Abstract
In the present study, a novel single domain antibody (sdAb) fusion protein, named everestmab, composing of a mutated GLP-1(A8G) fused to the tandem bispecific humanized GLP-1R-targeting and albumin-binding nanobodies was designed and characterized for the therapies for type 2 diabetes mellitus (T2DM). Surface plasmon resonance (SPR) measurements demonstrated everestmab associates with serum albumins of rat and monkey species with high affinity, and tends to be cross-reactive with rat and monkey species. In vitro GLP-1R binding and activation assays revealed that everestmab can specifically activate the GLP-1R, and the antagonist exendin-4 (9–39) did not inhibit the activation yet. In vivo multiple oral glucose tolerance tests (OGTTs) and hypoglycaemic efficacy tests proved that a single injection of everestmab reduced the blood glucose for at least 144 h in Goto-Kakizaki (GK) rats. The plasma half-lives of 4.1 and 7.8 days were observed after a single s.c. administration of everestmab in SD rats and cynomolgus monkeys, respectively. Chronic treatment of everestmab to GK and diet induced obese (DIO) rats achieved beneficial effects on weight reducing, HbA1c lowering, glucose tolerance, liver and pancreas islet function impairment. In summary, everestmab is a unique G-protein-coupled receptor-targeted nanobody fusion protein and exerts potential as a therapeutic treatment for T2DM.
Introduction
Type 2 diabetes mellitus (T2DM), a chronic metabolic disorder, was characterized by hyperglycaemia resulting from progressive insulin resistance and relatively insufficient amount of secretion [Citation1,Citation2]. Not only that, T2DM is also a gradually deteriorating disease accompanied by weaker β-cell function and progressive diabetes-related complications over time [Citation3,Citation4]. Although several traditional hypoglycaemic agents have been widely utilized for glycemic control, continuing demands for improved diabetic therapies still exist [Citation5,Citation6]. Meanwhile, T2DM and obesity, which were collectively referred to as “diabesity”, were both associated with dramatic rise of morbidity and mortality around the world. So there is obviously clinical need for development of antidiabetic therapies with accompanied effectively weight lowering [Citation7,Citation8].
Glucagon-like peptide-1 (GLP-1) is a naturally occurring peptide hormone secreted from gut endocrine L-cells in response to nutrient ingestion, and plays an important role in glucose homeostasis and nutrient metabolism [Citation9–11]. GLP-1 exerts biological effects, especially for the protection of β-cells from progressive failure and hyperglycaemic effects, by binding and activating the GLP-1 receptor (GLP-1), a classical seven-transmembrane domain G-protein-coupled receptor [Citation12,Citation13]. Under physiological condition, GLP-1 is rapidly filtrated by glomerular and digested by dipeptidyl peptidase-IV (DPP-IV) leading to a half-life of ∼3 min which restricts its clinical scope. Although several incretin-based hypoglycaemic agents, such as exenatide and Liraglutide, have been widely utilized for glycemic control, continuing demands for the more long-lasting and comprehensive diabetic drugs is stillthere, especially in terms of weight reduction and protection of β-cells function [Citation14–17].
It is well-known that improvement of the physical interaction of GLP-1 with serum albumin represents an effective strategy to develop long-acting GLP-1 analogs [Citation18]. Single domain antibody or nanobodies are therapeutic proteins based on the smallest functional fragments of heavy chain-only (VHH) antibodies, naturally occurring in the Camelidae family [Citation19–21]. In present study, we describe aspects of a novel long-acting GLP-1R agonist, everestmab, consisting of three domains. As is showed in , first domain of everestmab was a mutated GLP-1(A8Aib) fragment with enhanced DPP-IV-resistant ability. The second domain was designed to improve the in vivo half-life of the fusion nanobody by binding to human serum albumin (HSA). The third domain was a GLP-1R-targeting nanobody to increase the binding robustness. Both the anti-HSA and anti-GLP-1R VHH were obtained from a phage display library derived from a llama immunized with each recombinant immunogen and following screening for HSA or GLP-1R binding. In the framework regions, VHH humanisation was conducted to yield a final domain with more than 90% homology compared to the human germline consensus sequence ().
Everestmab possesses pharmacological effects of the GLP-1 and the structural attribute of a specific single domain antibody with high affinity for HSA and GLP-1R. Its affinity and potency was characterized using in vitro systems, and in vivo pharmacodynamic properties were fully studied in Goto-Kakizaki (GK) and dose induced obesity (DIO) model in rats. In addition, the pharmacokinetic characters of everestmab were also investigated to identify its efficient long-acting antidiabetic properties.
Material and methods
Materials and animals
Everestmab were produced in a Pichia pastoris strain (Thermo Fisher Scientific, USA) and the medium containing products was subsequently clarified by filtration. Final products were obtained after being further purified using a process comprising affinity and ion-exchange column chromatography. The purity and mass of fusion proteins were identified using reversed high-performance liquid chromatography (RP-HPLC, Aglient, USA) and MALDI-TOF/MS (Bruker, Germany), respectively.
Type 2 diabetic GK rats, DIO rats and cynomolgus monkeys were purchased from JOINN Laboratories, Inc. (Beijing, China). Male DIO and GK rats rats were fed a diabetogenic diet, which is high fat diet with 60% kcal from fat (high-fat diet (60%) diet D12492), for a minimum of 2 months before experiments. During the experiment period, these model animals, were allowed with free access to diet and water, and accli mated to controlled ambient conditions of 12-h light/dark cycle. All studies were approved by and performed according to the guidelines of the Institutional Animal Care and Use Committee of the JOINN Laboratories and the approval codes was ACU18-101 and ACU19-009.
Surface plasmon resonance measurements
Surface plasmon resonance (SPR) measurements were performed with BIACORE T200 system (General Electric Company, USA) for evaluating associating affinity of everestmab for GLP-1R and serum albumins of mouse, rat, dog, monkey, and human species. Experimental details were carried out according to the user’s manual. In short, we fixed the different kinds of the albumins to the CM5 chips at the fixed level of ∼150 RU. Then the everestmab was diluted to eight gradients (0, 0.1, 0.3, 0.9, 2.7, 8.1, 24.3, and 72.9 nM) and flew through the CM5 chip coated with albumin at the flow rate of 45 μl/min using the HBS EP + buffer under the binding and dissociating duration of 300 s at 25 °C. The further parameter of binding affinity were obtained by standard fitting procedure introducing in a 1:1 binding model after the double deductions.
GLP-1 receptor activation assay
To evaluate the specificity of GLP-1R activation, everestmab was co-incubated with or without exendin-4 (9-39), a GLP-1R antagonist, in the Chinese hamster ovary (CHO) cells stably expressing hGLP-1R cells for 30 min at 4 °C in PBS containing 2% FBS and 0.02% sodium azide. The binding and activation of GLP-1R were assessed for cAMP using homogeneous time-resolved fluorescence technology (Cisbio Bioassays, France) in accordance with established protocols.
Islet insulin secretion
Islets were isolated from rats after common bile duct cannulation and tissues was distended after the collagenase IV (Sigma, USA) digestion and were subsequently cultured into fresh RPMI 1640 media (Thermo Fisher, USA) according to previous reports [Citation22,Citation23]. The purified islets were furtherly seeded at 10 islet/well in Krebs-Ringer bicarbonate (KRB) buffer containing 2.8 or 16.8 mM glucose, and incubated with different concentrations of dulaglutide and everestmab for 1 h. The secreted insulin level was detected by using insulin EIA kit.
Oral glucose tolerance test
After an overnight fast, GK rats were subcutaneously dosed with saline, everestmab (0.1, 0.3 and 0.9 mg/kg). Half an hour later, three groups were challenged with an oral bolus of 2 g/kg glucose. Blood glucose levels were detected using glucometers (JNJ, USA) at pre-dose, 15, 30, 45, 60, 120 and 180 min. Area under the curve (AUC) calculations were obtained from the glucose concentrations measured between 0 and 180 min. Multiple OGTTs were performed at an interval of 40 h after first administration of everestmab. Area under the curve (AUC) was calculated to evaluate the antihyperglycemic effect from 0 to 3 h, 40 to 43 h, 80 to 83 h and 120 to 123 h, separately.
Hypoglycaemic duration test
Under non-fasting conditions, male type 2 diabetic rats received a single subcutaneous injection of saline, dulaglutide (0.6 mg/kg) and everestmab (0.3 and 0.9 mg/kg). Blood samples were subsequently collected from tail veins at pre-dose and 0.5, 1, 2, 4, 8, 16, 24, 48, 72, 96, 144, 192, 240 h). BGLs were detected using a glucometer (Bayer, Germany). Glucose-lowering durations to under a BGL of 8.35 mmol/L (150 mg/dL) were also checked.
Pharmacokinetics in vivo
The pharmacokinetic profiles of everestmab administered by subcutaneous injection were studied in rats (0.3 and 0.9 mg/kg, n = 6) and male rhesus monkeys (0.1 and 0.3 mg/kg, n = 4). Then, blood samples were collected at pre-dose, 0.5, 1, 2, 4, 8, 24, 36, 48, 96, 144, 168, 192, 216, 240 and 288 h. Plasma samples were obtained and stored at -80 °C. Plasma concentrations levels of everestmab were determined by a sandwich ELISA method using a MonoRab-Rabbit Anti-Camelid VHH Antibody (Genscript, China) as capture antibody and an anti-GLP-1 antibody (Abcam, UK) as detection antibody. Assay sensitivity was estimated to be 15 ng/mL anti-everestmab and drug detection range was between 50 and 5000 ng/mL.
Chronic in vivo studies
Chronic in vivo studies on GK rats
Male diabetic GK rats were assigned into four treatment groups with same average weight and random blood glucose level, and then were injected subcutaneously with everestmab (0.3 and 0.9 mg/kg) and Dulaglutide (1 mg/kg) every 3 days for consecutive 4 weeks. Body weight, food intake, fasting blood glucose levels of GK rats were measured daily. To evaluate the improvement on the glucose tolerance, the GK rats were subjected to OGTT at week 0, 8 and 9 (a week after drug flushing). After the experiment was completed, GK rats were sacrificed, and then the blood samples from each group were obtained and detected blood biochemical indexes, such as the %HbA1c, triglyceride (TG), total cholesterol (TC), low density lipoprotein (LDL) and High density lipoprotein (LDL) values by automatic biochemical analyser.
The pancreatic and liver tissues of GK and DIO rats were also isolated and processed according to the previous reported method [Citation24–26].
Chronic in vivo studies on DIO rats
To evaluate the anti-obesity effects of the everestmab, male DIO rats were assigned and injected with samples similarly as described above. Body weight, food intake, fasting blood glucose levels of GK rats were measured daily. Improvement on the glucose tolerance of DIO rats were assessed at week 0, 8, and 9. In addition, plasma levels of TNF-α, IL-6, fasting insulin, glucose and HOMA value for insulin resistance (IR) of GK rats were also measured using ELISA kits.
Results
Design of of everestmab
As is showed in , everestmab was designed by fusing a GLP-1(A8G) to a half-life prolonged bispecific nanobody consisting of two humanized variable domains of VHH antibodies, obtained from a llama-derived phage library, via two linkers (GGGGSGGGGSGGGGS). One domain interacts with human serum albumin and extends the in vivo lifepans of everestmab, while the other provides the specificity of GLP-1R, a G protein-coupled receptor (GPCR). This design brought a novel long-acting GLP-1R agonist with a minimized molecular weight of about 30 kDa, while a conventional Fc modified or intact antibody usually yields a molecular weight of approximately 60 or 150 kDa, respectively. Once everestmab was subcutaneously administrated, the fusion protein can fastly associate with HSA so that its circulation life can be enhanced. The associative everestmab can be protected from fast elimination by glomerulus and endogenous enzyme digestion. When the fusion protein dissociate from HSA, everestmab will specifically target the GLP-1R via GLP-1R nanobody, and consequentially activate the GLP-1R-mediated signalling pathway.
In vitro characterization of everestmab
The purified everestmab was analyzed with high purity (analyzed by size-exclusion chromatography without aggregation, and correct molecular weight of 34266.9 Da (analyzed by LC-MS, ).
Figure 3. Characterization of novel GLP-1R agonist. (A) HPLC chromatogram. (B) mass spectra analysis of everestmab (34266.81 Da). In vitro biological activities of everestmab. (C) Representative concentration response curves for stimulation of cAMP accumulation by everestmab in CHO cells stablely expressing human GLP-1R. (D, E) Inhibition of the luciferase activity by exendin (9–39), an antagonist of GLP-1R. (F) Insulinotropic activity of everestmab on isolated rat islets. Results are presented as means ± SD (n = 3 each group). ***p<.001, **p<.02, *p<.05, comparing saline to GLP-1R agonists injection.
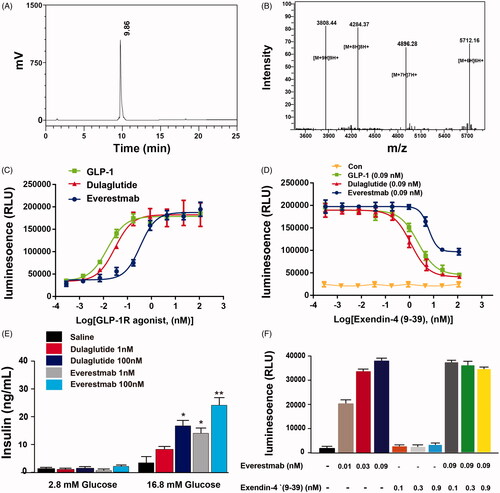
Everestmab specifically bound to stablely expressed human GLP-1R in CHO cell line, and EC50 were 5.09 nM with ∼4 and ∼2.4 times greater than GLP-1 (7-37) and dulaglutide, respectively (). In addition, exendin (9-39), a GLP-1R an antagonist, obviously inhibited luciferase responses induced by GLP-1 (7-37) or dulaglutide, while not significantly changed in everestmab treated group ().
We further evaluated the effects of everestmab on glucose-induced insulin secretion in isolated rat islet. As a result, insulin secretion of rat islets was potently stimulated by everestmab at a concentration of 1 and 100 nM in the extracellular medium at high (16.8 mM) glucose (. In addition, co-incubation the exendin-4 (9-39) and everestmab (0.09 nM) did not significantly inhibit insulin secretion compared with individual everestmab (0.09 nM) ().
Albumin binding abilities and species cross-reactivity of everestmab
Cross-reactivity tests were performed using SPR technology and results demonstrated that the high affinity for albumin were partially preserved in rat and monkey species, but not with mouse or beagle (). Cross-reactivity of the GLP-1R targeting domain was evaluated and was shown to be cross-reactive with mouse (Kd∼1.12 nM), rat (Kd∼21.2 nM) and monkey (Kd∼2.45 nM) species (Figures not shown), but not with beagle, while there are cross-reactivity between HSA targeting nanobody and albumin of rat (KD∼1.09 μM) and monkey (KD∼33.7 nM) only. Overall the rat and monkey species were confirmed enable to be available preclinical animal models.
Glucose-stabilizing capability of everestmab in fasted male GK rats
The glucose-stabilizing capability of everestmab was also evaluated in GK rats using a previous reported method of multiple oral glucose tolerance tests simulating the daily meals [Citation27,Citation28]. The three times glucose administration were performed at 0.5 h, 72 h, and 144 h, respectively, after subcutaneous injection of everestmab (0.1, 0.3, and 0.9 mg/kg) and dulaglutide (1 mg/kg). Results were shown in , blood glucose levels of saline treated ones rapidly increased and peaked over 28 mmoL/L at 0.5 h and then slowly returned to the initial level at least 2 h after each round administration of glucose, while the rats treated with a single dose of everestmab (0.1, 0.3, and 0.9 mg/kg) were below 15 mmol/L in first round OGTT. In addition, the GK rats subcutaneously treated with everestmab (0.1, 0.3 and 0.9 mg/kg) showed control of BGLs in a dose-dependent manner. Furthermore, the sustained BGL control by the single injection of everestmab (0.3 and 0.9 mg/kg) was observed during the entire experiment period (0–146 h) which is comparable to the glucose-lowering content of dulaglutide (1 mg/kg), one of the best-selling weekly GLP-1R agonists.
Figure 5. Multiple OGTTs of the everestmab in GK rats. OGTTs were performed at 0.5 h, 24 h, 72 h, and 144 h after single-dose treatment of everestmab (0.1, 0.3, and 0.9 mg/kg) or Dulaglutide (1 mg/kg). Hypoglycaemic effects of everestmab based on the calculated glucose AUC0–2h, AUC24–26h, AUC72–74h and AUC144–146h values. Results are presented as means ± SD (n = 8 each group). ***p<.001; **p<.02; *p<.05, comparing saline to GLP-1R agonists injection.
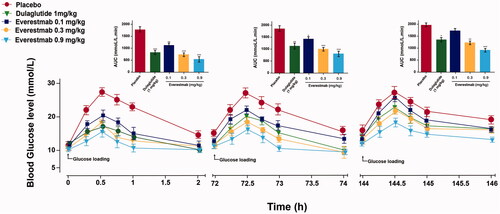
Hypoglycaemic duration tests
To evaluate the hypoglycaemic duration of everestmab, a single subcutaneous injection at two doses (0.3 and 0.9 mg/kg) were performed in diabetic GK rats. According previous reports [Citation11,Citation28], the normal BGL of rats was set below 8 mmol/L and the euglycemic durations under this set were considered as an indicative of its promising role inanti-diabetic treatment. As shown in , saline treated GK rats maintained a hyperglycaemic state (BGL > 20 mmol/L) while those ones administrated with everestmab at 0.3 and 0.9 mg/kg rapidly reached normal BGL values (<8 mmoL/L) and last for almostly 16 and 29 h, respectively, which was comparable to the dulaglutide (1 mg/kg) treated rats (). In addition, everestmab exerted a glucose lowering percentage of 36.5% (0.3 mg/kg) and 59.3% (0.6 mg/kg) for 0–144 h compared with saline group, respectively ().
Pharmacokinetics of everestmab in SD rats and cynomolgus monkeys
After a single subcutaneous administration in SD rats (), the plasma concentration of everestmab slowly reached the peak at ∼12 h, then descended graduately during entire experiment. Similar pattern was also observed in cynomolgus monkeys (). As a result, half-life of everestmab (0.9 mg/kg) in SD rats and cynomolgus monkey was approximately ∼98 h and ∼187 h, respectivey, after a single subcutaneous administration.
Chronic study in GK rats
GK rats received chronic administration of everestmab to assess the in vivo anti-diabetes activity and potential therapeutic scope for 8 weeks. Dulaglutide (1 mg/kg) and everestmab (0.3 and 0.9 mg/kg) were subcutaneously administrated for every 3 days. The body weight and food consumption of diabetic GK rats were carefully detected daily and results showed that everestmab inhibited cumulative food intake and final body weight gain by up to 35% and 25%, respectively, compared with the saline group (). The prior to treatment fasting BGL in everestmab treated GK rats were ∼12.5 mmol/L and lowered towards the ∼7.1 mmol/L levels after 21 days, however the saline treated ones worsened somewhat to ∼21 mmol/L (). Chronic injections of everestmab (0.3 mg/kg, 0.9 mg/kg) also obviously reduced the HbA1c by 1.0 and 0.9%, respectively, at the end of treatment indicating an effectively improved glycemic control (). To determine if the chronic everestmab treatment could effectively improve glucose tolerance of GK rats, OGTT were conducted before, at week 8 (after treatment) and week 9 (after a week washout period). It was observed that no difference was between three group in both BGL and AUC (), however, after 8-weeks treatment, the everestmab treated rats showed better glucose stabilising ability and returned to normal value within 90 min after the administration of glucose, whereas saline treated ones exert a slowly glucose lowering pattern, keeping the BGL in the hyperglycaemic state at least for 120 min (). Before the OGTT at week 9, the GK rats were not treated with everestmab for seven days wash-out and administrated with glucose only (). The excellent glycemic control, especially for the obvious HbA1c reduction and glucose tolerance improvement, ameliorating by the long-term administration of everestmab is indicative of its promising role in clinical application. We further examined the fasting insulin levels and calculated HOMA value for insulin resistance (IR) in DIO rats are presented in . Not surprisingly, HOMA-IR indices of everestmab treated DIO rats were significantly decreased which was comparable to the dulaglutide. Furthermore, there is obvious decrease in serum concentration of TC, TG, LDL-C, AST and ALT in everestmab treated GK rats (). Finally, paraffin sections of GK rat pancreas stainning with H&E were conducted to evaluate the characters of morphology (). As a result, improved islet area and number of the pancreases from everestmab treated GK rats were calculated and showed in ), compared with the saline-treated ones.
Figure 8. The effects of chronic everestmab treatment on GK rats. (A) Body weight gain. (B) Food intake. (C) Fasting BGL level. (D) %HbA1c. Glucose tolerance test in (E) week 1, (F) week 8, (G) week 9 (after a week washout period). Results are presented as means ± SD (n = 8 each group). ***p<.001, **p<.02, *p<.05, comparing saline to GLP-1R agonists injection.
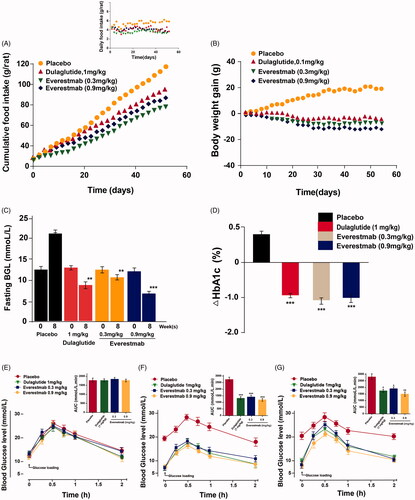
Figure 9. The effects of chronic everestmab treatment on blood biochemistry indexes (A–D) and pancreas HE staining (E) and H&E staining of insulin (F). Results are presented as means ± SD (n = 8 each group). *p<.05; **p<.02; ***p<.001, comparing saline to GLP-1R agonists injection.
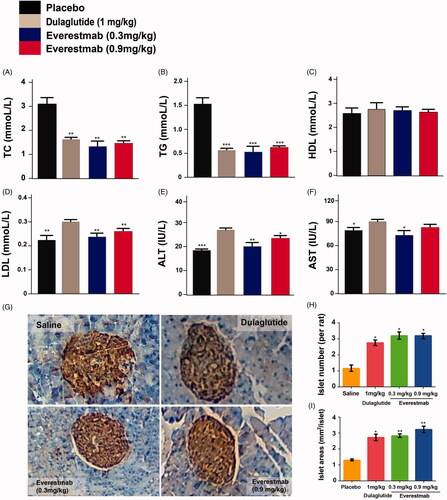
Table 1. Effects of everestmab on insulin levels and HOMA-IR values of GK rats.
Chronic study in DIO rats
In order to furtherly evaluate the anti-obesity potential of long-term treatment of everestmab, an 8-weeks chronic study was conducted by s.c. injection of everestmab (0.9 mg/kg) for every 3 days in DIO rats. As a result, both the food intake and body weight of DIO rats were significantly suppressed among everestmab (0.9 mg/kg) treated group, to a very close content to dulaglutide (1 mg/kg) treated ones, compared with the placebo treatment group (). OGTT were performed at 1 and 8 weeks. Before the treatment of everestmab, there is not obvious difference between the DIO rats among the four groups. It was observed that chronic treatment of Everstmab exert progressively improved effect on glucose tolerance of DIO rats at week 8 (). Glucose metabolism in DIO rats was also improved due to the chronic treatment of everestmab. Injection of everestmab (0.9 mg/kg) for every 3 days significantly decreased fasting blood glucose and insulin levels of DIO rats (). At the end of the chronic treatment, HbA1c, as indirect indicator of cumulative blood glucose level for nearly three months, was significantly lower in everestmab treatment group than the saline treatment ones.
Figure 10. The effects of chronic everestmab treatment on metabolic profiles in DIO rats. (A) Food intake; (B) body weight gain; (C) %fat of body mass; (D) AUC of OGTT; fasting (E) BGL and (F) insulin level; (G) %HbA1c change. Real-time quantitative PCR (qPCR) analysis on the expression of the gene encoding PPARγ (J), TNFα (K), and protein expression of TNFα (H) and IL-6 (I). Results are presented as means ± SD (n = 8 each group). ***p<.001, **p<.02, *p<.05, comparing saline to GLP-1R agonists injection.
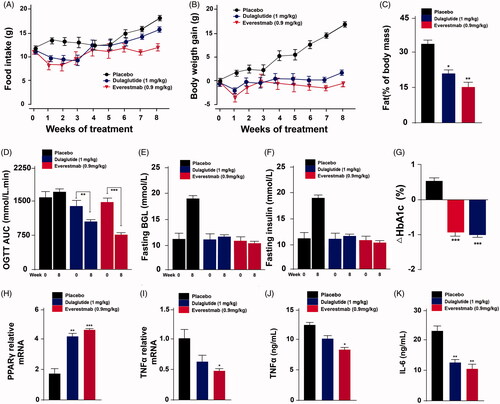
We further examined the expression of PPARγ, a factor in epididymal white adipose tissue regulating the activity of leptin and insulin. The mRNA expression of PPARγ was significantly upregulated in rats after the treatment of everestmab for 8 weeks compared with the control DIO ones, and a little higher than dulaglutide group (). Previous reports showed that in DIO rats, the epididymal white adipose tissue (eWAT) expansion are usually correlated with the pro-inflammatory macrophages accumulation into adipose tissue which contributes to the formation and occurrence of insulin resistance. The effects of everestmab treatment on TNF-α and IL-6 levels in DIO rats are presented in . Nevertheless, everestmab significantly reduced the pro-inflammatory TNF-α and IL-6, and fasting blood insulin level compared with saline treated ones. Together, above results indicated that the treatment of everestmab induce obvious changes in eWAT which is usually consistent with improvement of insulin responsiveness.
Chronic treatment of everestmab obviously decreased the number of lipid droplets, reduced TG levels and weight of liver, indicating a significantly improved ectopic lipid deposition in the liver of DIO rats (). Importantly, chronic treatment with everestmab did not induce hepatocellular toxicity as reflected by the absence of changes in plasma levels of ALP, AST and ALT (). Together, above results indicated that longterm treatment of everestmab prevents ectopic fat deposition in the liver induced by high-fat diet.
Figure 11. Effects of chronic everestmab treatment on DIO rats. (A) Representative microscopy images of liver tissue from DIO rats. (B) Lipid droplet number per HPF; (C) Content of liver triglyceride and (D) liver weight. Plasma levels of (E) ALP, (F) ALT and (G) AST. Results are presented as means ± SD (n = 8 each group). ***p<.001, **p < 0.02, *p<.05, comparing saline to GLP-1R agonists injection.
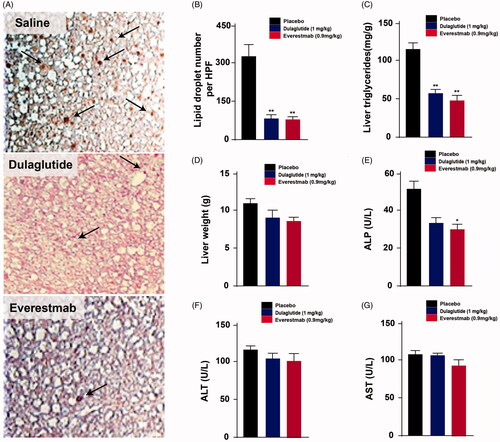
Discussion
In this study, we design and present a novel antidiabetic agent, everestmab, consisting of three domains: a mutated GLP-1 and a bispecific nanobody with hight affinity for GLP-1R, combined with a prolonged lifespans by a targeting human serum albumin nanobody. To date, several short- or long-acting GLP-1R agonists are currently approved and available in clinical application [Citation18,Citation29]. The action mode of GLP-1R agonists have also been clinically validated by the widespread application of exenatide and dulaglutide for glycemic control and improved β-cell function with few side-effects [Citation30–33].
The highly competitive and specific profiles of therapeutic antibodies, including nanobodies, can be ascribed to the protein-binding characters, such as the affinity of antibody for antigen, the excellent selectivity and biological activity [Citation34,Citation35]. Typical characteristics of nanobody, such as smaller size, more robust folding, and better stability, make the construction of the bispecific or difunctional candidates more straightforward [Citation21]. Everestmab, as a completely new fusion protein, is different from all the clinically approved anti-diabetic agents, such as dulaglutide, a GLP-1/IgG4 Fc fusion protein.
The affinity of everestmab for HSA was determined to a KD of 0.83 nM using SPR method. Considering that the high concentration of albumin in human plasma (∼ 600 μM), high affinity of everestmab for HSA can be expected to a predictable extension of the in vivo half-life. In the species cross-reactivity tests, the human albumin-targeting nanobody of everestmab was proved with comparable higher binding affinity for serum albumin of rat and monkey compared with mouse and dog, and thus rat and monkey species were selected as in vivo evaluation models of pharmaceutical effects. To assess the hypoglycaemic effects of everestmab, GK rats were performed with a modified multiple OGTTs. As is illustrated in , the long-acting characteristic of everestmab was significantly improved, which probably due to its reduced enzyme metabolism and renal clearance resulting from the point mutation of native GLP-1 and strong binding affinity for albumin. Importantly, the hypoglycaemic efficacies of everestmab were shown to be in an obviously dosage- and glucose-dependent manner within the range of 0.3–0.9 mg/kg, offering benefits for the future design of preclinical efficacy study and guaranteed drug safety without t the risk of hypoglycaemia.
The in vivo prolonged glucose-lowering profiles of everestmab were furtherly evaluated by hypoglycaemic duration test in GK rats. As illustrated in , BGLs in placebo treatment group maintained a hyperglycaemic state during the entire experiment. In contrast, administration of everestmab (0.3 and 0.9 mg/kg) rapidly normalized blood glucose to a level of 8.35 mmol/L within 2 h and maintained for at least 19.1 h and 26.7 h, respectively, which is comparable to 20.5 h of dulaglutide at the dose of 1 mg/kg. Furthermore, everestmab exerted significantly glucose-lowering effects with glucose lowering percentage of 36.5% for 0.3 mg/kg and 59.3% for 0.9 mg/kg, respectively, during the entire experiment (0–144 h) compared with the saline treated group.
GLP-1 protects the β-cells by binding to GLP-1R which contributes to the decrease of apoptosis and improvement of proliferation, and simultaneously enhancing insulin secretion upon glucose stimulation [Citation36,Citation37] As illustrated in , chronic administration of everestmab effectively suppressed exorbitantly glycemic fluctuations, improved glucose tolerance and insulin resistance. Importantly, everestmab (0.3 and 0.9 mg/kg) obviously improved β-cell function and morphology of pancreatic tissue. HbA1C.
GLP-1 was reported to inhibit gastric emptying and to reduce food intake, water consumption and body weight [Citation38,Citation39]. In the present study, the effect of everestmab on obesity related profiles, such as food intake, body weight gain, composition of body, and blood biochemical indexes, were carefully evaluated in DIO rats, a mostly used obesity model with overweight, insulin resistance and some systemic inflammation reactions. Results show that the chronic treatment of everestmab reduced the food intake of DIO rats by inhibiting the gastric emptying, and also decreased the body weight, improved the insulin resistance and inflammation reactions. Furthermore, every three days injection of everestmab to DIO rats achieved long-term beneficial effects on HbA1c lowering and other blood biochemical indexes.
In summary, everestmab is a novel and bispecific nanbody fusion protein targeting GLP-1R and HSA simultaneously. This study shows that everestmab, exerting the enhanced receptor binding and activitation of biological activity, protracted anti-diabetic characteristics, and chronic beneficial effects, is a potential weekly antidiabetic agent for the treatment of T2DM.
Disclosure statement
No potential conflict of interest was reported by the author(s).
References
- Frier BM. Hypoglycaemia in diabetes mellitus: epidemiology and clinical implications. Nat Rev Endocrinol. 2014;10(12):711–722.
- Wild S, Roglic G, Green A, et al. Global prevalence of diabetes: estimates for the year 2000 and projections for 2030. Diabetes Care. 2004;27(5):1047–1053.
- Saisho Y. β-cell dysfunction: its critical role in prevention and management of type 2 diabetes. World J Diabetes. 2015;6(1):109–124.
- Ali S, Davies MJ, Brady EM, et al. Guidelines for managing diabetes in Ramadan. Diabet Med. 2016;33(10):1315–1329.
- DeFronzo RA. Pharmacologic therapy for type 2 diabetes mellitus. Ann Intern Med. 2000;133(1):73–74.
- Galloway JA. Current trends in diabetes therapy. Trends Pharmacol Sci. 1984;5:33–35.
- Das L, Mohan R, Makaya T. The bid to lose weight: impact of social media on weight perceptions, weight control and diabetes. Curr Diabetes Rev. 2014;10(5):291–297.
- Ross SA, Dzida G, Vora J, et al. Impact of weight gain on outcomes in type 2 diabetes. Curr Med Res Opin. 2011;27(7):1431–1438.
- Ospina NS, Montori VM. Review: in type 2 diabetes, GLP-1 agonists plus basal insulin reduce HbA1c without increasing hypoglycemia. Ann Intern Med. 2015;162(2):JC6.
- Davidson JA. Advances in therapy for type 2 diabetes: GLP-1 receptor agonists and DPP-4 inhibitors. Cleve Clin J Med. 2009;76 Suppl 5(Suppl 5):S28–S38.
- Zhong X, Chen Z, Chen Q, et al. Novel site-specific fatty chain-modified GLP-1 receptor agonist with potent antidiabetic effects. Molecules. 2019;24(4):779.
- Smith EP, An Z, Wagner C, et al. The role of β cell glucagon-like peptide-1 signaling in glucose regulation and response to diabetes drugs. Cell Metab. 2014;19(6):1050–1057.
- Rolin B, Larsen MO, Gotfredsen CF, et al. The long-acting GLP-1 derivative NN2211 ameliorates glycemia and increases beta-cell mass in diabetic mice. Am J Physiol Endocrinol Metab. 2002;283(4):E745–752.
- Mafong DD, Henry RR. Exenatide as a Treatment for Diabetes and Obesity: Implications for Cardiovascular Risk Reduction. Curr Atheroscler Rep. 2008;10(1):55–60.
- Bunck M, Diamant MA, Eliasson B, et al. One-year treatment with exenatide improves beta-cell function, compared with insulin glargine, in metformin-treated type 2 diabetic patients: a randomized, controlled trial. Diabetes Care. 2009;32(5):762–768.
- Schwasinger-Schmidt T, Robbins DC, Williams SJ, et al. Long-term liraglutide treatment is associated with increased insulin content and secretion in β-cells, and a loss of α-cells in ZDF rats ⋆. Pharmacol Res. 2013;76:58–66.
- Retnakaran R, Kramer CK, Choi H, et al. Liraglutide and the preservation of pancreatic β-cell function in early type 2 diabetes: the LIBRA trial. Diabetes Care. 2014;37(12):3270–3278.
- Lotte , Bjerre , Knudsen , et al. The discovery and development of liraglutide and semaglutide. Front Endocrinol. 2019; 10:155.
- Unciti-Broceta JD, Del Castillo T, Soriano M, et al. Novel therapy based on camelid nanobodies. Ther Deliv. 2013;4(10):1321–1336.
- Muyldermans S. Nanobodies: natural single-domain antibodies. Annu Rev Biochem. 2013;82:775–797.
- Van Roy M, Ververken C, Beirnaert E, et al. The preclinical pharmacology of the high affinity anti-IL-6R nanobody® ALX-0061 supports its clinical development in rheumatoid arthritis. Arthritis Res Ther. 2015;17:135.
- Delle H, Noronha IL. Induction of indoleamine 2,3-dioxygenase by gene delivery in allogeneic islets prolongs allograft survival. Am J Transplant. 2010;10(8):1918–1924.
- Koh G, Suh K, Chon S, et al. The effect of cAMP-elevating agents on high glucose-induced apoptosis of isolated islets of rat pancreas. KoMCI. 2004;28:490–500.
- Zhong X, Yang S, Liu T, et al. Engineering a novel protease-based exendin-4 derivative for type 2 antidiabetic therapeutics. Eur J Med Chem. 2018;150:841–850.
- Adeghate E, Ponery AS, Pallot D, et al. Distribution of serotonin and its effect on insulin and glucagon secretion in normal and diabetic pancreatic tissues in rat. Neuro Endocrinol Lett. 1999;20(5):315–322.
- Li L, Gao L, Wang KJ, et al. Knockin of Cre gene at Ins2 locus reveals no cre activity in mouse hypothalamic neurons. Sci Rep. 2016;6:20438.
- Han J, Huang X, Sun LD, et al. Novel fatty chain-modified glucagon-like peptide-1 conjugates with enhanced stability and prolonged in vivo activity. Biochem Pharmacol. 2013;86(2):297–308.
- Sun LD, Huang X, Han J, et al. Site-specific fatty chain-modified exenatide analogs with balanced glucoregulatory activity and prolonged in vivo activity. Biochem Pharmacol. 2016;110–111:80–91.
- Guo XH. The value of short- and long-acting glucagon-like peptide-1 agonists in the management of type 2 diabetes mellitus: experience with exenatide. Curr Med Res Opin. 2016;32(1):61–76.
- Parkes DG, Mace KF, Trautmann ME. Discovery and development of exenatide: the first antidiabetic agent to leverage the multiple benefits of the incretin hormone, GLP-1. Expert Opin Drug Discov. 2013;8(2):219–244.
- Camastra S, Astiarraga B, Tura A, et al. Effect of exenatide on postprandial glucose fluxes, lipolysis, and ss-cell function in non-diabetic, morbidly obese patients. Diabetes Obes Metab. 2017;19(3):412–420.
- Mari A, Del Prato S, Ludvik B, et al. Differential effects of once-weekly glucagon-like peptide-1 receptor agonist dulaglutide and metformin on pancreatic β-cell and insulin sensitivity during a standardized test meal in patients with type 2 diabetes . Diabetes Obes Metab. 2016;18(8):834–839.
- Wysham C, Blevins T, Arakaki R, et al. Efficacy and safety of dulaglutide added onto pioglitazone and metformin versus exenatide in type 2 diabetes in a randomized controlled trial (AWARD-1). Dia Care. 2014;37(8):2159–2167.
- Cortez-Retamozo V, Backmann N, Senter PD, et al. Efficient cancer therapy with a nanobody-based conjugate. Cancer Res. 2004;64(8):2853–2857.
- Van de Broek B, Devoogdt N, D’Hollander A, et al. Specific cell targeting with nanobody conjugated branched gold nanoparticles for photothermal therapy. ACS Nano. 2011;5(6):4319–4328.
- Cases AI, Ohtsuka T, Kimura H, et al. Significance of expression of glucagon-like peptide 1 receptor in pancreatic cancer. Oncol Rep. 2015;34(4):1717–1725.
- Yabe D, Seino Y. Two incretin hormones GLP-1 and GIP: Comparison of their actions in insulin secretion and β cell preservation. Prog Biophys Mol Biol. 2011;107(2):248–256.
- Meena A, Michael B, Knop FK, et al. Do the actions of glucagon-like peptide-1 on gastric emptying, appetite, and food intake involve release of amylin in humans? J Clin Endocrinol Metab. 2010;95(5):2367–2375.
- Chelikani PK, Haver AC, Reidelberger RD. Intravenous infusion of glucagon-like peptide-1 potently inhibits food intake, sham feeding, and gastric emptying in rats. Am J Physiol Regul Integr Comp Physiol. 2005;288(6):R1695–1706.