Abstract
Good biological properties for titanium implants will shorten the treatment cycle and improve patient comfort, which are also the main goals of dentistry and orthopaedics. At present, the biological properties of titanium implants are mainly enhanced in two aspects: their surface chemistry and surface morphology. In this study, a surface modification strategy combining bioactive trace elements with surface micromorphology modification was used to enhance the biological properties of pure titanium. A new coating incorporating silicon micropore/microsphere topography was prepared on a titanium plate by micro-arc oxidation (MAO) technology. The properties of the coating and its effects on the adhesion and osteogenic differentiation of rat bone marrow mesenchymal stem cells (BMSCs) were further analyzed. The experimental results show that a coating doped with amorphous silicon with micropore/microsphere topography was incorporated onto the titanium surface and the surface roughness in the treated groups was obviously higher than that in the Ti group. In vitro, the presence of a silicon-incorporating coating with a micropore/microsphere topography on the titanium surface significantly enhanced the initial adhesion, proliferation and osteogenic differentiation of BMSCs. These results indicate that the silicon-incorporating coating with micropore/microsphere topography has potential applications in dentistry and orthopaedics.
Introduction
Since Professor Brånemark first reported the phenomenon of “osseointegration,” the term osseointegration has been used to describe the stable combination of titanium and bone tissue [Citation1,Citation2]. Titanium and its alloys have good mechanical properties, biocompatibility and corrosion resistance. They are mature biomaterials for use in dental and orthopaedic applications. However, titanium and its alloys are inert biological materials. They have poor bioactivity and cannot form solid chemical bonds with bone tissue quickly and effectively [Citation3]. They cannot achieve sufficient osseointegration, which leads to an increase in the aseptic loosening and premature failure of implants [Citation4]. To improve osseointegration and the success rate of implants utilizing titanium and its alloys, various surface treatments have been used.
Generally, the surface treatment of titanium implants depends on three factors: surface morphology [Citation5,Citation6], chemical composition [Citation7,Citation8] and drug delivery [Citation9–11]. Surface morphology, including the characteristics at the micro/nanoscale, the various shapes of the particles (rod, spherical, porous, etc.) and roughness, affect the adhesion, proliferation and differentiation of cells at the implant-bone interface, thereby affecting the formation and growth of new bone at the interface and the speed and quality of bone integration [Citation12–14]. The chemical components at the implant surface also play an important role in protein adsorption and cell adhesion [Citation15]. The addition of Ca, Si, P, Sr and other bioactive trace elements is the most common and useful method for improving this [Citation5,Citation16]. The addition of many bioactive ions can regulate the behaviour and function of cells at the implant-bone interface, thus affecting the efficiency of bone integration. During drug delivery, recombinant proteins are used to enhance vascularised bone regeneration around titanium implants and a proper surface structure is beneficial to the drug loading and release efficiency [Citation15]. In conclusion, the biological activity of the titanium implant surface is stronger after physical or chemical surface modification, which can promote more rapid bone integration.
Surface topography modification is an important way to improve the biological properties of implants [Citation17,Citation18]. Studies have confirmed that an improved microscale surface morphology has beneficial effects on osteoblast activity in vitro and bone conductivity in vivo [Citation12,Citation19,Citation20]. In recent years, common surface morphology modification methods have included chemical vapour deposition, alkali heat treatment, acid treatment, magnetron sputtering, plasma immersion ion implantation and micro-arc oxidation (MAO) [Citation21]. MAO can be used to form a ceramic film with high bonding strength on the surface of substrates in situ by controlling the power supply parameters and the composition of the electrolyte [Citation22]. MAO technology is characterized by simple operation, stable processing, a lack of limitations in terms of the workpiece geometry and control of the coating composition. It was found that coatings with different surface morphologies could be prepared on the surface of titanium by adjusting the electrical parameters and the composition and concentration of the electrolyte [Citation23,Citation24]. Our previous study has found that coatings with different pore sizes can be prepared by adjusting the duration of MAO, and the resulting rough, porous surface has good biocompatibility, which can better promote the adherence and growth of stem cells [Citation25]. Coatings with different surface morphologies can also be prepared by adjusting the MAO voltage [Citation24]. Studies show that too high or too low of a voltage will lead to an unsatisfactory surface morphology in the coating [Citation23,Citation24]. When the voltage is too low, it cannot exceed the critical breakdown voltage of the passive film on the titanium substrate. However, when the MAO voltage is too high, the micro-arc discharge is more intense, resulting in the ablation of the coating surface or even cracks [Citation26]. Therefore, only an appropriate voltage can be used to prepare more biologically active material coatings.
The addition of bioactive components is another important way to improve the biological properties of implants [Citation5,Citation17]. For example, adding biologically active elements such as Ca, P, Si and Sr by using MAO technology can significantly improve the morphology and biological properties of the titanium surface coating [Citation8,Citation27–29]. Silicon is an essential trace element in the human body and is widely distributed in all human tissues and organs [Citation30]. Electron microprobe analysis showed that silicon was mainly concentrated in the areas of growth in developing bone and had the highest activity in osteoblasts and it has been proven that silicon is effective in promoting the proliferation and differentiation of osteoblasts [Citation31]. In recent years, some silicon-containing materials have been widely used in the clinical research of implant materials because they can compensate for the inadequacy of the surface bioactivity of metal implants [Citation32,Citation33]. It has been found that materials with ion precipitates, such as silicon ions, can promote the expression of osteogenesis-related genes (BMP2, ALP, Runx2, etc.) and thus promote the proliferation, differentiation and mineralization of osteoblasts [Citation34]. Therefore, the incorporation of silicon into the surface of titanium is an effective way to improve the bioactivity of the material surface and promote good osseointegration.
In this study, we prepared bioactive silicon-incorporating coatings with micropore/microsphere topography on titanium plate surfaces in an electrolyte-containing silicate by adjusting the MAO voltage. The initial adhesion, proliferation and osteogenic differentiation of rat BMSCs () were examined to evaluate the biological properties of silicon-incorporating coatings with micropore/microsphere topography.
Materials and methods
Sample modification
The commercially obtained pure titanium material was cut into 10 mm × 10 mm × 1 mm plates. The titanium plates were polished sequentially with 400–1200 grit SiC sandpaper. The titanium plates were cleaned sequentially with acetone, ethanol and deionised water in an ultrasonic pot for 10 min and then dried at room temperature. The electrolyte was prepared with 0.02 M sodium dihydrogen phosphate pentahydrate (Na2HPO4·5H2O) and 0.1 M sodium metasilicate nonahydrate (Na2SiO3·9H2O). A model WHD-20 MAO power supply was used (Harbin University of Technology Sino-Russian Science and Technology Co., Ltd., China). The frequency and time were set to 50 Hz and 10 min, respectively. The voltage was set to 320 V, 350 V and 380 V for the MAO-320, MAO-350 and MAO-380 groups, respectively. The Ti group was the control group.
Material characteristics
Scanning electron microscopy (SEM, SEMS-3400N, Japan) was used to observe the surface morphology of the material. X-ray diffraction was used to analyze the surface phase composition (XRD, Rigaku, D/max-2550VB/PC, Japan) and an energy dispersive spectrometer was used to determine the element composition (EDS, JAX-8100, Japan). ImageJ and Digimizer software were used to analyze the diameter and number of micropores and microspheres on the surfaces of the materials [Citation25,Citation35,Citation36]. The surface morphology of the samples was examined by scanning probe microscopy (SPM, Di Nanoscope, Veeco, USA). The average roughness (Ra) of the coatings was calculated by means of SPM image analysis.
Cell culture
Tibias and femurs from 4-week-old SPF SD rats (provided by the Animal Laboratory Centre, Medical College of Sun Yat-sen University) were used as sources of bone marrow mesenchymal stem cells (BMSCs). The rats were sacrificed by the dislocation of the vertebra and disinfected in 75% alcohol for 3 min. The rats were placed in an ultra-clean bench and the femurs and tibias of the hind limbs were removed, after which the bone marrow was washed out with DMEM containing 1% penicillin-streptomycin (Gibco, USA) and 10% foetal bovine serum (Gibco, USA). The BMSCs were cultured in an incubator at 37 °C in 5% CO2 and the culture medium was changed every 3 days. The cells were passaged when they grew to 80–90% confluence and the second- and third-generation cells were used in this study.
Cell adhesion and extension
To detect cell adhesion and extension, the number and extension of the cell adhesions were measured by fluorescence staining after 4 h of cell culture on the material surface. BMSCs were inoculated onto the surface of the material for 4 h and then fixed at room temperature for 20 min with 4% paraformaldehyde (PFA). One group of fixed cells was used to detect the number of adherent cells. The nuclei were stained with 4′, 6-diamidino-2-phenylindole (DAPI, 1:1000 dilution) for 5 min, observed and photographed under a fluorescence microscope and then counted with Image-Pro Plus software (IPP, USA). Another group of fixed cells was used to detect the cell extension. The cytoskeleton was stained with FITC-phalloidin (Yeasen, China, 1:200 dilution), observed and photographed under a fluorescence microscope and measured with ImageJ software.
Morphological observation of BMSCs on the material surface
BMSCs from the four groups were inoculated onto the material surface and cultured in an incubator for 6 h and 24 h. The culture medium was removed from the culture dish, which was then rinsed with PBS to remove the non-adherent cells. The cells were fixed with 2.5% glutaraldehyde solution. Ethanol with a series of concentration gradient of 30, 50, 75, 90, 95, and 100 v/v% was used sequentially to dehydrate the samples. The samples were dehydrated at each gradient concentration for 15 min and the dehydrated samples were fully dried [Citation37]. After spraying gold on the surface of the materials, the cell morphology was observed by SEM.
Cell activity and proliferation
To detect cell viability, BMSCs were inoculated onto the surfaces of the samples for 24 h, stained with live-dead cell staining reagent (Yesen, China) for 10 min and then photographed under a fluorescence microscope. To detect the proliferation of cells on the metal surface, a cell counting kit-8 assay (CCK-8, Tongren Institute of Chemistry, Japan) and 5-ethynyl-2′-deoxyuridine (EdU, Yesen, China) tests were carried out. For the CCK-8 test, the samples were placed into 24-well plates and each group had 3 vice-wells. The BMSCs were inoculated onto the samples in a 24-well plate for 1, 3, and 7 days, and then the CCK-8 reagent was added and cocultured with the cells for 2 h. Then, the optical density (OD) value was measured at 490 nm. To detect the proliferation of cells on the surface of the materials more accurately, EdU fluorescence staining was performed. The cells were inoculated on the samples for 4 h to allow the cells to adhere completely. EdU dye (1:1000 dilution) was added and the cells were incubated in the incubator for 2 h. Before adding the Apollo dye solution and incubating them at room temperature for 30 min, the cells were fixed with 4% PFA and then permeated with 0.5% Triton X-100 for 20 min. The nuclei were stained with DAPI for 5 min and photographed under a fluorescence microscope.
Alkaline phosphatase (ALP) activity
After 7 days of inoculation on the surfaces of the samples, the cells were fixed with 4% PFA, and then ALP reagent (Beyotime, China) was added for staining for 30 min at 37 °C. The expression of ALP on the surfaces of the samples was observed and photographed under a stereomicroscope. To measure the ALP activity, the cell lysates and p-NPP (Beyotime, China) were cocultured at 37 °C for 30 min. The OD value was measured at 405 nm. The protein concentration was measured by the BCA method and the OD value was measured at 630 nm. The ALP semiquantitative activity was expressed as OD value/protein concentration.
Osteogenesis-related gene expression
After 7 days of the coculture of cells and samples, the total RNA was extracted with TRIzol reagent (Thermo Fisher Scientific, USA). cDNAs were generated using the PrimeScript 1st Strand cDNA Synthesis kit (Takara, Japan). The expression of the osteogenic-related genes ALP, OCN, OSX and BMP2 was detected by RT-qPCR. The housekeeper gene glyceraldehyde-3-phosphate dehydrogenase (GAPDH) was used for standardization. The sequences of the specific primers used in this study are listed in and were provided by Sangon Biotech (China).
Table 1. Primers used for RT-qPCR.
Statistical analysis
All the experimental results were expressed as the mean ± standard deviation. Each experiment was repeated at least three times. ANOVA and the Student-Newman-Keuls method (SNK) were used to test the differences between the different groups. Differences were considered statistically significant when p < .05 and p < .01.
Results
Surface morphology
As shown in , the SEM results showed that a large variety of micropore/microsphere topographies were observed on the surfaces of the samples in the MAO-320, MAO-350 and MAO-380 groups. A uniform volcanic pore shape was observed for the micropores in the MAO-320 and MAO-350 groups, while the micropores in the MAO-380 group were irregular. As shown in , the diameters of the micropores and microspheres increased with increasing voltage. Upon software analysis, we found that the aperture increased with increasing voltage and there was an obvious difference between the three groups (. Clearly, with increasing voltage, the number of micropores decreased gradually and there was a significant difference among the three groups (. The diameter and number of microspheres were also analysed by the software. The diameters of the microspheres increased with increasing voltage, and there was an obvious difference between the three groups (. It is noteworthy that the average diameter of the microspheres in the MAO-380 group was 10 μm, which was approximately 5 times greater than that of the microspheres in the MAO-320 and MAO-350 groups. The number of microspheres decreased gradually and there was a significant difference between the three groups (. In addition, we found that the surfaces of the microspheres were not smooth and there were many nanoscale rough structures (.
Material characteristics
shows three-dimensional SPM images of the MAO coatings formed by using different applied voltages. The coating surface of the material was rough and showed a typical porous and spherical structure. It is noteworthy that the microsphere structure on the surface of the MAO coating increased the roughness of the coating surface. shows the roughness changes in the samples before and after MAO treatment. The roughness in the Ti group was lower than that in the treated group and the roughness increased with increasing applied voltage. The roughness in all treated samples was observed at the microscale and the roughness in the MAO-380 group is significantly higher than that in the MAO-320 and MAO-350 groups.
Figure 4. Average roughness (Ra) of the four groups of samples. (a) Three-dimensional SPM maps of the surfaces of the samples (scale bar = 6 μm). (b) Ra of the samples (*p < .05 compared with Ti; ##p < .01 compared with Ti, MAO-320 and MAO-350).
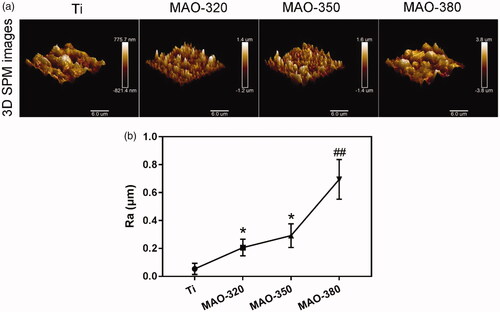
To further analyze the composition of the elements on the surfaces of the samples, we used the EDS method to detect the material coating. As shown in , Ti, O, Si, and P were clearly detected. As shown in , with increasing voltage, the weight% of Si increased gradually, while that of titanium decreased gradually. shows the XRD images of different samples after MAO treatment. From the graph, the existence of a titanium peak was detected on the surfaces of each group of samples, but no Si peak was detected. Titanium peaks were only detected in pure titanium after MAO treatment, but there was no peak position for TiO2, which indicated that TiO2 mainly existed in an amorphous form in the treated coating. Similarly, Si existed mainly in an amorphous state.
Cell adhesion and extension
We detected the number of cells that initially adhered to the surfaces of the samples. We found that the MAO-350 group adhered to more cells () in the initial 4 h and the number of adherent cells was significantly higher than that in the MAO-380 and Ti groups (), but there was no significant difference between the MAO-380 and Ti groups. We tested the cell extension on the surfaces of the samples; as shown in , it can be seen that the cells adhered well on the surfaces of each group of samples. The results of the software analysis showed that the average cell extension area in the MAO-350 group was the largest and was significantly greater than that of the MAO-380 and Ti groups, but there was no significant difference between the MAO-380 and Ti groups (. The above results showed that the MAO-350 group was more conducive to initial cell adhesion and extension.
Figure 7. Cell adhesion on the samples. (a) The number of adherent cells on the surfaces of the samples at 4 h (scale bar = 500 μm). (b) The number of adherent cells per unit area. (c) Fluorescence staining of actin on the sample surfaces at 4 h (Scale bar = 100 μm). (d) The average fluorescence area of the cells (*p < .05; n = 3).
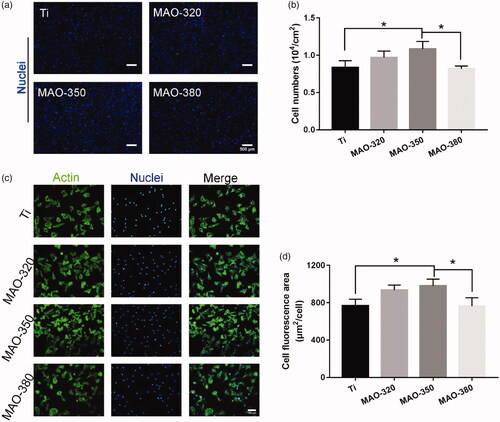
Cell morphology on the material surface
shows the morphology of the BMSCs cultured on the surfaces of the samples for 6 and 24 h. It can be seen from the figure that the adhesion speed of the Ti group, MAO-320 group and MAO-350 group was increased and the cells showed a more obviously stretched state on the surfaces of the three groups of materials, which is more conducive to the maturation of cells and the expression of related proteins. However, the extension area in the MAO-380 cells was not increased significantly at 6 h and 24 h, which may be related to the fact that the surface structure of the material was not conducive to cell adhesion.
Cell activity and proliferation
The results of live-dead staining () and the statistics for the live-dead cells () showed that there were no significant differences between the four groups, indicating that there was no significant difference between the surface toxicity of the treated groups and that of the Ti group. To detect the proliferation of cells on the surfaces of the samples, CCK-8 and EdU experiments were carried out. The EdU staining results () showed that the proliferation of BMSCs was different on different samples. The proliferation effect in the MAO-350 group was the most obvious () and the statistical results showed significant differences. The results of the CCK-8 () assay showed that the proliferation effect in the MAO-350 group was significantly improved at 1, 3 and 7 days. The above results showed that the treated samples had no obvious toxicity towards cells and the MAO-350 group was more conducive to cell proliferation.
Figure 9. Cell activity on the sample surfaces. (a) Live-dead fluorescence staining was performed 24 h after inoculation (scale bar = 100 μm). (b) Statistical results for the live and dead cells (n = 3).
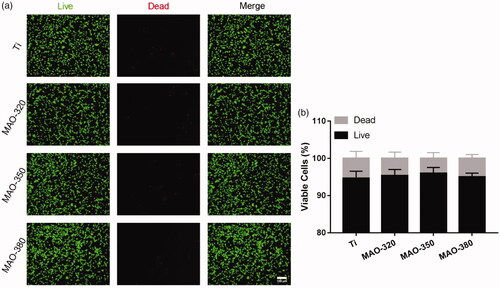
Figure 10. Cell proliferation on the surfaces of the samples. (a) Fluorescence staining for EdU at 2 h (scale bar = 500 μm). (b) Cell proliferation rate in the four groups of samples. (c) CCK-8 results for cells cultured on the four groups of samples for 1, 3 and 7 days (*p < .05; #p < .05 compared with Ti, MAO-320 and MAO-350; n = 3).
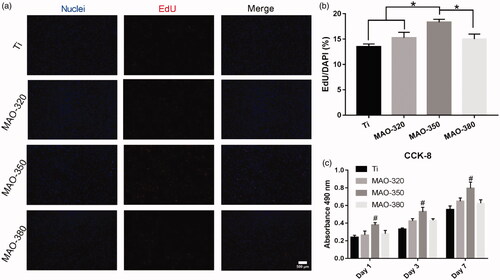
Osteogenic differentiation
ALP is an important indicator of early expression in the process of osteogenic differentiation and its secretion can reflect the state of osteogenic activity in cells. By combining the results of ALP staining () and semiquantitative detection (), it can be seen that the expression of ALP in the MAO-350 group was significantly higher than that in the Ti group, MAO-320 group and MAO-380 group. In addition, we detected the expression of ALP, OCN, BMP2 and OSX by RT-qPCR. According to the results (), we can see that the expression of the four osteogenesis-related genes in the MAO-350 group is significantly higher than that in the other three groups. It was concluded that the surface in the MAO-350 group was more conducive to the osteogenic differentiation of cells.
Discussion
MAO is a simple and effective chemical method for preparing high-strength, bonded oxide coatings on metal substrates and it is an interesting method for the adjustment of the composition and morphology of coatings by adjusting the electrical parameters and electrolyte composition [Citation5,Citation15]. It is noteworthy that a large number of studies have prepared coatings with different morphologies by adjusting the MAO voltage. Previous studies have shown that with increasing the film thickness and aperture increase, the surface becomes rougher [Citation37,Citation38]. When the voltage exceeds a certain limit, the film will be ablated [Citation39,Citation40]. It can be seen in and Citation3 that the surface morphology of the coating at 380 V is significantly different from that at 320 V and 350 V. Even the diameters of the microspheres are 5 times larger than those in the other two groups and the number of micropores decreases sharply and becomes irregular. The morphology of the coating is obviously not conducive to cell adhesion and osteogenic differentiation, which also shows that the voltage used for the preparation of the environment in this study should not exceed 380 V.
The surface properties of titanium and titanium alloys in terms of surface morphology and other physical and chemical properties have a significant impact on bone-implant bonding [Citation6]. Studies in vitro have shown that cell adhesion, morphology, mineralization and gene expression in osteoblasts are sensitive to microscale and nanoscale characteristics [Citation5,Citation33,Citation41]. Recently, some reports have also shown that surface morphology can significantly affect integrin expression, focal adhesions and cell signal transduction [Citation5,Citation6]. Compared with a smooth material surface, a microscale surface morphology that results in the increased roughness of the material surface makes it easier for cells and bone tissue to adhere to the material surface. Thus, a close mechanical chimaera will be formed to increase the stability of the implant [Citation42]. On the other hand, the micron-scale morphology can also increase the surface area of implanted materials to promote the adsorption of fibrin and provide a stable microenvironment for cells [Citation13,Citation43,Citation44]. Previous studies have shown that microporous coatings prepared by MAO are beneficial for cell adhesion and osteogenic differentiation [Citation25,Citation45]. In this study, when the MAO voltage was 350 V, the ratio of micropores to globules was more conducive to the adherence and osteogenic differentiation of stem cells.
The results show that the bioactivity of the implant surface is related not only to the surface morphology of the material but also to the chemical composition of the implant surface. Compared with the former, the chemical composition of the implant surface plays a more important role [Citation12,Citation15]. Therefore, to ensure the long-term stability of the implant, it is necessary to endow the material with bioactive elements. Studies have shown that MAO-Si coatings with enhanced cell compatibility and corrosion resistance were prepared by MAO with sodium silicate in the electrolyte [Citation33,Citation34]. In this study, silicon-containing coatings with different silicon contents were prepared by adding sodium silicate at the same concentration to the electrolyte and adjusting the voltage parameters. EDS analysis showed that silicon was successfully incorporated into the coating (). The XRD results () showed that no peak for Si was detected, indicating that the elemental Si in the silicon-doped coating mainly existed in an amorphous state, while the noncrystalline Si was easier to precipitate, which was conducive to the osteogenic differentiation of local cells ().
Cell-material interactions play an important role in the analysis of the mechanisms of bone integration [Citation46]. Cell adhesion is the first step in the interaction between cells and the underlying matrix, and cell adhesion is also a key regulator of cell proliferation, differentiation, activation and migration, which can determine the fate of the interaction between the biological interface and the implant [Citation6]. The silicon-incorporating micropore/microsphere coating prepared in this study was obviously beneficial for the initial adhesion, extension and proliferation of cells. ALP is a marker of early osteogenic differentiation that participates in the premineralization process and promotes the formation of mineral nodules [Citation47]. ALP staining and semiquantitative assays showed that the surfaces in the MAO-320 and MAO-350 groups were significantly beneficial to the expression of ALP (). The expression results for the osteogenesis-related genes () confirm the above results; that is, the material surfaces treated with appropriate voltage were obviously conducive to the osteogenic differentiation of cells, which may be related to the morphology of the material surface and the elemental silicon content.
In most cases, changes in one aspect of the material surface are always accompanied by significant changes in the other surface properties, so it is difficult to determine the main factors affecting cell responses [Citation5]. In this study, it was not clear whether the micropores/microspheres or silicon had a greater impact on the biological properties of the cells, but the results of the in vitro cytological experiments showed that the elements and microsurface morphology had a synergistic effect on the behaviour of cells. This study is a preliminary study on the biological properties of silicon-doped coatings with micropore/microsphere topography. Next, it will be necessary to further clarify the effects of element composition and morphology on the biological behaviour of cells and to explore the underlying mechanisms.
Conclusion
In this study, a novel silicon-incorporating coating with a micropore/microsphere topography was developed on a titanium surface by adjusting the MAO voltage. The coating significantly promoted the adhesion, proliferation and osteogenic differentiation of BMSCs in vitro, and these cytological behaviours were attributed to the synergistic effects of the micropore/microsphere topography and silicon incorporation. These results indicate that silicon-incorporating implant coatings with micropore/microsphere topography have potential applications in dentistry and orthopaedics.
Disclosure statement
No potential conflict of interest was reported by the authors.
Additional information
Funding
References
- Branemark PI, Adell R, Albrektsson T, et al. Osseointegrated titanium fixtures in the treatment of edentulousness. Biomaterials. 1983;4:25–28.
- Adell R, Lekholm U, Rockler B, et al. A 15-year study of osseointegrated implants in the treatment of the edentulous jaw. Int J Oral Surg. 1981;10(6):387–416.
- Jiang N, Guo Z, Sun D, et al. Promoting osseointegration of Ti implants through micro/nanoscaled hierarchical Ti phosphate/Ti oxide hybrid coating. ACS Nano. 2018;12(8):7883–7891.
- Nyan M, Tsutsumi Y, Oya K, et al. Synthesis of novel oxide layers on titanium by combination of sputter deposition and micro-arc oxidation techniques. Dent Mater J. 2011;30(5):754–761.
- Zhang W, Wang G, Liu Y, et al. The synergistic effect of hierarchical micro/nano-topography and bioactive ions for enhanced osseointegration. Biomaterials. 2013;34(13):3184–3195.
- Zhang W, Li Z, Liu Y, et al. Biofunctionalization of a titanium surface with a nano-sawtooth structure regulates the behavior of rat bone marrow mesenchymal stem cells. Int J Nanomedicine. 2012;7:4459–4472.
- Wu C, Zhou Y, Fan W, et al. Hypoxia-mimicking mesoporous bioactive glass scaffolds with controllable cobalt ion release for bone tissue engineering. Biomaterials. 2012;33(7):2076–2085.
- Kang JI, Son MK, Choe HC. Hydroxyapatite coatings containing Mn and Si on the oxidized Ti-6Al-4V alloy for dental applications. J Nanosci Nanotechnol. 2018;18(2):833–836.
- Liu Y, Enggist L, Kuffer AF, et al. The influence of BMP-2 and its mode of delivery on the osteoconductivity of implant surfaces during the early phase of osseointegration. Biomaterials. 2007;28(16):2677–2686.
- Souza AT, Bezerra BL, Oliveira FS, et al. Effect of bone morphogenetic protein 9 on osteoblast differentiation of cells grown on titanium with nanotopography. J Cell Biochem. 2018;119(10):8441–8449.
- Jiang L, Zhang W, Wei L, et al. Early effects of parathyroid hormone on vascularized bone regeneration and implant osseointegration in aged rats. Biomaterials. 2018;179:15–28.
- Li Y, Xiao Y, Liu C. The horizon of materiobiology: a perspective on material-guided cell behaviors and tissue engineering. Chem Rev. 2017;117(5):4376–4421.
- Gittens RA, McLachlan T, Olivares-Navarrete R, et al. The effects of combined micron-/submicron-scale surface roughness and nanoscale features on cell proliferation and differentiation. Biomaterials. 2011;32(13):3395–3403.
- Bai L, Liu Y, Du Z, et al. Differential effect of hydroxyapatite nano-particle versus nano-rod decorated titanium micro-surface on osseointegration. Acta Biomater. 2018;76:344–358.
- Zhang W, Cao H, Zhang X, et al. A strontium-incorporated nanoporous titanium implant surface for rapid osseointegration. Nanoscale. 2016;8(9):5291–5301.
- Hu D, Li K, Xie Y, et al. Different response of osteoblastic cells to Mg(2+), Zn(2+) and Sr(2+) doped calcium silicate coatings. J Mater Sci Mater Med. 2016;27(3):56.
- Kim HD, Amirthalingam S, Kim SL, et al. Biomimetic materials and fabrication approaches for bone tissue engineering. Adv Healthc Mater. 2017;6(23):1700612.
- Guglielmotti MB, Olmedo DG, Cabrini RL. Research on implants and osseointegration. Periodontol 2000. 2019;79(1):178–189.
- Li G, Cao H, Zhang W, et al. Enhanced osseointegration of hierarchical micro/nanotopographic titanium fabricated by microarc oxidation and electrochemical treatment. ACS Appl Mater Interfaces. 2016;8(6):3840–3852.
- Li X, Xu H, Zhao B, et al. Accelerated and enhanced osteointegration of MAO-treated implants: histological and histomorphometric evaluation in a rabbit model. Int J Oral Sci. 2018;10(2):11.
- Le Guehennec L, Soueidan A, Layrolle P, et al. Surface treatments of titanium dental implants for rapid osseointegration. Dent Mater. 2007;23:844–854.
- Bai Y, Zhou R, Cao J, et al. Microarc oxidation coating covered Ti implants with micro-scale gouges formed by a multi-step treatment for improving osseointegration. Mater Sci Eng C Mater Biol Appl. 2017;76:908–917.
- Wang Y, Jiang B, Lei T, et al. Dependence of growth features of microarc oxidation coatings of titanium alloy on control modes of alternate pulse. Mater Lett. 2004;58(12–13):1907–1911.
- Du Q, Wei D, Wang Y, et al. The effect of applied voltages on the structure, apatite-inducing ability and antibacterial ability of micro arc oxidation coating formed on titanium surface. Bioact Mater. 2018;3(4):426–433.
- Zhou W, Huang O, Gan Y, et al. Effect of titanium implants with coatings of different pore sizes on adhesion and osteogenic differentiation of BMSCs. Artif Cells Nanomed Biotechnol. 2019;47(1):290–299.
- Yao ZQ, Ivanisenko Y, Diemant T, et al. Synthesis and properties of hydroxyapatite-containing porous titania coating on ultrafine-grained titanium by micro-arc oxidation. Acta Biomater. 2010;6(7):2816–2825.
- Chung CJ, Long HY. Systematic strontium substitution in hydroxyapatite coatings on titanium via micro-arc treatment and their osteoblast/osteoclast responses. Acta Biomater. 2011;7(11):4081–4087.
- Sato M, Chen P, Tsutsumi Y, et al. Effect of strontium ions on calcification of preosteoblasts cultured on porous calcium- and phosphate-containing titanium oxide layers formed by micro-arc oxidation. Dent Mater J. 2016;35(4):627–634.
- Yuan X, Cao H, Wang J, et al. Immunomodulatory effects of calcium and strontium co-doped titanium oxides on osteogenesis. Front Immunol. 2017;8:1196.
- Biss EE, Epting T, et al. Reference values for serum silicon in adults. Anal Biochem. 2005;337:130–135.
- Landis WJ, Lee DD, Brenna JT, et al. Detection and localization of silicon and associated elements in vertebrate bone tissue by imaging ion microscopy. Calcif Tissue Int. 1986;38(1):52.
- Kaya S, Cresswell M, Boccaccini AR. Mesoporous silica-based bioactive glasses for antibiotic-free antibacterial applications. Mater Sci Eng C Mater Biol Appl. 2018;83:99–107.
- Liu H, Huang X, Yu H, et al. A cytocompatible micro/nano-textured surface with Si-doped titania mesoporous arrays fabricated by a one-step anodization. Mater Sci Eng C Mater Biol Appl. 2017;73:120–129.
- Sun J, Wei L, Liu X, et al. Influences of ionic dissolution products of dicalcium silicate coating on osteoblastic proliferation, differentiation and gene expression. Acta Biomater. 2009;5(4):1284–1293.
- Taniguchi N, Fujibayashi S, Takemoto M, et al. Effect of pore size on bone ingrowth into porous titanium implants fabricated by additive manufacturing: an in vivo experiment. Mater Sci Eng C. 2016;59:690–701.
- Rnjak-Kovacina J, Wise SG, Li Z, et al. Tailoring the porosity and pore size of electrospun synthetic human elastin scaffolds for dermal tissue engineering. Biomaterials. 2011;32(28):6729–6736.
- Wang X, Lu T, Wen J, et al. Selective responses of human gingival fibroblasts and bacteria on carbon fiber reinforced polyetheretherketone with multilevel nanostructured TiO2. Biomaterials. 2016;83:207–218.
- Li L-H, Kong Y-M, Kim H-W, et al. Improved biological performance of Ti implants due to surface modification by micro-arc oxidation. Biomaterials. 2004;25(14):2867–2875.
- Guo H, An M, Huo H, et al. Microstructure characteristic of ceramic coatings fabricated on magnesium alloys by micro-arc oxidation in alkaline silicate solutions. Appl Surf Sci. 2006;252(22):7911–7916.
- Song W-H, Jun Y-K, Han Y, et al. Biomimetic apatite coatings on micro-arc oxidized titania. Biomaterials. 2004;25(17):3341–3349.
- Zhang W, Li Z, Huang Q, et al. Effects of a hybrid micro/nanorod topography-modified titanium implant on adhesion and osteogenic differentiation in rat bone marrow mesenchymal stem cells. Int J Nanomedicine. 2013;8:257–265.
- Xiu P, Jia Z, Lv J, et al. Tailored surface treatment of 3D printed porous Ti6Al4V by microarc oxidation for enhanced osseointegration via optimized bone in-growth patterns and interlocked bone/implant interface. ACS Appl Mater Interfaces. 2016;8(28):17964–17975.
- Zhang R, Elkhooly TA, Huang Q, et al. A dual-layer macro/mesoporous structured TiO2 surface improves the initial adhesion of osteoblast-like cells. Mater Sci Eng C Mater Biol Appl. 2017;78:443–451.
- Wang G, Li J, Zhang W, et al. Magnesium ion implantation on a micro/nanostructured titanium surface promotes its bioactivity and osteogenic differentiation function. Int J Nanomedicine. 2014;9:2387–2398.
- Lee SJ, Choi JS, Park KS, et al. Response of MG63 osteoblast-like cells onto polycarbonate membrane surfaces with different micropore sizes. Biomaterials. 2004;25(19):4699–4707.
- Li J, Song Y, Zhang S, et al. In vitro responses of human bone marrow stromal cells to a fluoridated hydroxyapatite coated biodegradable Mg-Zn alloy. Biomaterials. 2010;31(22):5782–5788.
- Cao FY, Fan JX, Long Y, et al. A smart fluorescence nanoprobe for the detection of cellular alkaline phosphatase activity and early osteogenic differentiation. Nanomedicine. 2016;12(5):1313–1322.