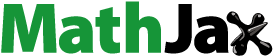
Abstract
This study aimed to increase the solubility of glycyrrhetinic acid (GA) in water and enhance its liver-targeting ability using self-assembling nanomicelles (NMs) based on stearic acid-modified fenugreek gum (FG-C18). The GA/FG-C18 NMs were prepared by an ultrasonication dispersion method. The nanomicelles were spherical particles with a particle size of 198.61 ± 1.58 nm and a zeta potential of −30.12 ± 0.28 mV. The drug loading and encapsulation efficiency were 13.34 ± 0.24% and 80.07 ± 1.44%, respectively. The results of differential scanning calorimetry (DSC) and X-ray powder diffraction (XRD) indicated that GA was successfully encapsulated into the nanomicelles in a molecularly dispersed state. An in vitro release test showed that GA/FG-C18 NMs possessed a slow drug release profile in PBS (pH 7.4) over 200 h. The cytotoxicity assay indicated that GA/FG-C18 NMs showed much higher inhibitory efficacy in HepG2 cells than in MCF-7 cells. Tissue section studies indicated that the accumulation of DiR-loaded FG-C18 nanomicelles in the liver of mice was higher than that of the DiR solution, and the fluorescence intensity decreased over time. GA/FG-C18 NMs showed a larger area under the curve (AUC) and mean residence time (MRT) compared with free GA after intravenous administration in mice. The in vivo studies showed that GA mainly accumulated in the liver after encapsulation by FG-C18 NMs, and the drug concentration was higher than that of free GA. These results suggested that FG-C18 NMs could serve as a potential drug delivery system for targeting GA to liver tissue.
Introduction
Hepatocellular carcinoma (HCC) is the most common type of malignancy worldwide [Citation1]. Currently, chemotherapy has been applied as a major clinical treatment for liver cancer but has resulted in undesirable toxicities in other normal organs because of poor specificity and resistance mechanisms [Citation2]. Nanocarriers have attracted much attention recently due to their remarkable efficiency in targeting the delivery of anti-tumour drugs [Citation3]. Among them, polymeric micelles are novel drug vehicles with unique core-shell structures consisting of self-assembled amphiphilic blocks or grafted copolymers and usually possess a small size of a few nanometres in aqueous media [Citation4]. Poorly water-soluble drugs can be entrapped in the hydrophobic inner cores of the micelles. This special structure could improve the stability and water solubility of the drug, prolong drug circulation time in the bloodstream and enhance drug accumulation in tumour tissues by the enhanced permeability and retention (EPR) effect [Citation5]. Moreover, nanomicelles that were modified by various active targeting ligands could effectively deliver the drug to specific tissues, since these ligands recognise receptors that are overexpressed on the membranes of tumour cells [Citation6,Citation7].
Asialoglycoprotein receptor (ASGP-R), a specific receptor of galactose ligands that is highly expressed on the surfaces of hepatocytes, was a promising target for improving targeting efficiency by clathrin-enabled receptor-mediated endocytosis [Citation8]. Thus, a nanocarrier with galactose residues is critical for targeted drug delivery into the liver. Fenugreek gum (FG) is a natural polysaccharide with non-toxic, biocompatible and biodegradable properties. FG was extracted from the seeds of fenugreek (Trigonella foenum-graecum L.) and consists of a backbone of 1→4 linked β-D mannose units with (1→6) D-galactose units as side chains at a molar ratio of 1:1 [Citation9]. FG has been widely applied in the food field [Citation10], but few reports of FG as a nanocarrier have been made because its high swelling and hydrophilic characteristics resulted in low encapsulation efficiency for poorly water-soluble drugs [Citation11]. In our previous study, FG was successfully modified with the hydrophobic chain of stearic acid (C18) [Citation12]. The obtained amphiphilic derivative showed a favourable self-assembly ability in water and good biocompatibility. The FG-C18 NMs were expected to possess a certain degree of liver-targeting ability for drug delivery.
Glycyrrhetinic acid (GA), a pentacyclic triterpene derivative, is a major active agent extracted from liquorice (the root of Glycyrrhiza uralensis Fisch.) [Citation13]. GA possessed extensive therapeutic effects, such as anti-cancer, anti-inflammatory and anti-viral effects [Citation14]. However, it showed low solubility in water (<0.01 mg/mL in water) and poor oral bioavailability [Citation15]. Therefore, in the present study, GA, as a model drug, was encapsulated into FG-C18 NMs to improve their liver distribution via ASGP-R after i.v. administration. The morphology, particle size, zeta potential and in vitro drug release properties were characterised. An in vitro cytotoxicity assay, a pharmacokinetic study and an in vivo biodistribution study were also performed to evaluate the performance of liver-targeted drug delivery by GA/FG-C18 NMs.
Materials and methods
Materials
Glycyrrhetinic acid (≥98%) was supplied by Nanjing Jingzhu Biotechnology Co. Ltd. (Nanjing, China). Tween 20 was from Shanghai Chemical Reagent Co., Ltd. (Shanghai, China). Pancreatic enzyme was purchased from Sigma-Aldrich (St. Louis, MO, USA). 1, 1′-Dioctadecyl-3, 3, 3′ 3′-tetramethylindotricarbocyanine iodide (DiR) was bought from Amyjet Scientific Inc. (Wuhan, China). Phosphoric acid was obtained from Tianjin Chemical Company (Tianjin, China). Dialysis tubing (molecular weight cut-off 3.4 kDa) was supplied by Greenbird Biological Technology Co., Ltd. (Shanghai, China). Dulbecco’s Modified Eagle’s Medium (DMEM) and foetal calf serum (FCS) were obtained from Thermo Fisher Scientific, Inc. (Waltham, MA, USA). The methyl thiazolyl tetrazolium (MTT) kit was purchased from KeyGEN BioTECH Co., Ltd (Nanjing, China). Both HPLC grade Methanol and Methyl tertiary butyl ether (MTBE) were supplied by Chemical Company (Tianjin, China). Triton x-100 was from HiTo Co., Ltd. (Guangzhou, China). Phosphate-buffered saline (PBS; 0.01 M, pH 7.2–7.6) and other reagents were analytical grade and used as received. Ultrapurified water (Milli-Q, Millipore, USA) was used throughout the experiment.
Preparation of glycyrrhetinic acid-loaded nanomicelles
FG-C18 was successfully synthesised and characterised according to its physicochemical properties in our previous report [Citation12]. GA/FG-C18 NMs were prepared by an ultrasonication dispersion method. Briefly, FG-C18 (25.0 mg) was dissolved in water at a polymer concentration of 2.5 mg/mL. Glycyrrhetinic acid (2.5 mg) was dissolved in dehydrated ethanol (0.5 ml) and then added dropwise to the polymer solution. The mixture was stirred for 24 h at room temperature and then treated using probe-type ultrasonic oscillation (20–25 kHz, Ningbo Scientz Biotechnology Co. Ltd., China) at 200 W for 10 min in an ice-water bath. Finally, the free GA was removed by centrifugation (3500 rpm, 20 min) and filtered through a 0.45 µm syringe filter to obtain the drug-loaded nanomicelles.
Characterisation of GA/FG-C18 NMs
The particle size, zeta potential and polydispersity index (PDI) of GA/FG-C18 NMs were determined using a Zeta Sizer (Nano-ZS90, Malvern Instruments, UK). The morphology of the GA/FG-C18 NMs was observed under a transmission electron microscope (TEM, H-600-4, HITACHI, Japan). The samples were dropped onto a carbon-coated copper grid, stained with 2% (w/v) phosphotungstic acid solution and dried in air before observation.
DSC and XRD measurements
DSC of GA, FG-C18, a physical mixture of the two and GA/FG-C18 NMs powder were carried out using a thermogravimetric analyser (SETSYS-1750 CS, Setaram Instrumentation, Caluire-et-Cuire, France). Heating curves were recorded at a scan rate of 10 °C/min from 30 °C to 450 °C under a dry nitrogen atmosphere.
The crystalline state of the samples was measured by an X-ray powder diffraction instrument (D/MARX2200/PC, Rigaku Co., Tokyo, Japan) using CuKα radiation at 40 mA and 40 kV. Standard runs were performed with a scanning rate of 0.02°/min over a 2θ range of 3–85°.
Drug loading and encapsulation efficiency analysis
Before analysis, the samples were dissolved in methanol during a continuous ultrasonic process and then filtered through a 0.22 μm syringe filter. The GA concentration in the filtrate was quantified by an HPLC method (Agilent 1220, Agilent Technologies, Santa Clara, CA, USA) at 254 nm. The mobile phase was composed of water (adjusted to pH 4.0 with phosphoric acid), methanol and acetonitrile (50:16:34, v/v). The sample was separated by a Phenomenex C18 column (5 µm, 4.6 × 150 mm) at a flow rate of 0.8 ml/min at 35 °C. The drug loading (DL%) and encapsulation efficiency (EE%) were calculated as follows:
(1)
(1)
(2)
(2)
In vitro drug release study
An in vitro release study of GA/FG-C18 NMs was conducted by a dialysis method in PBS (pH 7.4) containing 0.5% (v/v) Tween 20. Briefly, GA/FG-C18 NMs were placed in a dialysis bag (molecular weight cut-off of 3.4 kDa) and immersed in 100 ml of release media at 37 ± 0.5 °C followed by shaking at a continuous speed of 100 rpm. Two millilitre samples were collected at predetermined times (0.5, 1, 2, 3, 4, 6, 8, 12, 24, 36, 48, 72, 96, 120, 146, 192 and 216 h), and the same volume of fresh medium was added immediately. The samples were filtered through a 0.22 µm syringe filter, and the drug content was measured by HPLC. Each sample was analysed in triplicate.
Cytotoxicity test
The cytotoxicity of free GA and GA/FG-C18 NMs was evaluated in ASGP-R-overexpressing HepG2 cells and MCF-7 cells, which served as the negative expression control group [Citation16]. A competitive inhibition experiment was performed by the addition of 0.2 M galactose. In brief, 100 μL of cell suspension at a density of 3 × 104 cells/mL was seeded in each well of a 96-well plate supplemented with 10% FCS and incubated for 24 h in a 5% CO2 atmosphere at 37 °C. Galactose or culture medium was added to pretreat the HepG2 and MCF-7 cells for 24 h of incubation before the addition of the drug. Free GA or an equivalent amount of GA-loaded NMs were diluted by culture medium in the treated groups. The untreated cells were used as the control group. Then, the cells were treated with 20 μL MTT solution at a concentration of 5 mg/mL for 4 h. The medium was aspirated carefully, and 150 μL of DMSO was added into each well with constant shaking for 10 min to lyse the cells. The ratio of cell viability was determined by the MTT test at a wavelength of 490 nm using a Model 550 microplate reader (Bio-Rad, Segrate, Italy). The percentage of the relative cell viability (CV, %) was calculated based on the following equation:
(3)
(3)
where ODtreated and ODcontrol are the absorbance of the treated and control groups, respectively.
Tissue section study
Kunming mice (certificate number SYXK, Ningxia 2011-0001) weighing 22 ± 3 g were purchased from the Animal Experimental Centre of Ningxia Medical University. All experiments were conducted in accordance with the Guidelines for the Care and Use of Laboratory Animals and the Guidelines for the Care of Laboratory Animals at Ningxia Medical University issued by the Animal Experimental Committee.
The mice were deprived of food but given free access to water for one night before administration. The mice were dosed with DiR (a lipophilic NIR-fluorescent cyanine dye) solution or an equivalent amount of DiR/FG-C18 NMs at a dose of 0.25 mg/kg by injection via the tail vein. The dose of the formulation was determined by analysing the content of DiR through a fluorescence spectrophotometer (970CRT, Shanghai, China) at an excitation wavelength of 750 nm and an emission wavelength of 780 nm. The animals were sacrificed at 30 min, 2 h and 4 h after injection. The liver tissues were collected and frozen for 1 h at −80 °C and cut into slices. The obtained sections were fixed with 4% paraformaldehyde for 15 min, washed 3 times with PBS, pH 7.4, for 3 min and then immersed in Triton solution. After washing the sections, the samples were dried in air, stained using DAPI for 10 min and rinsed with PBS. To inhibit fluorescence quenching, the sections were treated with antifade mounting medium, and the tissue slices were observed with a confocal fluorescence microscope (CLSM, Olympus FV1000-IX81, Tokyo, Japan).
Pharmacokinetic and tissue distribution study
Kunming mice were randomly divided into two groups, the free GA and GA/FG-C18 NM groups, and then intravenously injected via the tail vein with GA at a dosage of 1.2 mg/kg. Blood samples of 0.3 ml were collected from the orbital vein, and mice were sacrificed at 0.083, 0.25, 0.5, 1, 2, 4, 6, 8, 12, and 24 h (n = 6 for each time point). Blood samples were immediately spun down at 3000 rpm for 5 min to isolate the plasma from blood cells. All of the main organs, including the heart, liver, spleen, lung and kidney, were harvested. The blood of the heart was removed, and the remaining organs were flushed with normal saline. All organs were weighed and homogenised with normal saline at a concentration of 4 ml/g. Three hundred microliters of tissue homogenate or plasma were mixed with 900 μL MTBE. After centrifugation for 10 min at 12,000 rpm, 600 μL supernatant was transferred to a 1.5 ml test tube and evaporated under nitrogen gas flow. The residue was added to 100 μL of methanol followed by vortexing for 10 min. The mixture was centrifuged at 12,000 rpm for 10 min. Twenty microliters of supernatant were injected into the HPLC system for analysis.
According to the literature [Citation17], the drug targeting efficiency (Te) of GA for the liver can be calculated based on the area under the GA concentration-time curve (AUC0–24 h) for each tissue.
(4)
(4)
where i refers to the target tissue and j refers to other nontargeted tissues.
The relative targeting efficiency (Rd) compared with that of free GA was calculated by using the following equation:
(5)
(5)
Statistical analysis
All results were expressed as the mean and standard deviation. The pharmacokinetic parameters were evaluated by the statistics software (DAS 3.2, Shanghai, China). The statistical analysis was performed by Student’s t-test using SPSS statistics software (SPSS software version 16.0, IBM, Armonk, NY, USA). The p-value of less than .05 was considered statistically significant.
Results and discussion
Preparation and characterisation of GA/FG-C18 NMs
The diagram showing the scheme used for the preparation of GA/FG-C18 is presented in . As shown in , the morphology of the GA/FG-C18 NMs was almost spherical under TEM. The average particle size () was approximately 200 nm with a narrow PDI of less than 0.3. shows that the particles possessed a moderate size distribution. The particle size is a critical parameter for liver-targeted delivery, and it was reported that nanoparticles with diameters of 150–300 nm might be mainly located in the liver and spleen [Citation18]. A small particle size was also helpful for the intravenous administration for particles. The nanomicelles were negatively charged, which may be attributed to the presence of the hydroxyl groups of FG-C18. A high zeta potential (absolute value above 30 mV) may enhance the stability of nanomicelles because of the electrostatic repelling force [Citation19]. The EE value of GA/FG-C18 NMs was approximately 80%, and the DL value was 13.34 ± 0.24%, which was favourable for the encapsulation of water-insoluble drugs.
Figure 1. The scheme used for the preparation of GA/FG-C18 NMs (A), the particle size distribution (B) and the TEM image (C), bar = 200 nm.
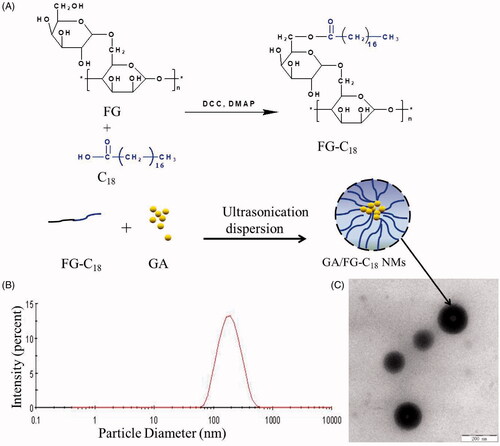
Table 1. Physicochemical properties of GA/FG-C18 nanomicelles.
DSC and XRD measurements
The thermogram curves of the drug, polymer, the physical mixture and drug-loaded micelles were determined by DSC, as shown in . A sharp endothermic peak of GA was observed at 296 °C (a), and FG-C18 showed a wide endothermic peak at 160 °C (b). Both of the characteristic peaks of GA and FG-C18 appeared in the physical mixture (c). In contrast, no melting peaks were observed in the curve of the GA/FG-C18 NMs (d), which was due to the loss of the crystallinity of GA after encapsulation in the FG-C18 nanocarriers.
Figure 2. DSC thermograms (A) and XRD patterns (B) of GA (a); FG-C18 (b); physical mixture of the two (c) and GA/FG-C18 NMs (d).
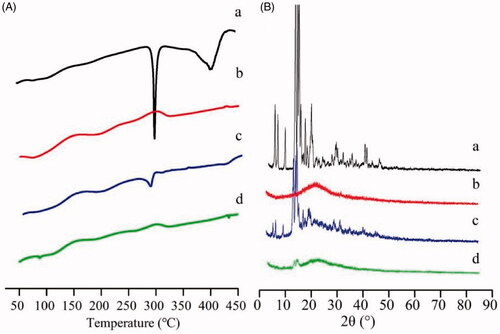
To further support the DSC results, the XRD patterns are presented in . By analysing the XRD spectra, broad peaks were detected for FG-C18 (b). The predominant peaks of crystalline GA (a) in the 2θ range of 12°–35° were detected in the diffractograms of both GA and the physical mixture (c). However, the typical peaks of GA disappeared for the GA/FG-C18 NMs (d). Both the DSC and XRD results showed that GA was highly dispersed within the nanocarriers.
In vitro drug release study
The in vitro drug release profile of the GA/FG-C18 NMs was examined in PBS (pH 7.4) using a dialysis bag method. As shown in , the cumulative release ratio of GA from GA/FG-C18 NMs was above 26% within 24 h, and over 80% of GA was released into the medium within 200 h. However, the drug was rapidly released from the free GA powder into the media, and nearly 80% of GA was released within 1 h. These results indicated that GA/FG-C18 NMs exhibited sustained release behaviour without obvious initial fast release. On the other hand, the slow release behaviour also suggested that GA/FG-C18 NMs were stable in medium, which is necessary for extending the circulation of drug-loaded nanocarriers in vivo to avoid their clearance before their arrival at the targeted tissue [Citation20].
Cytotoxicity test
It was reported that ASGP-R is overexpressed in HepG2 cells but negatively expressed in MCF-7 cells [Citation16]. The in vitro cytotoxicity of GA/FG-C18 NMs and free GA were evaluated in HepG2 and MCF-7 cells for different times. As shown in , the survival rates of cells incubated with GA/FG-C18 NMs and GA decreased over time. The inhibitory effect of GA/FG-C18 NMs on cell proliferation was higher than that of free GA, especially after incubation for 72 h. Compared with MCF-7 cells, GA/FG-C18 NMs also showed significantly higher cytotoxicity towards HepG2 cells. A competitive inhibition experiment was also designed to demonstrate the targetability of GA/FG-C18 NMs to HepG2 cells. The HepG2 cell viability in the presence of GA/FG-C18 NMs was significantly increased after pre-treatment with galactose solution, but pre-treatment with galactose showed almost no influence on MCF-7 cells (because of low ASGP-R expression). The free GA-treated groups did not show obvious differences in cytotoxicity for either cell line whether they were exposed to galactose or not. The GA/FG-C18 NMs showed decreased toxic effects on HepG2 cells after pre-treatment with free galactose because the affinity between free galactose and ASGP-R was higher than that of polysaccharides or polymers containing galactose [Citation16]. Therefore, the binding between GA/FG-C18 NMs and ASGP-R was blocked by free galactose, resulting in low cytotoxicity. These results confirmed that GA/FG-C18 NMs could specifically recognise HepG2 cells by ASGP-R-mediated recognition and showed better selectivity in terms of cytotoxicity towards HepG2 cells in comparison with the free GA.
Figure 4. Cell viability of HepG2 and MCF-7 cells treated with free GA and GA/FG-C18 NMs at 24 h (A), 48 h (B) and 72 h (C). Note: +Gal: with 0.2 M galactose; -Gal: without galactose. Each point is represented as the mean ± standard deviation (n = 6). **p < .01, compared with HepG2 cells pre-treated with galactose and MCF-7 cells without galactose.
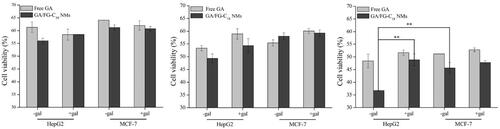
Tissue section study
The DiR/FG-C18 NMs and DiR solution were administered intravenously to mice to evaluate the liver targeting capability of FG-C18 NMs in vivo. As shown in , the fluorescence intensity of the DiR/FG-C18 NMs gradually decreased over time. The liver sections from the nanomicelle group exhibited much stronger fluorescence at 30 min compared with those from the DiR solution group. However, the fluorescence signals were gradually weakened after 4 h and 8 h. This was mainly ascribed to the DiR/FG-C18 NMs, which could be recognised by ASGP-R presented on the membranes of liver cells and then easily retained by liver [Citation21]. However, there was little change in free DiR at all timepoints, indicating that free DiR barely entered into the cells since there was no ligand-medicated recognition, which was consistent with the results of a previous report [Citation22]. These results indicated that FG-C18 NMs were efficient vehicles that could improve the accumulation of DiR in the liver.
Pharmacokinetic and tissue distribution studies
The drug concentration-time curves for both preparations are presented in . The pharmacokinetic parameters are summarised in . Compared with free GA, the GA/FG-C18 NMs possessed a larger AUC, indicating a higher amount of drug in plasma. It could be observed that the MRT for GA/FG-C18 NMs was 37.5 h, which was 1.5 times higher than that of free GA due to slow drug release from the nanoparticles, while the total clearance (CL) of the drug was similar in the free GA group. Moreover, the half-life (t1/2) of the free GA group was 19.7 h, while the half-life (t1/2) of the GA/FG-C18 NMs groups was prolonged to 27 h. These results showed that GA/FG-C18 NMs possessed a longer circulation time in blood in comparison with that of the free GA group. These results may be attributed to the properties of the hydrophobic inner core of the nanomicelles, which provided a reservoir for the sustained release of GA that simultaneously prolonged the presence of the drug in blood [Citation23]. The in vitro release behaviour also indicated this phenomenon.
Figure 6. Plasma concentration-time curve after the intravenous administration of GA/FG-C18 NMs and free GA.
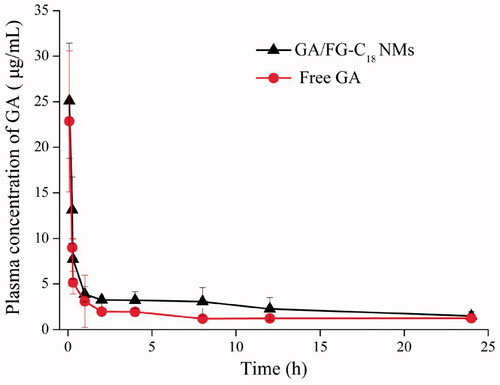
Table 2. Pharmacokinetic parameters after the intravenous administration of GA/FG-C18 NMs and free GA (n = 6).
The distribution of GA in each tissue after intravenous injection with GA/FG-C18 NMs and free GA is shown in . For free GA, it was shown that GA was mainly distributed in the spleen, while the drug content was decreased after the administration of GA/FG-C18 NMs. The levels of GA in the liver were obviously higher than those in other organs, and a maximum concentration of 6 µg/g at 1 h was observed in the GA/FG-C18 NMs group, which was approximately 11-fold higher than that in the free GA group. This observation was in good agreement with the tissue section study that showed that the fluorescence signal was stronger at 30 min than at 4 h and 8 h for the DiR/FG-C18 NMs group because the nanoparticles were metabolised by the liver over time. The GA level in the liver remained relatively high for 8 h following administration of GA/FG-C18 NMs but was rapidly reduced in the free GA group. However, the difference in brain, kidney and brain between the free GA and GA/FG-C18 NMs groups was not significant, indicating that GA/FG-C18 NMs had a low toxic effect on other tissues.
Figure 7. Concentration-time profile of GA/FG-C18 NMs and free GA in heart, liver, spleen, lung, kidney and brain at predetermined times after tail vein i.v. injection.
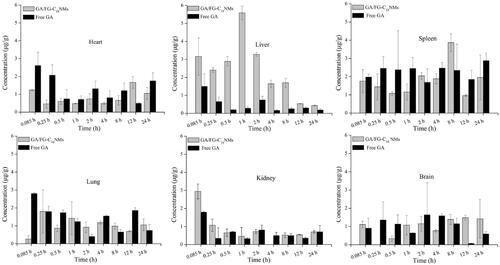
The liver targeting ability of nanomicelles and free GA was estimated according to the Te, and Rd parameters and are presented in . It was observed that the Te of free GA was 40.92% in spleen and 4.31% in liver, whereas this value was altered completely after the encapsulation GA into FG-C18 NMs. The Te in liver for GA/FG-C18 NMs was 44.57% and was 10-fold higher than that for free GA. In addition, the AUC0–24 h of GA/FG-C18 NMs for liver was clearly higher than that for other tissues. These biodistribution results suggested that GA/FG-C18 NMs exhibited excellent liver targeting efficiency. It has been reported that particle sizes ranging between 150 and 300 nm could improve the accumulation of drugs in liver and spleen by the non-specific reticuloendothelial system (RES) uptake-induced hepatic passive targeting effect [Citation18]. However, the hydrophilic outer shell composed of polysaccharides and the high negative charge of the GA/FG-C18 NMs prevented RES uptake [Citation23]. The hepatic target ability of GA/FG-C18 was increased significantly in comparison with that of free GA, and the Rd value for spleen was lower than that for all other organs. Thus, the particle size was not the only critical factor contributing to liver targeting. Moreover, many studies have demonstrated that galactosylated copolymers or grafted polysaccharides containing galactose residues on the surfaces of nanoparticles could enhance the drug level in the liver via the recognition of nanoparticles by ASGP-R [Citation17,Citation24]. The previous cellular uptake experiment [Citation12] and the cytotoxicity assay performed in this study also indicated that GA/FG-C18 NMs were first internalised into HepG2 cells, followed by GA release into the cytoplasm, which inhibited the growth of cells. The specific recognition between ligand and ASGP-R receptor was competed by the galactose solution. Therefore, the liver targeting capacity of GA/FG-C18 NMs was mainly attributed to ASGP-R receptor-mediated endocytosis.
Table 3. The AUC0–24 h and Te of each tissue after intravenous administration of GA/FG-C18 NMs and free GA.
Conclusion
In this study, FG-C18 nanoparticles were fabricated as novel liver targeting nanocarriers to deliver GA by ASGP-R-mediated recognition. The obtained micelles possessed ideal drug loading and encapsulation efficiency with an average size of 200 nm and a negative charge. GA/FG-C18 NMs could be specifically recognised by HepG2 cells via ASGP-R and inhibit the proliferation of HepG2 cells. More importantly, FG-C18 NMs could also effectively deliver more DiR into the liver in comparison with the DiR solution in a tissue section study. We also found that FG-C18 NMs could enhance the accumulation of GA in the liver and that its liver target ability was nearly 10 times higher than that of free GA. These data suggest that FG-C18 NMs have great potential for the targeted delivery of chemotherapy drugs for hepatocellular carcinoma therapy.
Ethics statement
All procedures were approved by the Institutional Animal Ethics Committee (IACE NO. 2013-140) on Animal Experimentation, Ningxia Medical University.
Disclosure statement
No potential conflict of interest was reported by the author(s).
Additional information
Funding
References
- Yim HJ, Sang JS, Um SH. Current management of hepatocellular carcinoma: an eastern perspective. World J Gastroenterol. 2015;21(13):3826–3842.
- Wang Y, Du H, Zhai G. Recent advances in active hepatic targeting drug delivery system. Curr Drug Targets. 2014;15(6):573–599.
- Huang L, Chaurasiya B, Wu D, et al. Versatile redox-sensitive pullulan nanoparticles for enhanced liver targeting and efficient cancer therapy. Nanomedicine. 2018;14(3):1005–1017.
- Liu Y, Sun J, Zhang P, et al. Amphiphilic polysaccharide-hydrophobicized graft polymeric micelles for drug delivery nanosystems. Curr Med Chem. 2011;18(17):2638–2648.
- Brigger I, Dubernet C, Couvreur P. Nanoparticles in cancer therapy and diagnosis. Adv Drug Deliv Rev. 2002;54(5):631–651.
- Cabral H, Kataoka K. Progress of drug-loaded polymeric micelles into clinical studies. J Control Release. 2014;190:465–476.
- Nishiyama N, Kataoka K. Current state, achievements, and future prospects of polymeric micelles as nanocarriers for drug and gene delivery. Pharmacol Ther. 2006;112(3):630–648.
- D’Souza AA, Devarajan PV. Asialoglycoprotein receptor mediated hepatocyte targeting – strategies and applications. J Control Release. 2015;203:126–139.
- Srinivasan K. Fenugreek (Trigonella foenum-graecum): a review of health beneficial physiological effects. Food Rev Int. 2006;22(2):203–224.
- Brummer Y, Cui W, Wang Q. Extraction, purification and physicochemical characterization of fenugreek gum. Food Hydrocolloids. 2003;17(3):229–236.
- Bera H, Mothe S, Maiti S, et al. Carboxymethyl fenugreek galactomannan-gellan gum-calcium silicate composite beads for glimepiride delivery. Int J Biol Macromol. 2018;107(Pt A):604–614.
- Zhou M, Li B, Zhang X, et al. Synthesis and evaluation of hydrophobically modified fenugreek gum for potential hepatic drug delivery. Artif Cells Nanomed Biotechnol. 2019;47(1):1702–1709.
- Sun YQ, Dai CM, Zheng Y, et al. Binding effect of fluorescence labeled glycyrrhetinic acid with GA receptors in hepatocellular carcinoma cells. Life Sci. 2017;188:186–191.
- Khan R, Khan AQ, Lateef A, et al. Glycyrrhizic acid suppresses the development of precancerous lesions via regulating the hyperproliferation, inflammation, angiogenesis and apoptosis in the colon of Wistar rats. PLoS One. 2013;8(2):e56020.
- Lu Y, Li J, Wang G. In vitro and in vivo evaluation of mPEG-PLA modified liposomes loaded glycyrrhetinic acid. Int J Pharm. 2008;356(1–2):274–281.
- Zhang Y, Zhang X, Zhang J, et al. Microfluidic chip for isolation of viable circulating tumor cells of hepatocellular carcinoma for their culture and drug sensitivity assay. Cancer Biol Ther. 2016;17(11):1177–1187.
- Wang W, Zhao X, Hu H, et al. Galactosylated solid lipid nanoparticles with cucurbitacin B improves the liver targetability. Drug Deliv. 2010;17(3):114–122.
- Gaumet M, Vargas A, Gurny R, et al. Nanoparticles for drug delivery: the need for precision in reporting particle size parameters. Eur J Pharm Biopharm. 2008;69(1):1–9.
- Elsabahy M, Wooley KL. Design of polymeric nanoparticles for biomedical delivery applications. Chem Soc Rev. 2012;41(7):2545–2561.
- Wei M, Guo X, Tu L, et al. Lactoferrin-modified PEGylated liposomes loaded with doxorubicin for targeting delivery to hepatocellular carcinoma. Int J Nanomed. 2015;10:5123–5137.
- Jiang SL, Li MY, Hu Y, et al. Multifunctional self-assembled micelles of galactosamine-hyaluronic acid-vitamin E succinate for targeting delivery of norcantharidin to hepatic carcinoma. Carbohydr Polym. 2018;197:194–203.
- Wang X, Gu X, Wang H, et al. Synthesis, characterization and liver targeting evaluation of self-assembled hyaluronic acid nanoparticles functionalized with glycyrrhetinic acid. Eur J Pharm Sci. 2017;96:255–262.
- Wang XD, Gu XQ, Wang HM, et al. Enhanced delivery of doxorubicin to the liver through self-assembled nanoparticles formed via conjugation of glycyrrhetinic acid to the hydroxyl group of hyaluronic acid. Carbohydr Polym. 2018;195(1):170–179.
- Zhang Y, Zhou T, Luo L, et al. Pharmacokinetics, biodistribution and receptor mediated endocytosis of a natural Angelica sinensis polysaccharide. Artif Cells Nanomed Biotechnol. 2018;1:1–10.