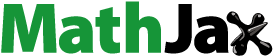
Abstract
Staphylococcus aureus is an important infectious factor in the food industry and hospital infections. Many methods are used for detecting bacteria but they are mostly time-consuming, poorly sensitive. In this study, a nano-biosensor based on iron nanoparticles (MNPs) was designed to detect S. aureus. MNPs were synthesized and conjugated to Biosensors. Then S. aureus was lysed and nano-biosensor (MNP-TiO2-AP-SMCC-Biosensors) was added to the lysed bacteria. After bonding the bacterial genome to the nano-biosensor, MNPs were separated by a magnet. Bacterial DNA was released from the surface of nano-biosensor and researched by Nano-drop spectrophotometry. The results of SEM and DLS revealed that the size of MNPs was 20–25 nm which increased to 38–43 nm after modification and addition of biosensors. The designed nano-biosensor was highly sensitive and specific for the detection of S. aureus. The limit of detection (LOD) was determined as 230 CFU mL−1. There was an acceptable linear correlation between bacterial concentration and absorption at 3.7 × 102–3.7× 107 whose linear diagram and regression was Y = 0.242X + 2.08 and R2 = .996. Further, in the presence of other bacteria as a negative control, it was absolutely specific. The sensitivity of the designed nano-biosensor was investigated and compared through PCR.
Introduction
Detection of pathogen microorganisms is very important in medical, health, and biologic fields. Staphylococcus aureus is one of the most ubiquitous pathogens in public healthcare worldwide. Different methods have been developed for detection of microorganisms, such as PCR, ELISA, SPR, and latex [Citation1–5]. PCR is one of the most sensitive methods for detection but it is able to detect only 103–107 CFU mL−1 [Citation3,Citation6,Citation7]. In ELISA method, an expert person could detect microorganisms with greater specificity compared to PCR method, but ELIASA is also limited to detecting 104–107 CFU mL−1 of microorganisms [Citation8–10]. Latex method is easier and quicker but less sensitive compared with ELISA method. SPR is a more accurate technique but needs an expert person to conduct the process and is more expensive. Therefore, offering a simple, sensitive, and specific method for microorganism detection and isolation is an important challenge of today [Citation11–14].
Over the past decades, nano-biotechnology has made great progress and opened new doors in different fields. Due to their physical and chemical properties, nanoparticles have created many opportunities in the development of different sciences. Iron nanoparticle (MNPs) is one of the important metal nanoparticles employed in different research fields [Citation15–18]. A variety of methods are used for iron nanoparticle production, including co-precipitation, hydrothermal sol-gel, micro-emulsion and some other methods with polymer utilized in the process of production. Co-precipitation is one of the commonest methods with sodium hydroxide and ammonia being the main components used in the production process [Citation19–22]. The size of MNPs is dependent on the following factors: the proportion of iron salt, type of salt and alkaline substance, mixing rate, pH, presence of nitrogen, and the temperature. MNPs are rapidly oxidized in the presence of oxygen and water causing precipitation and reduction in magnetic properties of nanoparticles [Citation23–25]. An effective technique to prevent this is creating a layer of particles such as silica, amine, carboxyl, thiol, gold, and silver on them and forming a core-layer structure [Citation26–29]. This process is called capsulation and creates a core-shell structure that is resistant to the presence of oxygen and water. In this case, MNPs have a higher magnetic strength than their oxide, which can be used as a superparamagnetic nanoparticle. Therefore, the formation of a coating on these nanoparticles could be preventing of perception and oxidation of MNPs [Citation30–32].
Modified magnetic nanoparticles have many usages in biology and medical fields, such as DNA extraction, purification, drug delivery, hyperthermal therapy, magnetic resonance imaging, enzyme activity assay, and separation of target cells. The amine form nanoparticles could be used for isolation and extraction of proteins and pathogenic bacteria by creating electrostatic, hydrogen and covalent bound [Citation32–34]. Also, magnetic nanoparticles modified with small biomolecules such as cytosine, mannose, antibody, Aptamers, DNA biosensors, and antibiotics are used for targeting and extracting bacteria, fungi, and viruses. The efficiency and specificity of the nanoparticles can be enhanced through the design and selection of appropriate functional groups. In this study, in order to boost the sensitivity and efficiency of DNA separation, MNPs’ surface was modified by an amine, SMCC and biosensor groups. Also, for elevating the specificity of detection of S. aureus target DNA, the MNPs were conjugated with DNA biosensors. The sensitivity of the nano-biosensor designed was investigated and compared through PCR and Nano-drop spectrophotometry.
Materials and methods
Materials
The standard bacteria of S. aureus ATCC 25923 and S. aureus ATCC 29213 as positive controls were prepared from Pasteur Institute, Tehran, Iran. The Escherichia coli ATCC 25,922 Pseudomonas aeruginosa ATCC 27853, Shigella dysentery PTCC 1188, Klebsiella pneumoniae ATCC7881 and Staphylococcus epidermidis ATCC 12228 as negative controls were prepared from Musavi Hospital, Zanjan, Iran. All oligonucleotides were purchased from Bioneer Co. (Daejeon, South Korea). dithiothreitol (DTT), ethyl acetate; NaCl, Luria broth (LB-broth), tetraethoxysilane (TEOS) and 3-aminopropyltriethoxysilane (APTES), ferric chloride hexahydrated (FeCl3·6H2O) and ferrous chloride tetrahydrate (FeCl2 4H2O) were prepared from Merck Co. Germany. Sulfosuccinimidyl 4-N-maleimidomethyl cyclohexane-1-carboxylate (SMCC) and sulfo-NHS acetate were prepared from Thermo Co. (Waltham, MA).
Designing primers and biosensors
For the detection of S. aureus, primers and biosensors were designed for protein A as a target DNA. Gene bank database was used for defining the protein A DNA sequence and a small sequence of protein A was selected as a target DNA. Oligo software (Sina gen, Co, Iran) and DNASIS Molecular Biology Insights, Inc., Cascade, CO) were used for designing forward, reverse primers, and biosensors. Primers and biosensors were synthesized by Bioneer Co. Korea. The primers and the biosensors sequence are presented in .
Table 1. Strand DNA and primers sequence.
MNPs synthesis
MNPs were prepared by alkaline co-precipitation of ferric chloride hexahydrates (FeCl3·6H2O) and ferrous chloride tetra hydrated (FeCl2·4H2O) in an aqueous solution. Briefly, 1.6 g FeCl3·6H2O and 0.8 g FeCl2·4H2O were mixed on a shaker at 80 °C. Ethanol and propanol (4 mL) were added to the mixture reaction. The reaction continued at 80 °C for 30–45 min. When the yellow colour of the solution changed to brown, hydroxide ammonia (NH3OH, 25%, 15 mL) was added to the reaction mixture through dripping to regulate the pH on 8–9 [Citation3,Citation19,Citation21]. When the formed black precipitated, the mixture reaction was shaken at 80 °C for 2–3 h, and then washed three times ().
Figure 1. Schematic view of the designed nano-biosensor for detection of S. aureus, (A) biosynthesis and conjugation of nanoparticles, (B) preparation of bacteria target DNA, (C) conjugation of target DNA with nano-biosensor, and (D) release of bacteria target DNA of nano-biosensor and the concentration determined by Nanodrop spectrophotometry.
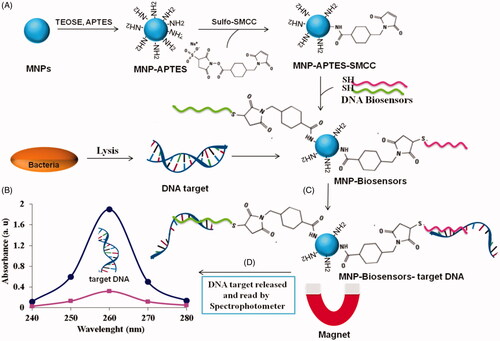
MNP-TiO2 synthesis
The MNPs prepared in Section “MNPs synthesis” were encapsulated by silica nanoparticles. Briefly, 2.5 g MNPs was added to 15 mL hydroxide ammonia (25%) dripping to regulate pH on 9–10. Then, 1.0 mL TEOS solution (98%) was added to the mixture reaction and shaken at 80 °C with 700 rpm for 1–2 h. The TEOS was bound to the surface of MNPs. The final production was MNPs-TiO2 which was isolated from the reaction mixture with a magnet and washed with distilled water three times () [Citation3,Citation19,Citation21].
MNP-TiO2-AP synthesis
The MNP-TiO2 synthesized in Section “MNP-TiO2 synthesis” was modified with APTES. For this purpose, hydroxide ammonia (25%, 15 mL) was added to the reaction mixture to regulate pH at 9–10. The APTES (0.5 mL, V/V 98%) was solved in ethanol (5 mL), after which the solution was added to the reaction mixture at 80 °C with 700 rpm for 3 h. Finally, MNP-TiO2-AP was isolated with a magnet and washed with distilled water three times () [Citation3,Citation19,Citation21].
MNP-TiO2-AP-SMCC synthesis
The MNP-TiO2-AP synthesized in Section “MNP-TiO2-AP synthesis” was mixed with 300 μg of SMCC at 5 mL of coupling buffer (0.1 M PBS, 0.2 M NaCl, pH 7.2) and mixed with 700 rpm at room temperature for 3–5 h. Afterwards, MNP-TiO2-AP conjugated with SMCC was isolated with a magnet. The MNP-TiO2-AP-SMCC was washed with coupling buffer three times. These nanoparticles could bind to the thiol agents of biosensor, enzymes, and proteins by a covalent band () [Citation3,Citation19,Citation21].
Functionalization of biosensor
Due to the presence of the thiol group on the biosensors, they form disulphide bonds with each other, reducing the efficiency of conjugation. Therefore, the disulphide bonds between biosensors must be broken [Citation3,Citation19,Citation21]. For this purpose, 15 µg mL−1 of each biosensor was added to 50 μL dithiothreitol (DTT, 1 N) for 1 h at room temperature. Before that, conjugated biosensors must be removed from DTT of the environment because of inhibiting conjugation between biosensors and nanoparticles. Accordingly, 100 μL ethyl acetate was added to the reaction mixture to DTT dissolved in the organic phase and removed from the reaction mixture. Then, the reaction mixture was centrifuged at 14,000 rpm for 15 min, whereby the organic phase was isolated from the watery phase. This process was repeated three times to remove all DTT from the reaction mixture. Immediately, the biosensors in the watery phase must have been used as disulphide bonds could form again [Citation3,Citation19,Citation21].
Functionalization of magnetic nanoparticles with biosensors
For the conjugation of biosensors, the activated biosensors (15 µg mL−1) were added to 1 mL of MNP-TiO2-AP-SMCC complex in the coupling buffer (0.1 M PBS, 0.2 M NaCl, pH 7.2) at 700 rpm, at room temperature, for 8–12 h. The product was isolated with a magnet and washed three times with the coupling buffer. The group agents of nanoparticles that were not conjugated with biosensors were deactivated with 10 µg of sulfo-NHS-acetate. The sulfo-NHS-acetate stopped the polymerization of nanoparticles via these group agents. To this end, sulfo NHS-acetate (10 mM, 5 mL) was added to the MNP-TiO2-AP-SMCC-biosensors complex at room temperature, for 5 h. Then, the complex was isolated from the reaction mixture by a magnet and washed three times with passivation buffer (0.2 M Tris, pH 8.5). Finally, the MNP-TiO2-AP-SMCC-biosensors complex was stored in a storage buffer (100 mM PBS, 0.2 M NaCl, pH 7.4) [Citation3,Citation19,Citation21]. The efficiency of conjugation was measured and researched by Nanodrop spectrophotometry ().
Where, E.C: Percentage of conjugation of biosensor, CT: Primary concentration of the biosensors, Cs: Concentration of the biosensors at supernatant.
Detection of target DNA
Staphylococcus aureus was cultivated in LB-Broth, for 18 h. specifically; 1 mL of the culture media was transferred to a micro tub and centrifuged at 5000 g for 10 min. Then, the supernatant was isolated from precipitation. Protease K (10 μL), lysozyme (10 μL), and 200 μL lyse solution (30 mM Tris-HCl, pH 7.0, 1 M NaCl, 4.5 M GuSCN, 20 mM EDTA, 1.5% Triton X-100) were added to the bacterial precipitation. The mixture was pipetted a few times and incubated at 55 °C for 30 min. Next, 30 mg of MNP-TiO2-AP-SMCC-biosensors complex was added to 300 μL bounding buffer (100 mM PBS buffer, 0.15 M NaCl, 0.1% SDS, pH 7.4) after which S. aureus bacteria were added to the solution. Bacterial DNA was released and bounded to the nano-biosensor (MNP-TiO2-AP-SMCC-biosensors) at 55 °C. Then, 250 μL isopropanol was added to the reaction mixture for 15-20 min. The MNP-nano-biosensor was bounded to target DNA isolated by a magnet. The nano-biosensor was washed three times with each of the following solutions: (WB1: 30 mM Tris-HCl, pH 7.0, 2.5 M GuSCN, 56% ethanol; WB2: 10 mM Tris-HCl, pH 7.0, 70% ethanol). Once the washing was finished, the remnant was left to be completely air-dried. Finally, 100 μL releasing or elution buffer (Elution buffer: 10 mM Tris-HCl, pH 8.5, 1 mM EDTA) was added to the dried precipitant MNP-TiO2-APTES-Biosensors-target DNA complex and incubated for 5 min at 65 °C. This process caused the release of target DNA from nano-biosensors [Citation6,Citation12,Citation22]. The MNP-TiO2-AP-Biosensors were isolated from target DNA free using a magnet. The concentration of extracted target DNA was assayed via Nanodrop spectrophotometry ().
Sensitivity and specificity of nano-biosensor
In this study, for sensitivity assay, McFarland solution was used for different bacterial concentrations prepared ranging from 3.7 × 101 up to 3.7 × 108. Briefly, each bacterial concentration was added to 30 mg of MNP-TiO2-AP-SMCC-Biosensors according to the method mentioned in Section “Detection of target DNA”. The target DNA extracted from each concentration was evaluated through Nano-drop spectrophotometry at 260 nm wavelength, PCR, and gel agarose electrophoresis. Finally, the sensitivity of the two methods was researched and compared with each other. Note that the PCR was performed in 50 µL volume while containing forward and reverse primers (0.4 mM, 10 pm μL−1), PCR Buffer (10X, 5 µL), MgCl2 (1.5 mM), dNTP (0.2 mM), target DNA (2 µL) and Taq DNA polymerase (2.5 U) under the following conditions: initial denaturation at 95 °C for 5 min, 30 cycles; secondary denaturation at 94 °C for 30 s; annealing at 54 °C for 30 s; the extension temperature at 72 °C for 30 s; and the final incubation temperature at 72 °C for 5 min. Finally, the PCR product was electrophoresed on 2% agarose gel [Citation2,Citation22]
The specificity of the nano-biosensor under optimized condition (3.7 × 105 CFU mL−1, 30 mg of MNP-TiO2-AP-SMCC-Biosensors) was determined with different species of bacterial target DNA including S. aureus ATCC 25923, S. aureus ATCC 29213 as positive controls, E. coli ATCC 25922, P. aeruginosa ATCC 27853, S. dysentery PTCC 1188, K. pneumoniae ATCC 7881 and S. epidermidis ATCC 12228 as negative controls. The extracted target DNA was evaluated via Nano-drop spectrophotometry ().
Results and discussion
Characterization of MNPs
Microscopic characterization of MNPs
SEM images of MNPs, MNPs-biosensors, and MNP-biosensors-target DNA are shown in . The results of this study revealed that the size of MNP was 20–25 nm (). After the modification of MNPs with TEOS, APTES, and SMCC, the size of MNPs increased from 20–25 nm to 30–35 nm and 35–43 nm, respectively (). The results also indicated that the structure of nanoparticles was spherical and semi-spherical ().
DLS
The size and potential of MNPs were analyzed by Dynamic Light Scattering (DLS). The results are shown in . The size of MNPs was measured as 25 nm; after adding TEOS, APTES, and SMCC on the surface of MNPs, it changed to 32, 35, and 36 nm, respectively (). The size of MNP-TiO2-AP-SMCC after conjugation with biosensors was changed from 36 nm to 38 nm up to 43 nm (). On the other hand, the potential of MNPs was −8 mV but after adding TEOS, APTES, and SMCC it changed from −8 mV to −21 mV. After adding biosensors, the potential was altered from −21 mV to −12 mV. Finally, when the MNPs were conjugated with target DNA, the potential changed from −12 mV to −16 mV, which is associated with oligonucleotides.
FTIR
FTIR technique was used to determine the characteristic of biochemical interactions between the macromolecules and the surface of MNPs at the wavelength 400–4000 cm– 1. shows the IR spectra of MNPs, MNP-TiO2-AP, MNP- TiO2-AP-SMCC-biosensor, and MNP- TiO2-AP-SMCC-biosensor-DNA. The absorption peak within 568–580 cm −1 wavelength is associated with the stretching vibration of Fe–O. The absorption peak at 1625 cm−1 is related to the stretching vibration of O–H showing the presence of water molecules at the surface of MNPs. The absorption peak at wavelengths of 1009–1018 cm−1 and 634 cm−1 is related to Si–O–Fe and Si–O–Si, respectively, indicating the presence of TiO2 bound on the surface of MNPs. Also, the absorption peak at wavelengths of 2830–2937 cm−1 is related to CH2 and CH3 on the surface of APTES. Three bonds of 1560–1670 cm−1 and 3435 cm−1 are related to O–H and free NH2 on the surface of APTES. Finally, the absorption peak at 2387 cm−1 is related to the presence of SH at the surface of SMCC and biosensors representing their connection to the surface of MNPs ().
Efficiency of conjugation
The efficiency of the conjugation of biosensors with MNPs complex was determined using Nanodrop spectrophotometry. For this purpose, the absorbance and concentration of biosensors before and after conjugation with nanoparticles were determined at 260 nm. According to the results, the efficiency of biosensors binding on the surface of MNPs was approximately determined as 83% ().
Optimization
MNPs concentration
For the detection of S. aureus, different concentrations of MNPs including 80, 60, 50, 40, 30, 20 and 10 mg mL−1 were used. The results revealed that the optimum concentration for bacterial detection was 30 mg mL−1 as seen in . At higher concentrations of MNPs, no significant change of absorption was observed, while at concentrations less than 30 mg mL−1, there was an increase in the absorption by elevating the concentration from 10 up to 30 mg mL−1 ().
SMCC concentration
For the bonding of biosensors to MNPs, SMCC was used as a linker. For this purpose, different concentrations of SMCC (500, 400, 300, 200 and 100 µg mL−1) were used. The biosensors under optimum conditions were conjugated with MNP-SMCC and the absorption of target DNA of each concentration was studied by Nano-drop spectrophotometry. The results of this study revealed that the optimum concentration of SMCC for conjugation of biosensors was 300 µg mL−1 observed in .
Biosensors’ concentration
For the detection and extraction of bacterial target DNA, different concentrations of biosensors were used. Under optimum conditions, concentrations of 1, 5, 10, 15, 20 and 25 nm of biosensors were used. The results showed that the optimal concentration of biosensors was 15 nm as displayed in .
Sensitivity
To determine the sensitivity of this method, different concentrations of bacteria 3.7 × 101–3.7 × 108 CFU mL−1 were used. Firstly, after lysis of the bacteria with the mentioned protocol, 30 mg mL−1 nano-biosensor was added to the reaction mixture. Bacterial target DNA bonded to the nano-biosensor and extracted by a magnet. The quantity of the extracted target DNA was studied by Nano-drop spectrophotometry. The results revealed that the designed nano-biosensor was well able to detect S. aureus (). According to the results, there was a linear relationship between the bacterial target DNA concentration and absorbance at concentrations of 3.7 × 102–3.7 × 107 CFU mL−1, as observed in . At concentrations greater than 3.7 × 107 CFU mL−1, no significant change occurred in the adsorption rate (). Linear equation and regression for detection of S. aureus were calculated as follows Y = 0.242X + 2.08 and R2 = .9957. Limit of detection of this method was defined as 230 CFU mL−1, suggesting its higher sensitivity compared to other reports CBDGFG. Also, PCR and gel agarose electrophoresis methods were used to compare and determine the sensitivity of the two methods. The results indicated PCR and gel agarose electrophoresis methods could detect bacterial target DNA at concentration of 3.7 × 103–3.7 × 108 CFU mL−1 and 3.7 × 104–3.7 × 108 CFU mL−1, respectively (); they have lower sensitivity compared to Nano-drop spectrophotometric method ().
Figure 4. (A) Sensitivity of the designed nano-biosensor at different concentrations of bacteria 3.7 × 101–3.7 × 108 CFU mL−1 (from A up to H), (B) linear relationship between different concentrations of bacteria and absorbance within 3.7 × 101–3.7 × 108 CFU mL−1, (C) linear relationship between different concentrations of bacteria and absorbance within 3.7 × 102–3.7 × 107 CFU mL−1, (D) sensitivity of PCR gel agarose electrophoresis at different concentrations of bacteria within 3.7 × 108–3.7 × 101 CFU mL−1 (from 1 to 8), respectively, M: size marker 100 bp.
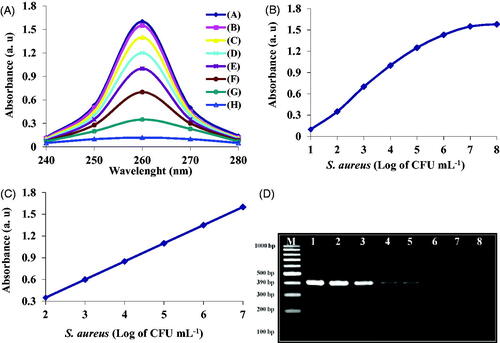
Table2. Comparison of the detection limit of S. aureus using different methods.
Specificity
To determine the specificity of this method, 30 mg mL−1 of the designed nano-biosensor were added to the target DNA (3.7 × 105 CFU mL−1) of S. aureus ATCC 25923 and S. aureus ATCC 29213 as positive controls. Further, 30 mg mL−1 of the designed nano-biosensor was added to the target DNA of E. coli ATCC 25922, P. aeruginosa ATCC 27853, S. dysentery PTCC 1188, K. pneumoniae ATCC7881, and S. epidermidis ATCC 12228 as negative controls. The results indicated that the nano-biosensor was specifically bound to S. aureus ATCC 25923 (, curve A), S. aureus ATCC 29213 (, curve B) target DNA, and increased the absorption rate as displayed in . However, the nano-biosensor was not bound to negative control bacterial target DNA (, curve from D up to H). Finally, there was a significant reduction in the absorption rate at 260 nm ().
Conclusions
In this study, a nano-biosensor based on iron nanoparticles (MNPs) was successfully designed for detection of S. aureus. Firstly, MNPs were synthesised and then the designed biosensors were conjugated with MNPs. The designed nano-biosensor was added to bacterial target DNA. After bonding of target DNA to the nano-biosensor, they were extracted using a magnet and washed for three times. Bacterial target DNA was released using a release buffer. The absorption and the concentration of target DNA were studied by spectrophotometry at 260 nm wavelength. Sensitivity of this method was studied at different concentrations of bacteria and compared with PCR and gel agarose electrophoresis. Also, the specificity was proved using positive controls and negative bacteria. The results of the study revealed that the size of synthesised MNPs was 20-25 nm which after conjugation with biosensors increased to 38–43 nm. The potential of modified MNPs was −21 mV; after conjugation, it changed into −16 mV. The optimal concentrations of biosensors, MNPs, SMCC linker, and bacterial concentration were determined as 15 nm, 30 mg mL−1, 300 µg, and 3.7 × 102–3.7 × 107, respectively. The minimum detection limit (LOD) was defined as 230 CFU mL−1. The sensitivity of the designed nano-biosensor was determined and compared to PCR and electrophoresis methods at different bacterial concentrations. Finally, the results indicated that the nano-biosensor was sensitive more than PCR and electrophoresis methods and completely specific for S. aureus target DNA.
Abbreviations | ||
MNPs | = | iron nanoparticles |
LOD | = | limit of detection |
SMCC | = | sulfosuccinimidyl 4-N-maleimidomethyl cyclohexane-1-carboxylate |
DTT | = | dithiothreitol |
LB-broth | = | Luria broth |
TEOS | = | Tetraethoxysilane; |
APTES | = | 3-aminopropyltriethoxysilane |
NHS | = | sulfo-NHS acetate |
MNP-TiO2-AP-SMCC-Biosensors | = | Nano-biosensors |
DLS | = | dynamic light scattering |
MNP-TiO2-AP-SMCC-biosensors | = | nano-biosensor |
HRP | = | horseradish peroxidase enzyme |
Disclosure statement
No potential conflict of interest was reported by the author(s).
References
- Piekarczyk J, Ratajkiewicz H, Jasiewicz J, et al. An application of reflectance spectroscopy to differentiate of entomopathogenic fungi species. J Photochem Photobiol B. 2019;190:32–41.
- Shahbazi R, Salouti M, Amini B, et al. Highly selective and sensitive detection of Staphylococcus aureus with gold nanoparticle-based core-shell nano biosensor. Mol Cell Probes. 2018;41:8–13.
- Amini B, Kamali M, Salouti M, et al. Fluorescence bio-barcode DNA assay based on gold and magnetic nanoparticles for detection of exotoxin A gene sequence. Biosensor Bioelectronics. 2017;92:679–686.
- Liu P, Han L, Wang F, et al. Sensitive colorimetric immunoassay of Vibrio parahaemolyticus based on specific nonapeptide probe screening from a phage display library conjugated with MnO2 nanosheets with peroxidase-like activity. Nanoscale. 2018;10(6):2825–2833.
- Liu P, Han L, Wang F, et al. Gold nanoprobe functionalized with specific fusion protein selection from phage display and its application in rapid, selective and sensitive colorimetric biosensing of Staphylococcus aureus. Biosens Bioelectron. 2016;82:195–203.
- Kose K. Nucleotide incorporated magnetic microparticles for isolation of DNA. Process Biochem. 2016;51:1644–1649.
- Su-Hua H. Gold nanoparticle-based immunochromatographic test for identification of Staphylococcus aureus from clinical specimens. Clin Chim Acta. 2006;373:139–143.
- Xiangyu M, Fulai L, Fan L, et al. Vancomycin modified PEGylated-magnetic nanoparticles combined with PCR for efficient enrichment and detection of Listeria monocytogenes. Sensors and Actuators B. 2017;247:546–555.
- Amini B, Kamali M, Salouti M, et al. Spectrophotometric, colorimetric and visually detection of Pseudomonas aeruginosa ETA gene based gold nanoparticles DNA probe and endonuclease enzyme. Spectrochimica Acta Part A. 2018;199:421–429.
- Ajima M, Piyasak C, Annop S. Colorimetric detection of Ehrlichia canis via nucleic acid hybridization in gold nano-colloids. Sensors. 2014;14:14472–14487.
- Jie Z, Cheng T, Lizeng G, et al. Silica coating magnetic nanoparticle-based silver enhancement immunoassay for rapid electrical detection of ricin toxin. Toxicon. 2010;55(1):145–152.
- Yalong B, Yan C, George C, et al. Synthesis of amino-rich silica-coated magnetic nanoparticles for the efficient capture of DNA for PCR. Colloids Surf B. 2016;145:257–266.
- Mohammad H, Nasrin S, Miguel de la G. Iron and iron-oxide magnetic nanoparticles as signal-amplification elements in electrochemical biosensing. Trends Anal Chem. 2015;72:1–9.
- Narmani A, Kamali M, Amini B, et al. Targeting delivery of oxaliplatin with smart PEG-modified PAMAM G4 to colorectal cell line In vitro studies. Process Biochem. 2018;69:178–187.
- Jia L, Yoshitaka K. Influence of silica coating process on fine structure and magnetic properties of iron oxide nanoparticles. Electrochim Acta. 2015;183:148–152.
- Zhang Z, Guan Y, Xia T, et al. Influence of exposed magnetic nanoparticles and their application in chemiluminescence immunoassay. Coll Surf A: Physicochem. 2017;520:335–342.
- Amini B, Kamali M, Abadi AZM, et al. Cloning of catalytic domain of exotoxin a from Pseudomonas aeruginosa. J Zanjan Univ Med Sci Health Serv. 2010;71:24–33.
- Jeong U, Shin HH, Kim Y. Functionalized magnetic core–shell Fe@SiO2 nanoparticles as recoverable colorimetric sensor for Co2+ ion. Chem Eng J. 2015;281:428–433.
- Narmani A, Kamali M, Amini B, et al. Highly sensitive and accurate detection of Vibrio cholera O1 OmpW gene by fluorescence DNA biosensor based on gold and magnetic nanoparticles. Process Biochem. 2018;65:46–54.
- Guo Y, Zhang L, Zhang S, et al. Fluorescent carbon nanoparticles for the fluorescent detection of metal ions. Biosens Bioelectron. 2015;63:61–71.
- Amini A, Kamali M, Amini B, et al. Bio-barcode technology for detection of Staphylococcus aureus protein A based on gold and iron nanoparticles. Int J Biol Macromol. 2019;124:1256–1263.
- Tang Y, Zou J, Ma C, et al. Highly sensitive and rapid detection of Pseudomonas aeruginosa based on magnetic enrichment and magnetic separation. Theranostics. 2013;3(2):85–92.
- Tangchaikeeree T, Sawaisorn P, Somsri S, et al. Enhanced sensitivity for detection of Plasmodium falciparum gametocytes by magnetic nanoparticles combined with enzyme substrate system. Talanta. 2017;164:645–650.
- Wang J, Qiu L, Wang C, et al. Probing antigen-antibody interaction using fluorescence coupled capillary electrophoresis. Int J Mol Sci. 2013;14(9):19146–19154.
- Amini A, Kamali M, Amini B, et al. Enhanced antibacterial activity of imipenem immobilized on surface of spherical and rod gold nanoparticles. J Phys D: Appl Phys. 2019;52(6):065401.
- Cheng P, Huang ZG, Zhuang Y, et al. A novel regeneration-free E. coli O157:H7 amperometric immunosensor based on functionalised four-layer magnetic nanoparticles. Sensors and Actuators B. 2014;204:561–567.
- Bai Y, Song M, Cui Y, et al. A rapid method for the detection of foodborne pathogens by extraction of a trace amount of DNA from raw milk based on amino-modified silica-coated magnetic nanoparticles and polymerase chain reaction. Anal Chim Acta. 2013;787:93–101.
- Abbaspour A, Norouz-Sarvestani F, Noori A, et al. Aptamer-conjugated silver nanoparticles for electrochemical dual-ap-tamer-based sandwich detection of Staphylococcus aureus. Biosens Bioelectron. 2015;68:149–155.
- Wang W, Xiao X, Ji L, et al. Carboxyl modified magnetic nanoparticles coated open tubular column for capillary electrochromatographic separation of biomolecules. J Chromatogr A. 2015;1411:92–100.
- Pivetal J, Ciuta G, Frenea-Robin M, et al. Magnetic nanoparticle DNA labeling for individual bacterial cell detection and recovery. J Microbiol Methods. 2014;107:84–91.
- Chan ML, Jaramillo G, Hristova KR, et al. Magnetic scanometric DNA microarray detection of methyl tertiary butyl ether degrading bacteria for environmental monitoring. Biosens Bioelectron. 2011;26(5):2060–2066.
- Yang K, Jenkins DM, Wen Su W. Rapid concentration of bacteria using submicron magnetic anion exchangers for improving PCR-based multiplex pathogen detection. J Microbiol Meth. 2011;86(1):69–77.
- Jin Y, Liu F, Shan C, et al. Efficient bacterial capture with amino acid modified magnetic nanoparticles. Water Res. 2014;50:124–134.
- Pinheiro PC, Daniel-da-Silva AL, Tavares DS, et al. Fluorescent magnetic bioprobes by surface modification of magnetite nanoparticles. Materials. 2013;6(8):3213–3225.
- Borsa BA, Tuna BG, Hernandez FJ, et al. Staphylococcus aureus detection in blood samples by silica nanoparticle oligonucleotides conjugates. Biosens Bioelectron. 2016;86:27–32.
- Yu J, Zhang Y, Zhang Y, et al. sensitive and rapid detection of Staphylococcus aureus in milk via cell binding domain of lysin. Biosens Bioelectron. 2016;77:366–371.
- Henares D, Brotons P, Buyse X, et al. Evaluation of the eazyplex MRSA assay for the rapid detection of Staphylococcus aureus in pleural and synovial fluid. Int J Infect Dis. 2017;59:65–68.