Abstract
The present study highlights the biological synthesis of silver nanoparticles (AgNPs) using Sphingobium sp. MAH-11 and also their antibacterial mechanisms against drug-resistant pathogenic microorganisms. The nanoparticle synthesis method used in this study was reliable, facile, rapid, cost-effective and ecofriendly. The AgNPs exhibited the highest absorbance at 423 nm. The TEM image expressed spherical shape of AgNPs and the size of synthesized AgNPs was 7–22 nm. The selected area diffraction (SAED) pattern and XRD spectrum revealed the crystalline structure of AgNPs. The results of FTIR analysis disclosed the functional groups responsible for the reduction of silver ion to metal nanoparticles. The biosynthesized AgNPs showed strong anti-microbial activity against drug-resistant pathogenic microorganisms. Moreover, Escherichia coli and Staphylococcus aureus were used to explore the antibacterial mechanisms of biosynthesized AgNPs. Minimal inhibitory concentrations (MICs) of E. coli and S. aureus were 6.25 μg/mL and 50 μg/mL, respectively and minimum bactericidal concentrations (MBCs) of E. coli and S. aureus were 25 μg/mL and 100 μg/mL, respectively. Results exhibited that biosynthesized AgNPs caused morphological changes and injured the membrane integrity of strains E. coli and S. aureus. The AgNPs synthesized by Sphingobium sp. MAH-11 may serve as a potent antimicrobial agent for many therapeutic applications.
Introduction
Bio-nanotechnology is an emerging and promising field in modern science which is the combination of biotechnology and nanotechnology that deals with the use of a biological system for eco-friendly synthesis of nanomaterials and nanoparticles. Metal nanoparticles are gaining importance because of their various applications in different fields of modern science including medical and agricultural sciences. Various types of nanoparticles have been reported such as silver, gold, copper, zinc, alginate, magnesium and titanium [Citation1–3]. Among various kinds of nanoparticles, silver nanoparticles (AgNPs) are widely used nanoparticles because of its potential applications in different fields of science including optics, optoelectronics, medical and pharmaceutical sciences for the development of therapeutic agents, biosensors, drug delivery, gene therapy, chronic disease diagnostics, etc [Citation4–7]. In recent years, AgNPs have been tested for remarkable applications such as on patients for dental implants, bone cement, trauma and prostheses [Citation8], and on protective clothing [Citation9], etc.
It is reported that AgNPs have strong antibiofilm, antimicrobial, anticancer and anti-inflammatory activity, out of which the antimicrobial properties of AgNPs against pathogenic and multi-drug resistant microorganisms including bacteria, viruses and eukaryotic micro-organisms have been exploited well [Citation3,Citation10]. Previous reports suggest that AgNPs have strong bactericidal and broad-spectrum sterilization effect against many pathogenic and drug-resistant microorganisms, the nanoparticles can kill over 650 kinds of bacteria in a short time [Citation11]. Because of strong antimicrobial properties, the use of AgNPs has been commercialized in numerous industrial products such as for wound dressings, hang gel, footwear, paints, medical catheter coverings, cosmetics, plastics and cavity filler [Citation12]. The accurate mechanism and manner of action behind the antimicrobial efficacy of AgNPs are still not well known. However, there are several proposed mechanisms for the action of nanoparticles, such as disturbance of the cell wall or cell membrane; alteration of nutrient uptake and electron transport, by damaging DNA and proteins or through the generation of reactive oxygen species (ROS), which lead to cell death [Citation13–15]. The silver nanoparticle is becoming as an ideal candidate in the medical and biological platform due to its antibiofilm, antimicrobial, anticancer, anticoagulant and anti-inflammatory efficacies [Citation3].
Physical and chemical methods used for the synthesis of metal nanoparticles are well known but these methods are expensive and involve the use of hazardous chemicals or high energy, produce a large number of hazardous byproducts and create environmental contamination [Citation16]. To overcome these limitations of chemical and physical methods, eco-friendly, non-toxic, facile and low-cost methodologies are essential for the synthesis of metal nanoparticles by avoiding the use of expensive and toxic solvent and chemicals [Citation7]. Recently, the synthesis of nanoparticles using a biological system has been highlighted, which is eco-friendly, non-toxic, safe, green, facile and economical [Citation3]. Among the biological synthesis methodologies, bacteria have received the most attention in the biosynthesis of nanoparticles because of its growing success, ease of manipulation, ease of handling and genetic modification [Citation17]. Recently several microorganisms, such as Brevibacterium frigoritolerans, Bacillus methylotrophicus and Novosphingobium sp. THG-C3 have been isolated for the biosynthesis of AgNPs [Citation7,Citation15,Citation18]. In this study, Sphingobium sp. MAH-11 strain was isolated from soil and used for the rapid, facile and eco-friendly synthesis of AgNPs. The utilization of culture supernatants from various bacteria for the biosynthesis of AgNPs is well known. Bacterial culture supernatant contains various enzymes including reductases which are responsible for the synthesis of nanoparticles, produced and secreted by microorganisms [Citation19]. In this study, the culture supernatant of Sphingobium sp. MAH-11 was used for the green and rapid synthesis of AgNPs without the addition of any reducing agent. The biosynthesized AgNPs were characterized and their antimicrobial activity against drug resistant pathogenic microbes was evaluated. In addition, the antibacterial mechanisms were studied by investigating the cell morphology of Escherichia coli and Staphylococcus aureus. The method used in the present study for the biosynthesis of AgNPs is facile, convenient, straightforward, rapid and eco-friendly. Moreover, this is the first report for the biosynthesis of AgNPs using Sphingobium sp. MAH-11.
Experimental
Materials
All media were purchased from Difco, MB Cell (Seoul, Korea). Standard antibiotics discs: penicillin G (P10) 10 μg/disc, erythromycin (E15) 15 μg/disc and vancomycin (VA30) 30 μg/disc were obtained from Oxoid Ltd., Basingstoke, UK. Analytical grade AgNO3 (silver nitrate) was purchased from Sigma-Aldrich Chemicals, St. Louis, MO. The pathogenic bacterial strains of E. coli [ATCC 10798], Pseudomonas aeruginosa [ATCC 6538] and S. aureus [ATCC 6538] were collected from Korean Collection for Type Cultures (KCTC) and Korean Agricultural Culture Collection (KACC).
Isolation and identification of bacterial strain
The bacterial strain MAH-11 was isolated from a soil sample, located in Anseong, Republic of Korea. 1 g soil sample was suspended in 10 mL sterile saline solution (0.85% NaCl), serially diluted and then spread on R2A agar (Reasoner’s 2A agar) plates containing 0.5 g yeast extract, 0.5 g protease peptone No. 3, 0.5 g casamino acids, 0.5 g dextrose, 0.5 g soluble starch, 0.3 g dipotassium phosphate, 0.05 g magnesium sulphate, 0.3 g sodium pyruvate, and 15 g agar in 1000 mL distilled water pH 7.0, to obtain isolated colonies. For the screening of AgNPs synthesis ability, the isolated colonies were streaked on R2A plate supplemented with 1 mM filter-sterilized silver nitrate (AgNO3) solution. Then, the plates were incubated at 30 °C for 48 h and observed bacterial growth. The strain was preserved at −80 °C as a suspension in R2A broth with 30% glycerol (v/v). Strain MAH-11 has been deposited to the KACC (number 19836). Genomic DNA of isolated strain MAH-11 was extracted and purified using a commercial genomic DNA extraction kit (Solgent, Daejeon, Korea). The universal bacterial primer pair 27F and 1492R were used to amplify 16S rRNA gene and then the purified PCR products were sequenced by Solgent Co., Ltd. (Daejeon, Korea). The 16S rRNA gene sequences of related taxa were collected from GenBank database and EzTaxon-e server [Citation20]. To discover the phylogenetic location of strain MAH-11, the phylogenetic tree was constructed using the neighbour-joining method [Citation21] and MEGA6 programme package [Citation22]. Other biochemical characteristics and enzyme activities were tested using API ZYM and API 20NE kits according to the manufacturer’s instructions (bioMérieux, Marcy-l'Étoile, France).
Biological synthesis of AgNPs using strain MAH-11
For the synthesis of AgNPs, strain MAH-11was cultured in 100 mL of R2A broth and incubated in shaking incubator at 30 °C and 140 rpm for 2 days. After 48 h of incubation, the supernatant was collected by centrifugation at 10,000 rpm for 10 min. 0.1 mL filter-sterilized AgNO3 solution (1 M/mL) was added to 100 mL of supernatant and incubated for 24 h in an orbital shaker at 140 rpm and 30 °C. The biosynthesis of AgNPs was confirmed through the colour change in the culture medium by visual inspection. Then, the mixture was first centrifuged with low rpm to remove any components of medium and finally, the synthesized AgNPs were collected by high-speed centrifugation at 16,000 rpm for 15 min. The pellets of biosynthesized AgNPs were washed several times using autoclaved distilled water and air-dried and used for characterization and antimicrobial studies.
Characterization of biosynthesized AgNPs
Ultraviolet-visible spectrophotometer analysis
The reduction of Ag + ions was observed and recorded using ultraviolet-visible (UV–vis) spectrophotometer (Optizen POP; Mecasys) in the range of 300–800 nm.
FE-TEM analysis
The morphology, elemental compositions and purity of biosynthesized AgNPs were investigated using field emission-transmission electron microscopy (FE-TEM), elemental mapping, energy dispersive X-ray (EDX) spectroscopy and selected area diffraction (SAED) pattern with a TEM-2100F (JEOL, Akishima, Japan) electron microscope operated at an accelerating voltage of 200 kV. A drop of biosynthesized AgNPs solution (dispersed in distilled water) was placed on a carbon-coated copper grid, drying at room temperature and then, transferring it to the microscope for analysis.
XRD analysis
The X-ray diffraction (XRD) analysis was accomplished over the 2θ range of 30–90° using X-ray diffractometer (D8 Advance, Bruker, Hamburg, Germany), operated at 40 kv, 40 mA with CuKα radiation, 0.02 step size and 6°/min scanning rate. Air-dried samples were used for XRD analysis.
FT-IR spectroscopy analysis
The Fourier transform-infra-red (FT-IR) spectrum was measured to identify the functional groups in the biomolecules which are responsible for synthesis and stabilization of AgNPs. FT-IR was performed using Perkin Elmer Fourier transform infra-red spectrometer in a range of 400–4000 cm−1 with resolution 4 cm−1. For FT-IR analysis, air-dried biosynthesized AgNPs and freeze-dried culture supernatant (positive control) in powder form were used. For freeze-drying, the culture supernatant was collected after centrifugation, incubated at −80 °C for 2 h and then put to freeze dryer machine.
DLS analysis
The particle size of biosynthesized AgNPs was investigated by dynamic light scattering (DLS) analysis using a Malvern Zetasizer Nano ZS90 (Malvern Instruments, Worcestershire, UK) at a 12° angle and at 25 °C. Pure water with viscosity 0.8878 cP, dielectric constant 78.3 and refractive index 1.3328 was used as the dispersive medium.
Antimicrobial activity of biosynthesized AgNPs
Disc diffusion assay was used to investigate the antimicrobial efficacy of biosynthesized AgNPs against pathogenic microbes. The tested pathogenic microbes (P. aeruginosa, S. aureus and E. coli) were grown overnight in Mueller–Hinton broth (MHB). 100 μL overnight growth bacterial culture of each pathogenic strain was spread thoroughly on Mueller–Hinton agar (MHA) plate. The sterile paper discs of 8 mm in diameter containing 30 μL of biosynthesized AgNPs (both 500 ppm and 1000 ppm, dissolved in water) were placed on the surface of the inoculated MHA plates. Then, the plates were incubated at 37 °C for 24 h. Likewise, the antibacterial activity of commercial antibiotics such as erythromycin (15 mg/disc), penicillin G (10 mg/disc) and vancomycin (30 mg/disc) has been inspected against P. aeruginosa, S. aureus and E. coli. The zones of inhibition were calculated after 24 h of incubation. The assay was carried out in triplets.
Determination of MIC and MBC
Escherichia coli and S. aureus were selected for this study as Gram-negative and Gram-positive model strains, respectively. Both strains were grown overnight at 37 °C in MHB and after the incubation period, turbidity was adjusted to approximately 106 CFUs/mL. MICs of the biosynthesized AgNPs were determined using a broth microdilution assay. Control experiments in the absence of AgNPs were also carried out. Briefly, two-fold serial dilutions of biosynthesized AgNPs were made in sterile R2A broth from a top concentration of 100 μg/mL to 1.56 μg/mL. 100 μL of the AgNPs dilutions was placed in a 96-well plate, and inoculated with 100 μL of the test organism in R2A to a final concentration of 5 × 105 CFU/mL and further incubated for 24 h (shaking) at 37 °C [Citation23]. The MIC of biosynthesized AgNPs was measured as the lowest concentration at which the growth of pathogenic bacterium was completely inhibited within 24 h of incubation at 37 °C. The optical density was taken and recorded every 3 h of incubation at 600 nm using a UV-vis spectrophotometer. For determination of MBC, 10 μL of each set was streaked on MHA plate and further incubated at 37 °C for 24 h. The plates were observed by the naked eye for determining the lowest concentration (MBC) that blocked bacterial growth [Citation23].
Study of morphogenesis of treated cells by FE-SEM
The morphological changes of strains E. coli and S. aureus were determined by SEM. In short, logarithmic growth phase cells (1 × 107 CFU/mL) were treated with biosynthesized AgNPs (suspended in 0.85% NaCl) at a concentration of 1 × MBC. As a control, bacterial cultures were treated by 0.85% NaCl without AgNPs. The overnight treated cells were harvested and washed with PBS to remove the residual media. Later, the cells were fixed with 2.5% glutaraldehyde for 4 h and washed with PBS several times. Cells were fixed again with 1% osmium tetroxide and subsequently, washed with PBS. Fixed cells were dehydrated by a series of ethanol concentrations (30, 50, 70, 80, 95, and 100% for 10 min) at room temperature. The samples were dried by using a desiccator. Later, cells were mounted on SEM metallic stubs and coated with gold. The structural changes of the cells were observed by FE-SEM (JSM-7100F, JEOL, Akishima, Japan) [Citation23].
Results and discussion
Characterization of AgNPs producing bacterial strain
The 16Sr RNA gene sequence of isolated strain MAH-11 was 1351 bp and the sequence of 16Sr RNA gene has been submitted to NCBI for GenBank accession number (MH553056). According to the EzTaxon-e server, strain MAH-11 showed highest sequence similarity with Sphingobium quisquiliarum P25T (98.1%). The phylogenetic analysis using neighbour-joining tree indicated that strain MAH-11 is clustered and clearly grouped within the genus Sphingobium (). The biochemical characteristics of isolated strain MAH-11 carried out using API ZYM and API 20 NE are shown in . The strain MAH-11 was positive for following enzyme activities: valine arylamidase, cystine arylamidase, leucine arylamidase, alkaline phosphatase, acid phosphatase, β-galactosidase, β-glucosidase, naphthol-AS-BI-phosphohydrolase and α-chymotrypsin. Weekly positive for following enzyme activities: lipase (C-14), α-glucosidase, esterase (C4), esterase lipase (C8) and trypsin, but negative for N-acetyl-β-glucosaminidase, α-galactosidase, α-fucosidase, α-mannosidase and β-glucuronidase (API ZYM). Tests for glucose fermentation, nitrate reduction and indole production are negative. Only D-glucose is utilized as sole carbon source, but other compounds such as L-arabinose, N-acetyl-glucosamine, D-mannitol, D-maltose, gluconate, D-mannose, malic acid, phenylacetic acid, adipic acid, triosodium citrate or capric acid are not utilized as sole carbon source (API 20 NE).
Figure 1. The neighbour-joining (NJ) tree based on 16S rRNA gene sequence analysis showing phylogenetic relationships of strain MAH-11 with related species. Bootstrap values more than 70% based on 1000 replications are shown at branching points. Scale bar, 0.05 substitutions per nucleotide position.
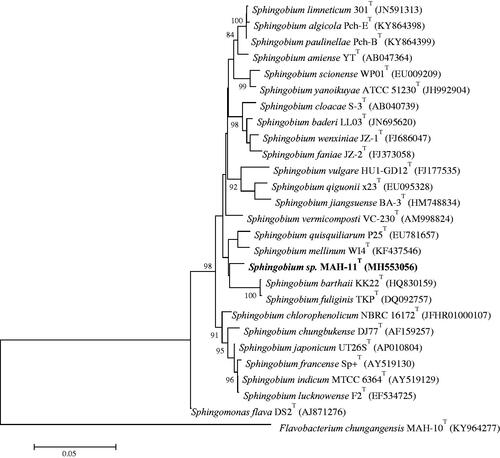
Table 1. Biochemical characterization of Sphingobium sp. MAH-11.
Biological synthesis of AgNPs
Biosynthesis of AgNPs by Sphingobium sp. MAH-11 was confirmed by visual observation of the colour of culture supernatant. The colour of MAH-11 culture supernatant was changed from watery yellow to deep brown within 24 h of incubation (). The colour change in culture supernatant indicates the synthesis of AgNPs, as the synthesized AgNPs cause surface plasmon resonance due to which deep brown colour appears [Citation3]. There was no colour changed in the control (without bacteria) when incubated for the same period and condition (). The exact mechanism of biosynthesis of AgNPs by bacteria is still in the preliminary stage. However, reports suggest that the enzymes secreted by microorganisms are an important part of the biological synthesis of nanoparticles. First, the metal ions are trapped inside or on the surface of bacterial cells. Then, the trapped metal ions are reduced to nanoparticles in the presence of microbial enzymes [Citation24,Citation25]. Two methods are available for the synthesis of nanoparticle using microorganisms which can be categorized as extracellular and intracellular depending on the place of nanoparticle synthesis. Extracellular synthesis of nanoparticles is more facile and convenient as the synthesized nanoparticles can be easily purified than intracellular synthesis which needs complex and difficult purification steps. In the present study, the extracellular methodology was used to synthesis AgNPs using Sphingobium sp. MAH-11 which is simple, green, eco-friendly and cost-effective.
Characterization of AgNPs
UV–vis spectrophotometer analysis
The synthesis of AgNPs was confirmed by UV-vis spectrophotometer scanning of the reaction mixture in the range of 300–800 nm. A strong peak at around 423 nm was observed (), which is attributed to the surface plasmon resonance band of AgNPs and indicated the formation of AgNPs. It is well known that the absorption peak at about 400–430 nm is due to surface plasmon resonance in nano-silver and confirmed the synthesis of AgNPs [Citation26].
FE-TEM analysis
FE-TEM images showed clear morphology of biological synthesized AgNPs. FE-TEM analysis revealed the spherical shape of biosynthesized AgNPs with size range of 7–22 nm (). showed the histogram of different sizes of biosynthesized AgNPs. The average size of biosynthesized AgNPs was 11.9 nm. Previous studies similarly configured the size and shape of biosynthesized AgNPs [Citation15]. The purity and composition of biosynthesized AgNPs were investigated using EDX spectrometer. EDX spectrum showed elemental signals of silver atoms in synthesized AgNPs approximately at 3 keV and exhibited homogenous distribution of AgNPs (). Reports suggest that EDX pattern of AgNPs usually showed typical absorption peak nearly at 3 keV [Citation18]. Additional signals of carbon and copper were also found in EDX spectrum because of the use of copper grid in FE-TEM. The elemental mapping results of synthesized AgNPs showed the highest distribution of silver elements which indicated that silver elements were the predominant in the sample ().
XRD spectrum of biosynthesized AgNPs
The XRD spectrum of the biosynthesized AgNPs showed the diffraction peaks at 2θ values of 38.144°, 44.266°, 64.479°, 77.451° and 81.454° match the respective 111, 200, 220, 311 and 222 lattice planes of AgNPs. The absence of other peaks indicates the purity of biosynthesized AgNPs (). The obtained XRD results were similar to a recently reported study, which showed the synthesis of AgNPs using microorganisms [Citation3,Citation15]. The SAED pattern showed sharp rings which reveal the crystalline structure of biosynthesized AgNPs and correspond to the following reflections at 111, 200, 220, 311 and 222. The crystalline structure of synthesized AgNPs was confirmed from both XRD spectrum and SAED pattern.
FT-IR profile of biosynthesized AgNPs
FTIR is an important tool for the investigation of functional groups that are responsible for the stabilization of synthesized nanoparticles. FT-IR spectrum that was recorded from freeze-dried powder of biosynthesized AgNPs reveals distinct peaks throughout the whole range of observation. FTIR analysis revealed visible bands at 3442.25, 2917.74, 2849.31, 2360.36, 1635.83, 1507.57 and 1112.58 cm−1 for biosynthesized AgNPs (). The band observed at 3442.25 cm−1 represents O–H (alcohol) and/or N–H (amine) stretching. Peaks at 2917.74 and 2849.31 cm−1 are attributed to C–H (alkane) stretching. The bands seen at 2360.36 cm−1 represents O = C=O (carbonyl bond group) stretching. The pronounced peak at 1635.83 cm−1 corresponds to the N–H (amine) bend. The bands observed at 1507.57 cm−1 and 1112.58 cm−1 can be assigned to the C–H (alkane) bend and C–O (alcohol/ether) stretching, respectively. FTIR spectrum of biosynthesized AgNPs () was compared with FTIR spectra of bacterial culture supernatant (, positive control) and AgNO3 (, negative control). It is evident that the biosynthesized AgNPs possess additional bands (functional groups) that are not originally observed in the FTIR spectrum of AgNO3 but observed in the FTIR spectrum of bacterial culture supernatant (). The FT-IR data suggests that the biological molecules could possibly involve in synthesis and stabilization of AgNPs. This data was supported by the previous study which showed the biological synthesis of nanoparticles using microorganisms [Citation27].
DLS analysis
The particle size of biosynthesized AgNPs was measured by DLS analysis. reveals the particle size distribution of synthesized AgNPs on the basis of intensity, number and volume. The average particle size of biosynthesized AgNPs was ∼82.7 nm with polydispersity value (0.366). The polydispersity value expresses the good quality of synthesized AgNPs. Previous studies have shown the similar particles size of biosynthesized AgNPs using microorganisms was 102 nm and 50–150 nm [Citation3,Citation7].
Antimicrobial activity of biosynthesized AgNPs
The multidrug-resistant pathogenic microorganisms have become a major concern in the medical field, as it is difficult to control these microbes by currently available commercial antibiotics. Biologically synthesized AgNPs were well known for their antimicrobial efficacy against pathogenic microbes [Citation3,Citation15,Citation17]. Biosynthesized AgNPs can be used to overcome the limitations of commercial antibiotics. In the present study, AgNPs were synthesized using the biological method and their antimicrobial efficacy was tested against multidrug-resistant pathogenic microorganisms (S. aureus, E. coli and P. aeruginosa). Results indicated that biosynthesized AgNPs have remarkable antimicrobial efficacy against all tested pathogens such as S. aureus, E. coli, and P. aeruginosa (). shows the clear zone of inhibition around the discs saturated with biosynthesized AgNPs (30 ul/disc, both 500 ppm and 1000 ppm). The average results of the zone of inhibition are shown in . The antimicrobial activity against pathogenic microbes was measured by calculating the diameter of zone of inhibition. The biosynthesized AgNPs showed the highest antimicrobial activity against antibiotics resistant P. aeruginosa followed by E. coli and S. aureus. The results of the present study suggest that the biosynthesized AgNPs were able to control the drug-resistant pathogenic bacteria and may have the potential to be used in the medical field. The present results of antimicrobial activity of biosynthesized AgNPs were consistent with a previously reported study [Citation15,Citation28]. Commercial antibiotics such as penicillin G, erythromycin and vancomycin were also used to check the antibacterial activity against pathogenic microbes such as P. aeruginosa, E.coli and S. aerius (). All these three pathogenic bacterial strains were resistant to the commercial antibiotics penicillin G, erythromycin and vancomycin and did not show any zone of inhibition around the antibiotic discs ().
Figure 7. Zones of inhibition of biosynthesized AgNPs (30 μL) at 500 ppm and 1000 ppm concentrations in the water against P. aeruginosa, E. coli and S. aureus.
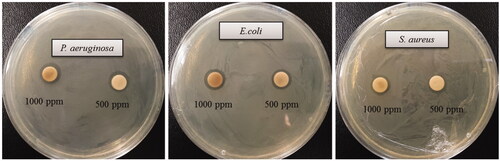
Figure 8. Zones of inhibition of commercial antibiotics against P. aeruginosa, E. coli and S. aureus. Abbreviation. E (erythromycin, 15 μg/disc), P (penicillin, G 10 μg/disc) and VA (vancomycin, 30 μg/disc).
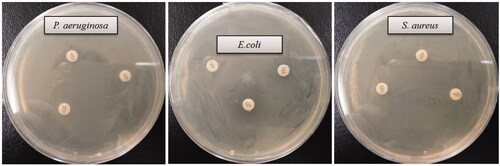
Table 2. Antimicrobial activity of biosynthesized AgNPs against P. aeruginosa, E. coli and S. aureus.
Table 3. Antimicrobial activity of commercial antibiotics against P. aeruginosa, E.coli and S. aureus. -: No zone of inhibition.
Determination of MIC and MBC
The MICs of biosynthesized AgNPs for E. coli and S. aureus were 6.25 and 50 μg/mL, respectively. showed that the biosynthesized AgNPs strongly suppressed the proliferation of strains E. coli and S. aureus. From this result, it was demonstrated that the MICs of the biosynthesized AgNPs by strain MAH-11 were significantly less than other antimicrobial agents. For example, the antimicrobial agent such as biosynthesized ZnO NPs where the MICs were 8 and 4 mg/mL against E. coli and S. aureus, respectively [Citation29]. Another antimicrobial agent was phenyl lactic acid where the MICs were 2.5 and 1.25 mg/mL against E. coli and L. monocytogenes, respectively [Citation30]. The MBCs of biosynthesized AgNPs for E. coli and S. aureus were 25 μg/mL and 100 μg/mL, respectively (). The result revealed that the MBCs of the newly synthesized antimicrobial agent using strain MAH-11 were much lower than those of previously synthesized antimicrobial agents [Citation29]. The MICs and MBCs of biosynthesized AgNPs were higher for S. aureus than those for E. coli. The higher AgNPs sensitivity of Gram-negative bacteria compared to that of Gram-positive bacteria is supported by the present study as well as previous studies [Citation23,Citation30]. This might be due to structural and compositional differences of the cell wall among Gram-negative and Gram-positive bacteria.
Disruption of E. coli and S. aureus after the treatment of the biosynthesized AgNPs
Escherichia coli and S. aureus cells that were treated with biosynthesized AgNPs were directly observed by FE-SEM to evaluate the morphological and structural changes (). As shown in , the untreated E. coli cells were observed to be normal rod-shaped with a regular surface (), while the cells that were treated with biosynthesized AgNPs displayed irregular, wrinkled, deformed and damaged outer surface and collapsed cell membranes (). On the other hand, the normal S. aureus cells were observed as regular plump sphere-shaped with smooth and intact cell surfaces without any damage (), while the cells treated with biosynthesized AgNPs appeared as deformed with a hole in the middle of the cell. The results demonstrated that the spherical-shape of S. aureus cell was no longer intact but appeared as deformed and damaged after AgNPs treatment (). The morphological changes of cell wall revealed that the newly biosynthesized AgNPs might interfere with the function of normal cells. The images revealed a higher sensitivity of E. coli than S. aureus, which might be due to the structural and compositional differences of the cell wall among Gram-negative and Gram-positive bacteria. It is worth to note that the present results were supported by previously published data [Citation23,Citation30]. Several proposed mechanisms have been reported for the antimicrobial activity of AgNPs. Among them, the broadly accepted mechanism is that of the positively charged silver ions in AgNPs, which can interact with various negatively charged sulphur or phosphorus bearing bio-macromolecules namely nucleic acids and proteins, leading to structural changes in bacterial cells. The structural changes involved the deformation of the cell wall and cell membrane might disrupt the metabolic process and ultimately cause the death of cells [Citation31]. Moreover, it was also suggested that nanoparticles produce numerous free radicals which are responsible for the damage of cell membrane through increased membrane permeability and finally lead to cell death [Citation32].
Conclusions
The present study reported the biologically rapid, facile and green synthesis of AgNPs using culture supernatant of Sphingobium sp. MAH-11 and also demonstrated the significant antimicrobial activity and mechanisms of biosynthesized AgNPs against multidrug-resistant pathogenic microbes. The methodology is eco-friendly, safe, facile, rapid, effective and economical. The strain Sphingobium sp. MAH-11 is capable of producing valuable AgNPs within 24 h of incubation. Additionally, the nanoparticle synthesis process was extracellular that makes the process more facile and convenient, which could be used for large-scale production. Moreover, the biosynthesized AgNPs showed significant antimicrobial activity against antibiotic-resistant human pathogenic strains S. aureus, E. coli, and P. aeruginosa. MICs of E. coli and S. aureus were 6.25 and 50 μg/mL, respectively, and the MBCs of E. coli and S. aureus were 25 and 100 μg/mL, respectively. The biosynthesized AgNPs cause morphological changes and could damage the cell wall and membrane integrity of strains E. coli and S. aureus, resulting in disrupting the reproduction of the cells and ultimately leading to cell death. This study might help to explain the antibacterial mechanism of AgNPs for both Gram-positive and Gram-negative pathogenic bacteria and promote the application of AgNPs as antimicrobial agent. Finally, the strain Sphingobium sp. MAH-11 could be useful for large scale production of AgNPs and synthesized AgNPs can be applied for many therapeutic applications as an antimicrobial agent.
Disclosure statement
No potential conflict of interest was reported by the author(s).
Additional information
Funding
References
- Schabes-Retchkiman PS, Canizal G, Herrera-Becerra R, et al. Biosynthesis and characterization of Ti/Ni bimetallic nanoparticles. Opt Mater. 2006;29(1):95–99.
- Ahmad Z, Pandey R, Sharma S. Alginate nanoparticles as antituberculosis drug carriers: formulation development, pharmacokinetics and therapeutic potential. Ind J Chest Dis Allied Sci. 2006;48:171–176.
- Singh P, Singh H, Kim YJ, et al. Extracellular synthesis of silver and gold nanoparticles by Sporosarcina koreensis DC4 and their biological applications. Enzym Microb Technol. 2016;86:75–83.
- Nam JM, Thaxton CS, Mirkin CA. Nanoparticle-based bio-bar codes for the ultrasensitive detection of proteins. Science. 2003;301(5641):1884–1886.
- Parak WJ, Gerion D, Pellegrino T, et al. Biological applications of colloidal nanocrystals. Nanotechnology. 2003;14(7):R15–R27.
- Majdalawieh A, Kanan MC, El-Kadri O, et al. Recent advances in gold and silver nanoparticles: synthesis and applications. J Nanosci Nanotech. 2014;14(7):4757–4780.
- Singh P, Kim YJ, Singh H. Biosynthesis, characterization, and antimicrobial applications of silver nanoparticles. Int J Nanomed. 2015;10:2567–2577.
- Zazo H, Colino CI, Lanao JM. Current applications of nanoparticles in infectious diseases. J Control Release. 2016;224:86–102.
- Farhadian N, Usefi Mashoof R, Khanizadeh S, et al. Streptococcus mutans counts in patients wearing removable retainers with silver nanoparticles vs those wearing conventional retainers: a randomized clinical trial. Am J Orthod Dentofac Orthop. 2016;149(2):155–160.
- Prabhu S, Poulose E. Silver nanoparticles: mechanism of antimicrobial action, synthesis, medical applications, and toxicity effects. Int Nano Lett. 2012;2(1):10.
- Nagasundaram N, Rahuman MA, Raghavan PS. Antibacterial application studies of nanosilver incorporated products. Int J Pharm Res Bio–Sci. 2014;3:153–164.
- Chaloupka K, Malam Y, Seifalian AM. Nanosilver as a new generation of nanoproduct in biomedical applications. Trends Biotechnol. 2010;28(11):580–588.
- AshaRani PV, Low Kah Mun G, Hande MP, et al. Cytotoxicity and genotoxicity of silver nanoparticles in human cells. ACS Nano. 2009;3(2):279–290.
- Marambio-Jones C, Hoek EM. A review of the antibacterial effects of silver nanomaterials and potential implications for human health and the environment. J Nanopart Res. 2010;12(5):1531–1551.
- Du J, Sing H, Yi TH. Biosynthesis of silver nanoparticles by Novosphingobium sp. THG-C3 and their antimicrobial potential. Artif Cells Nanomed Biotechnol. 2017;45(2):211–217.
- Rajeshkumar S, Malarkodi C, Paulkumar K. Intracellular and extracellular biosynthesis of silver nanoparticles by using marine bacteria Vibrio alginolyticus. J Nanosci Nanotechnol. 2013;3:21–25.
- Velusamy P, Kumar GV, Jeyanthi V, et al. Bio-inspired green nanoparticles: synthesis, mechanism, and antibacterial application. ToxicolRes. 2016;32(2):95–102.
- Wang C, Kim YJ, Singh P. Green synthesis of silver nanoparticles by Bacillus methylotrophicus, and their antimicrobial activity. Artif Cells Nanomed Biotechnol. 2016;44:1127–1132.
- Kumar SA, Abyaneh MK, Gosavi SW. Nitrate reductase-mediated synthesis of silver nanoparticles from AgNO3. Biotechnol Lett. 2007;29:439–445.
- Kim O-S, Cho Y-J, Lee K, et al. Introducing EzTaxon-e: a prokaryotic 16S rRNA gene sequence database with phylotypes that represent uncultured species. Int J Syst Evol Microbiol. 2012;62(3):716–721.
- Saitou N, Nei M. The neighbor-joining method: a new method for reconstructing phylogenetic trees. Mol Biol Evol. 1987;4:406–425.
- Tamura K, Stecher G, Peterson D, et al. MEGA6: molecular evolutionary genetics analysis version 6.0. Mol Biol Evol. 2013;30(12):2725–2729.
- Du J, Hu Z, Yu Z, et al. Antibacterial activity of a novel Forsythia suspensa fruit mediated green silver nanoparticles against food-borne pathogens and mechanisms investigation. Mater Sci Eng C. 2019;102:247–253.
- Hulkoti NI, Taranath TC. Biosynthesis of nanoparticles using microbes – a review. Colloids Surf B Biointerfaces. 2014;121:474–483.
- Singh OV. Bio-nanoparticles: Biosynthesis and Sustainable Biotechnological Implications. New York (NY): John Wiley & Sons; 2015.
- Singh N, Khanna PK. In situ synthesis of silver nano-particles in polymethylmethacrylate. Mater Chem Phys. 2007;104(2–3):367–372.
- Singh H, Du J, Singh P, et al. Extracellular synthesis of silver nanoparticles by Pseudomonas sp. THG-LS1.4 and their antimicrobial application. J Pharm Anal. 2018;8(4):258–264.
- Kalishwaralal K, BarathManiKanth S, Pandian SRK, et al. Silver nanoparticles impede the biofilm formation by Pseudomonas aeruginosa and Staphylococcus epidermidis. Colloids Surf B Biointerfaces. 2010;79(2):340–344.
- Rehman S, Jermy BR, Akhtar S, et al. Isolation and characterization of a novel thermophile; Bacillus haynesii, applied for the green synthesis of ZnO nanoparticles. Artif Cells Nanomed Biotechnol. 2019;47(1):2072–2082.
- Xu C, Li J, Yang L, et al. Antibacterial activity and a membrane damage mechanism of Lachnum YM30 melanin against Vibrio parahaemolyticus and Staphylococcus aureus. Food Control. 2017;73:1445–1451.
- Shankar S, Rhim JW. Amino acid mediated synthesis of silver nanoparticles and preparation of antimicrobial agar/silver nanoparticles composite films. Carbohydr Polym. 2015;130:353–363.
- Kim JS, Kuk E, Yu KN, et al. Antimicrobial effects of silver nanoparticles. Nanomed Nanotechnol Biol Med. 2007;3(1):95–101.