistic approach to the design, fabrication and evaluation of 3D printed micro and nano featured scaffolds for vascularized bone tissue repair. Nanotechnology. 2016;27:064001.
- Li J, Ai HJ. The responses of endothelial cells to Zr6 1Ti 2Cu25Al12 metallic glass in vitro and in vivo. Mater Sci Eng C Mater Biol Appl. 2014;40:189–196.
- Pavlov N, Frendo JL, Guibourdenche J, et al. Angiogenin expression during early human placental development; association with blood vessel formation. Biomed Res Int. 2014;2014:1.
- Muhammad R, Lim SH, Goh SH, et al. Sub-100 nm patterning of TiO2 film for the regulation of endothelial and smooth muscle cell functions. Biomater Sci. 2014;2:1740–1749.
- Mohan CC, Chennazhi KP, Menon D. In vitro hemocompatibility and vascular endothelial cell functionality on titania nanostructures under static and dynamic conditions for improved coronary stenting applications. Acta Biomater. 2013;9:9568–9577.
- Santos MI, Tuzlakoglu K, Fuchs S, et al. Endothelial cell colonization and angiogenic potential of combined nano- and micro-fibrous scaffolds for bone tissue engineering. Biomaterials. 2008;29:4306–4313.
- Tan AW, Liau LL, Chua KH, et al. Enhanced in vitro angiogenic behaviour of human umbilical vein endothelial cells on thermally oxidized TiO2 nanofibrous surfaces. Sci Rep. 2016;6:21828.
- Wang W, Liu Q, Zhang Y, et al. Involvement of ILK/ERK1/2 and ILK/p38 pathways in mediating the enhanced osteoblast differentiation by micro/nanotopography. Acta Biomater. 2014;10:3705–3715.
- Wang W, Zhao L, Wu K, et al. The role of integrin-linked kinase/beta-catenin pathway in the enhanced MG63 differentiation by micro/nano-textured topography. Biomaterials. 2013;34:631–640.
- Dalby MJ, Biggs MJ, Gadegaard N, et al. Nanotopographical stimulation of mechanotransduction and changes in interphase centromere positioning. J Cell Biochem. 2007;100:326–338.
- Dalby MJ, Gadegaard N, Herzyk P, et al. Nanomechanotransduction and interphase nuclear organization influence on genomic control. J Cell Biochem. 2007;102:1234–1244.
- Chinchilla P, Xiao L, Kazanietz MG, et al. Hedgehog proteins activate pro-angiogenic responses in endothelial cells through non-canonical signaling pathways. Cell Cycle. 2010;9:570–579.
- Soleti R, Lauret E, Andriantsitohaina R, et al. Internalization and induction of antioxidant messages by microvesicles contribute to the antiapoptotic effects on human endothelial cells. Free Radic Biol Med. 2012;53:2159–2170.
- Asai J, Takenaka H, Kusano KF, et al. Topical sonic hedgehog gene therapy accelerates wound healing in diabetes by enhancing endothelial progenitor cell-mediated microvascular remodeling. Circulation. 2006;113:2413–2424.
- Zhu SL, Luo MQ, Peng WX, et al. Sonic hedgehog signalling pathway regulates apoptosis through Smo protein in human umbilical vein endothelial cells. Rheumatology (Oxford). 2015;54:1093–1102.
- Guo W, Yi X, Ren F, et al. Activation of SHH signaling pathway promotes vasculogenesis in post-myocardial ischemic-reperfusion injury. Int J Clin Exp Pathol. 2015;8:12464–12472.
- Coultas L, Nieuwenhuis E, Anderson GA, et al. Hedgehog regulates distinct vascular patterning events through VEGF-dependent and -independent mechanisms. Blood. 2010;116:653–660.
- Lawson ND, Vogel AM, Weinstein BM. Sonic hedgehog and vascular endothelial growth factor act upstream of the Notch pathway during arterial endothelial differentiation. Dev Cell. 2002;3:127–136.
- Podlasek CA, Meroz CL, Korolis H, et al. Sonic hedgehog, the penis and erectile dysfunction: a review of sonic hedgehog signaling in the penis. Curr Pharm Des. 2005;11:4011–4027.
- Moran CM, Myers CT, Lewis CM, et al. Hedgehog regulates angiogenesis of intersegmental vessels through the VEGF signaling pathway. Dev Dyn. 2012;241:1034–1042.
- Agouni A, Mostefai HA, Porro C, et al. Sonic hedgehog carried by microparticles corrects endothelial injury through nitric oxide release. FASEB J. 2007;21:2735–2741.
- Zhao S, Zhang Z, Yao Z, et al. Tetramethylpyrazine attenuates sinusoidal angiogenesis via inhibition of hedgehog signaling in liver fibrosis. IUBMB Life. 2017;69:115–127.