Abstract
Curcumin (CM) is a natural polyphenolic compound with multiple biomedical functions. However, clinical applications face more challenges due to its low dissolution rate and poor bioavailability. Micronization is an effective strategy to overcome these drawbacks. Herein, CM nanoparticles (CM NPs, ∼300 nm) were fabricated using solution enhanced dispersion by supercritical CO2 (SEDS). The solubility of CM NPs was remarkably enhanced. Aim to study the effects of micronization on the biological functions of CM, we investigated the antibacterial activity of original CM and CM NPs upon Pseudomonas aeruginosa. In vitro, the minimal inhibitory concentrations (MIC) assay, solid-medium spot assay, growth kinetics assay and morphologic observation using atomic force microscopy (AFM) confirmed that the anti-P. aeruginosa activity of CM NPs was enhanced compared to original CM. Moreover, CM NPs also showed stronger inhibition for adhesion and biofilm formation of P. aeruginosa compared to original CM. Experiments on mice infected with P. aeruginosa showed that CM NPs have a better therapeutic effect than the original CM in vivo. In summary, CM NPs may be a novel and promising therapeutic candidate for bacterial infection.
Introduction
Pseudomonas aeruginosa is the best known and investigated member of the genus Pseudomonas. Due to its high metabolic versatility, P. aeruginosa becomes one of the most relevant pathogens causing human opportunistic infections [Citation1,Citation2]. Despite the continuous expansion of antibacterial agents, the available agents are unable to meet the growing demand for bacterial infections in patients. Although infections caused by P. aeruginosa can be treated with antibiotic agents, there is an urgent need to develop more effective antibacterial agents because of its intrinsic resistance to a wide range of antimicrobial agents [Citation3,Citation4]. Natural products with extensive biological activities, including curcumin, are attractive options for this purpose.
Curcumin (CM), a well-known dietary pigment, derives from Curcuma longa; and it exhibits multiple pharmacological activities, including antibacterial [Citation5], antifungal [Citation6], anticancer [Citation7], anti-inflammatory and antioxidant [Citation8] activities. Importantly, clinical trials have shown that CM is body safely, and oral administration of CM 12 g/d exhibited very little toxicity [Citation9]. However, CM has very low bioavailability and water solubility which makes clinical applications of insoluble CM face more challenges [Citation10]. CM has shown enhanced bioavailability in combination with other compounds or as a formulation. For example, the preparation of non-ionic surfactant/caseinate mixed nanoemulsions [Citation11], the use of LipiSperse technology [Citation12], synthesis of solid lipid nanoparticles (NPs) [Citation13] and so on. In addition, micronization is an effective strategy to overcome these limitations by reducing the size of drugs to increase their surface area [Citation14,Citation15], such like nanoprecipitation [Citation16], sonication [Citation17] and supercritical technique [Citation18–20].
Supercritical technique has advantages, such as mild process, solvent-free residue and environmentally friendly. And solution-enhanced dispersion by supercritical CO2 (SEDS) is an ideal tool for enhancing the solubility and bioavailability of poorly water-soluble drugs [Citation21,Citation22]. A solution containing the solute and supercritical CO2 are atomized via a specially designed coaxial nozzle to promote mass transfer. In SEDS, the morphology and particle size of the solute can be well controlled by adjusting the parameters [Citation23]. Herein, in order to improve the solubility of CM and dispersion in aqueous solution and to increase its biological activity, we fabricated CM nanoparticles (CM NPs) by using the SEDS technique (). In this work, the anti-P. aeruginosa activity of CM NPs was also investigated both in vitro and in vivo.
Materials and methods
Materials and chemicals
Original CM was obtained from Sigma-Aldrich (St. Louis, MO). Hexafluoroisopropanol (HFIP) was purchased from Huifengda Chemical (Jinan, China). Acetone was purchased from ALADDIN (Shanghai, China). Pseudomonas aeruginosa strain PAO1 WT, Escherichia coli ATCC (American type culture collection) 25922 and Staphylococcus aureus ATCC 25923 were provided by S. Lory (Harvard Medical School, Boston, MA).
Fabrication of CM NPs
The process for preparing CM NPs was based on our previous study [Citation5,Citation21], which is shown in . Briefly, original CM powders were dissolved in acetone (the final concentration was 1%). A carbon dioxide flow was kept to maintain the required pressure (22–22.5 MPa) and temperature (31–32.5 °C). Then the solution containing the solute was transported to a high-pressure vessel via a unique coaxial nozzle simultaneously. Finally, CM NPs were collected and dried at 60 °C for 12 h by vacuum drying apparatus.
Physicochemical characterization: SEM, FTIR, DSC and TGA
The surface morphology of original CM and CM NPs were visualized by scanning electron microscopy (SEM) (JEOL, Tokyo, Japan), an aluminium stub with a thin self-adherent carbon film was used to attached the sample, and the samples were then gold sputter-coated. The particle size and distribution of the samples were measured by software from the SEM photographs.
The samples were completely mixed with potassium bromide, and then pressed into a thin tablet. The Fourier transform infra-red spectroscopy (FTIR) spectra (Waltham, MA) for the samples were recorded in transmission mode with the wave number ranging from 3000 to 500 cm−1.
The thermal properties of the samples were measured by a differential scanning calorimeter (DSC) (Munich, Bavaria, German) at a heating rate of 10 °C/min over a temperature range of 25—400 °C in a nitrogen (N2) atmosphere, and a thermo-gravimetric apparatus (TGA) (Munich, Bavaria, German) under a certain condition, which the heating rate was 10 °C/min and N2 flow was maintained at 50 ml/min.
Dissolution rate
The dissolution rate was measured based on our previous studies [Citation21,Citation24]. Briefly, an excess amount (10 mg) of original CM and CM NPs were added into 10 ml of PBS (pH 7.4) with mild agitation at 37 °C. After 24 h dissolution, the concentration of the solutions was detected using a UV spectrometer at 410 nm.
In vitro antibacterial activity against planktonic cells
The minimal inhibitory concentrations (MIC) of tested agents (original CM and CM NPs) against bacteria (Pseudomonas aeruginosa, Escherichia coli and Staphylococcus aureus) was carried out using a modified microdilution method as previously reported [Citation25]. A stock solution of CM NPs was prepared in distilled water to give concentrations in the range of 2–1024 µg/ml. For original CM, a similar aqueous stock solution could not be prepared because CM is completely insoluble in water. Therefore, the stock solution was prepared using DMSO. Hundred microlitres of bacterial suspension (106 cells/ml) was added into 96-well microtitre plates, while 100 µl of tested agents was also added to each well with a final volume of 200 µl, to make sure that final concentrations of tested agents ranged from 1 to 512 µg/ml. The MIC was defined as the lowest concentration that inhibited visible growth by 100%. Inhibition of bacterial growth was determined by measuring the absorbance at 600 nm after an incubation at 37 °C for 24 h.
Solid-medium spot assay
Luria-Bertani agar (LA) containing tested agents (original CM and CM NPs) were prepared for spot assay to evaluate antibacterial activity against P. aeruginosa. Cell suspension was prepared with 109 cells/ml. Of 10 µl cell suspension was diluted and spotted onto prepared LA plates (1 × 107, 1 × 106, 1 × 105 and 1 × 104 cells each spot terminally in order) at 37 °C for 36 h.
Growth kinetics of P. aeruginosa in the presence of antibacterial agents
The effect of tested agents (original CM and CM NPs) on the kinetics of bacterial growth was studied using P. aeruginosa. Briefly, Luria-Bertani (LB) containing MIC/2 concentrations of tested agents was inoculated with bacterial cells to achieve an initial cell density (105 cells/ml) for 30 h at 37 °C. Growth curves were drawn using the optical density (OD600nm) values measured during growth.
Ultrastructural analysis using atomic force microscopy (AFM)
Ultrastructural observation of P. aeruginosa was performed under ambient conditions (air) using Nanosurf CoreAFM (Nanosurf, Liestal, Switzerland) equipped with BudgetSensors Tap190AI-G cantilevers. LB containing MIC/2 concentrations of original CM and CM NPs, respectively, was inoculated with bacterial (109 cells/ml) for 24 h at 37 °C. The cells were harvested, washed three times with 1 × PBS, lyophilized and observed by AFM.
Inhibition of adhesion to pulmonary epithelial cells (PECs)
The adhesion assay was conducted as described previously with minor revision [Citation26]. Briefly, P. aeruginosa was exposed to original CM or CM NPs at their MICs value for 1 h, and were then incubated with MLE-12 cells for another 1 h. The number of bacteria that adhered to PECs was quantified by Colony Formation Units Assay from 100 randomly collected and lysed PECs.
Quantitative measurement of P. aeruginosa biofilms
The dry weights of P. aeruginosa biofilms were investigated based on the method [Citation27] with minor revision. Briefly, strains were grown in LB at 37 °C for 24 h. Bacterial cells were harvested (109 cells/ml) and then treated with tested agents (original CM or CM NPs) at their MICs for 1 h. Of 500 µl mentioned mixture was added in wells of 24 well-plates (14 mm diameter silicone discs inside) and incubated at 37 °C for 2 h. Nonadherent cells were removed and washed gently with PBS, and then the discs were incubated in 1 ml LB for up to 72 h. The discs were gently washed with PBS to remove nonbiofilm cells, and dried to constant weight at 80 °C. The dry weights of biofilms were calculated.
In vivo antibacterial activities
1 × 106 cells of P. aeruginosa were prepared to infect the pulmonary of anaesthetized mice (C57BL/6 mice, 8–12 weeks old), which were randomly assigned to experimental or control groups. Mice were weighed daily and killed when they had become moribund. Organs were homogenized in PBS and used for the measurement of bacterial burdens by serial dilution onto LA plates and normalized to organ weights. Lung tissues were sectioned for histological observations by H&E staining. In addition, drug toxicity was assessed by monitoring the weight of mice with the original CM (2.0 mg/kg), or CM NPs (2.0 mg/kg).
Statistical analysis
All experiments were performed in triplicate or repeated at least three times. Data are presented as changes compared with the control groups. Results are shown as means ± SD. One-way ANOVA (Tukey’s post hoc) or Student’s t test was used for statistical analysis. p Values less than 0.05 were considered significant.
Results and discussion
Surface morphology and particle size
The surface morphology and size of NPs are critical physicochemical properties that affect biomedical functions [Citation28]. The surface morphology and size of the simples produced via SEDS were characterized by SEM (). As can be seen, the original CM powders exhibited irregular shapes and the particle size was much larger than 1 μm (). The vast majority of CM NPs are around 300 nm and show a spherical-like morphology (). It can be seen that CM NPs has been micronized via SEDS process and the size has reached the nanometre level. Obviously, the SEDS process is an effective approach to fabricate CM NPs.
Physicochemical characterization: FTIR, DSC and TGA
FTIR study can reveal the chemical changes by producing an infra-red absorption spectrum. Herein, chemical composition and structure of original CM and CM NPs were investigated and compared by FTIR. shows that the main peak at 1624 cm−1, 1510 cm−1, 1279 cm−1 and 1150 cm−1 found in the FTIR spectra of original CM, which were attributed to the stretching vibrations of the benzene ring, C=C vibrations, aromatic C–O stretching and C–O–C stretching modes, respectively, shifted to 1616 cm−1, 1512 cm−1, 1275 cm−1 and 1138 cm−1 after SEDS process. This result indicated that minor structural changes occurred at the molecular level is consistent with our previous study, which reported such a minor structure change of samples after SEDS process [Citation21]. However, no observable new characteristic peaks were found in the FTIR spectra of CM NPs. Therefore, there was no change in the chemical composition of CM NPs during SEDS process comparing to the original CM.
Figure 3. Physicochemical characterization and solubility evaluation of CM NPs prepared by the SEDS process. FTIR spectra (A), DSC curves (B), TGA (C) curves and solubility (D) of original CM and CM NPs.
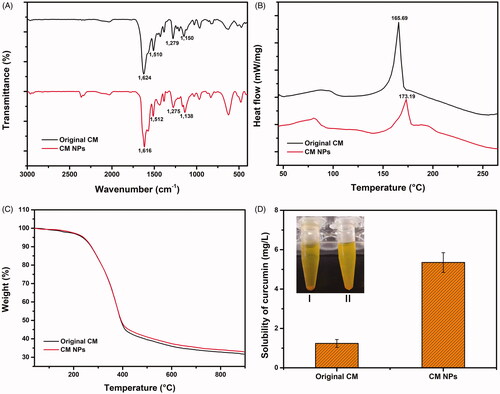
DSC and TGA are widely used to characterize the thermal behaviour of various materials, such as melting and crystallization behaviours. The DSC and TGA curves of samples are shown in . For the original CM, a sharp peak corresponding with the melting point was found near 165 °C [Citation29]. The results from DSC analysis showed original CM and CM NPs displayed a sharp peak at 165.69 and 173.19 °C, respectively. The weak difference can be attributed to the disrupted molecule chains and the decreased crystallization extent caused by the SEDS process. The TG curves that corresponding to the thermal weight losses of the original CM and CM NPs showed no significant difference (). Based on the tangent method, both the initial decomposition temperatures and the 50% decomposition temperatures of CM and CM NPs were the same, being 273.6 and 381.4 °C, respectively. The DSC and TGA results were well consistent with our previous study [Citation21,Citation24].
Solubility evaluation
The dissolution of original CM and CM NPs was investigated; there was significant precipitation in the original CM compared to CM NPs after resting for 24 h (). The solubility of original CM increased from 1.2 to 5.2 mg/l after micronized by using SEDS. The solubility of CM NPs was remarkably enhanced attributing to the higher surface area and the reduced crystallinity [Citation30,Citation31].
Analysis of the antibacterial activity of tested agents in vitro
The MIC was investigated as the lowest concentration that completely inhibited bacterial growth compared with drug-free control. As can be seen from , the MICs of original CM and CM NPs against P. aeruginosa were 256 µg/ml and 128 µg/ml, respectively; this can be explained that NPs of CM exhibited stronger effect than original CM. For S. aureus, CM NPs (MIC = 256 µg/ml) also displayed twice the effect of original CM (MIC = 512 µg/ml). However, both CM and CM NPs against E. coli were> 512 µg/ml. In generally, these results in vitro showed that the tested agents exhibited antibacterial activity against tested strains, especially for P. aeruginosa, but had a weak effect on E. coli. The reasons for lower MIC of CM NPs than original CM may be explained by the fact that NPs could anchor to the cell walls of bacterial to disrupt the membrane structure, and then penetrate inside the cells to break down the structure of cell organelles [Citation5,Citation32].
Table 1. Antibacterial activities of original CM and CM NPs.
Spot assay and growth studies of P. aeruginosa
Pseudomonas aeruginosa, as one of the major opportunistic pathogens of healthcare-associated infections, is associated with substantial high mortality and morbidity rates. Solid-medium spotting assay was applied to evaluate that CM was effective against pathogenic microorganism [Citation33]. In the case of the same bacterial cell implantation, the difference in spots was very obvious, which indicated that P. aeruginosa were more sensitive to CM NPs compared to CM at their respective MIC/2 drug concentrations (). This phenomenon was consistent with the initial MIC result, which the CM NPs displayed twice the effect of original CM for P. Aeruginosa ().
Figure 4. (A) Solid-medium spotting assay. P. aeruginosa strain was tested by spotting decreasing numbers of cells on potato dextrose agar plates containing MIC/2 concentrations of original CM and CM NPs. (B) Growth kinetics of P. aeruginosa in the presence of original CM and CM NPs at their MIC/2 concentrations.
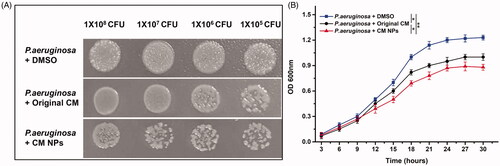
The growth of P. aeruginosa (1 × 105 cells/ml) was also investigated in the liquid culture adding original CM and CM NPs at their MIC/2 concentrations, respectively. shows that the lag phase of P. aeruginosa was almost no difference between original CM and CM NPs, however, the exponential phases was significantly inhibited and delayed by CM NPs compared to original CM, which was consistent with the MIC () and spot assay () results. The spot assay and growth study of P. aeruginosa proved that the antibacterial activity of CM NPs was better than that of original CM.
Ultrastructural analysis of P. aeruginosa cells using AFM
In order to understand the specific mechanism, ultrastructural analysis of P. aeruginosa when exposed to original CM and CM NPs was carried out. The effect of original CM or CM NPs on P. aeruginosa was investigated using AFM imaging. P. aeruginosa, which has not been treated and treated with DMSO, has a rod shape and a full form in the air (). After treated original CM or CM NPs, the morphology of P. aeruginosa has been destroyed and becomes irregular and rough, especially treated with CM NPs (). It is speculated that the antibacterial mechanism of CM may be due to the fact that agents destroy the cell wall of bacteria, causing the cell contents to leak out and the bacteria to die. The damage of CM NPs to the cell wall is more serious, which may be one of the reasons why its antibacterial effect is better than that of the original CM. However, it is unclear that how CM NPs really destroys the cell wall and causes P. aeruginosa death from the AFM results. We also need to use transmission electronic microscopy (TEM) and scanning electronic microscopy (SEM) for further ultrastructure observation.
Figure 5. AFM micrographs of P. aeruginosa (A), P. aeruginosa + DMSO (B), P. aeruginosa + original CM (C) and P. aeruginosa + CM NPs (D), respectively.
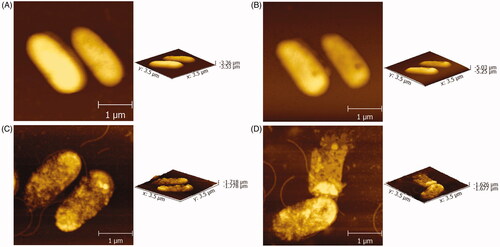
It has become a common method to observe the antibacterial effect of antibacterial agents on pathogenic microorganisms by means of AFM and to explore its antibacterial mechanism [Citation34,Citation35]. In order to systematically elucidate the underlying antibacterial mechanism of PEGylated nano-self-assembly of bacitracin A (PEGylated nano-BA12K) against Streptococcus pneumonia, AFM was used to study the morphological changes of S. pneumonia before and after PEGylated nano-BA12K treatment. It was found that structural changes, partial collapse and even lysis of bacteria were observed after incubation with PEGylated Nano-BA12K [Citation36].
Inhibition of adhesion and biofilm formation
The adhesion of microorganisms to host cells is a prerequisite for colonization and infection [Citation37] and biofilm is one of the key factors that is conducive for infection and drug resistance [Citation38]. Herein, original CM and CM NPs were investigated to further explore their ability to prevent the adhesion to mouse pulmonary epithelial cells (PECs), and inhibit the biofilm formation of P. aeruginosa.
These experiments of adhesion were performed with original CM or CM NPs at their MIC values, respectively. After drug treatment, the number of bacteria in the CM group was about 292 CFU/100 cells, while the number of bacteria in the CM NPs group was about 183 CFU/100 cells, and they were less than the blank control group (421 CFU/100 cells). It can also be seen that both CM and CM NPs were able to prevent the adhesion to PECs, but the CFU of the CM NPs group is lower than that of the CM group ().
Figure 6. (A) Adhesion assay. P. aeruginosa were exposed to original CM and CM NPs at their MIC value for 1 h at 37 °C, and were then incubated with MLE-12 cells for another 1 h at 37 °C. The number of bacteria that adhered to PEC was quantified by CFU assay. (B) Dry weights of P. aeruginosa biofilms were measured over 72 h with adding original CM and CM NPs. (C) Schematic diagram of biofilm formation with no CM NPs (I), and adding CM NPs (II).
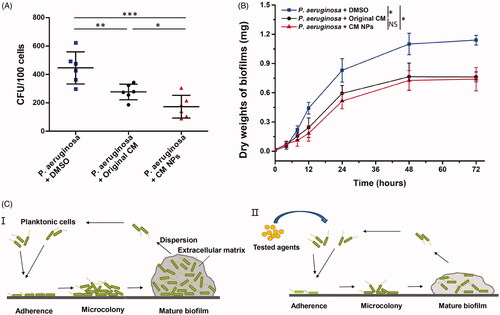
The measurement of dry weight of biofilm is widely used to assess the inhibition of biofilm on drugs [Citation39,Citation40]. shows that the biofilm formation was significantly inhibited in the presence of original CM or CM NPs. CM NPs group had a more significant inhibition of biofilm formation than original CM group at 24 h. However, there was almost no difference in the dry weight of biofilms between original CM and CM NPs when the time reached 72 h. is a good illustration of this general process. Compared to , the test drug in II) acts on the bacteria to prevent adhesion of bacteria to the surface, thereby inhibiting biofilm formation. Our results indicated that CM NPs are a promising antibacterial agent capable of inhibiting the adhesion of P. aeruginosa to PECs, with the potential to improve P. aeruginosa infection outcome in the lungs.
In vivo anti-P. aeruginosa activity test
C57BL/6 mice were infected with P. aeruginosa by intranasal instillation followed by injection (tail) 1 h later with the original CM (0.8 mg/kg) or CM NPs (0.8 mg/kg). The survival rates of mice were monitored over 7 d. The mortality of the mice was improved by an original CM or CM NPs, especially after the injection of CM NPs. Among them, approximately 50% of original CM-treated mice died within 2 d post infection, and all original CM-treated mice had died at 4 d; whereas approximately 20% of CM NPs-treated mice remained alive in 7 d (). Besides, CM NPs protected the mice from severe body weight loss compared with the original CM-treated mice (), which is consistent with survival results. The remaining colony forming units (CFU) in the lungs of moribund mice were also determined to investigate microbial burdens. We found that CM NPs-treated mice showed significantly decreased CFU compared with control or original CM-treated mice (). In order to verify the safety of agents, the toxicity of original CM and CM NPs was assessed using body weight measurement assay. There was no significant difference between the experimental group (tail injection with CM NPs or original CM) and the control group, indicating that the agents were safe at the experimental dose (). Moreover, lung histology analysis showed that CM NPs can protect mice from P. aeruginosa infection-induced alveolar damage and thickening of the respiratory tract mucosa (). Taken together, these results suggested that CM NPs may have a better therapeutic effect than the original CM in vivo.
Figure 7. In vivo anti-P. aeruginosa activity test. (A) Survival curve were monitored in the following 7 d upon P. aeruginosa infection. (B) Body weight was measured 24 h post infection. (C) Lung tissue fungal burdens were tested using CFU assay. (D) MTD (maximum tolerated dose) experiment of original CM (2.0 mg/kg) and CM NPs (2.0 mg/kg).
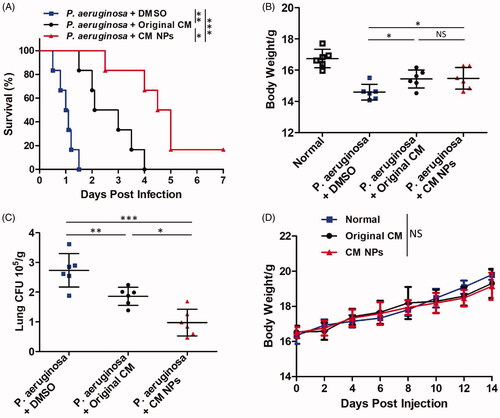
Conclusions
CM is a natural polyphenolic compound with the potential to treat bacterial infections, but is limited in clinical applications due to its low dissolution rate and poor bioavailability. Micronization is an effective strategy to improve the dissolution rate and bioavailability of drugs. Herein, CM NPs were fabricated by using SEDS. CM NPs exhibited better solubility than CM after the SEDS process, and anti-P. aeruginosa activity of CM NPs was enhanced compare to CM in vitro and in vivo. Supercritical derived nano CM NPs can be used as a promising therapeutic candidate for bacterial infection.
Disclosure statement
No potential conflict of interest was reported by the author(s).
Additional information
Funding
References
- Oliver A, Mulet X, Lopez-Causape C, et al. The increasing threat of Pseudomonas aeruginosa high-risk clones. Drug Resist Updat. 2015;21–22:41–59.
- Pourmand A, Mazer-Amirshahi M, Jasani G, et al. Emerging trends in antibiotic resistance: implications for emergency medicine. Am J Emerg Med. 2017;35(8):1172–1176.
- Ali Z, Mumtaz N, Naz SA, et al. Multi-drug resistant pseudomonas aeruginosa: a threat of nosocomial infections in tertiary care hospitals. J Pak Med Assoc. 2015;65(1):12–16.
- Umerska A, Strandh M, Cassisa V, et al. Synergistic effect of combinations containing EDTA and the antimicrobial peptide AA230, an arenicin-3 derivative, on gram-negative bacteria. Biomolecules. 2018;8(4):122.
- Xie MB, Fan DJ, Zhao Z, et al. Nano-curcumin prepared via supercritical: improved anti-bacterial, anti-oxidant and anti-cancer efficacy. Int J Pharm. 2015;496(2):732–740.
- Huang LL, Zhang J, Song TZ, et al. Antifungal curcumin promotes chitin accumulation associated with decreased virulence of Sporothrix schenckii. Int Immunopharmacol. 2016;34:263–270.
- Tian BQ, Wang ZP, Zhao YM, et al. Effects of curcumin on bladder cancer cells and development of urothelial tumors in a rat bladder carcinogenesis model. Cancer Lett. 2008;264(2):299–308.
- Ramsewak RS, DeWitt DL, Nair MG. Cytotoxicity, antioxidant and anti-inflammatory activities of curcumins I-III from Curcuma longa. Phytomedicine. 2000;7(4):303–308.
- Mirzaei H, Shakeri A, Rashidi B, et al. Phytosomal curcumin: a review of pharmacokinetic, experimental and clinical studies. Biomed Pharmacother. 2017;85:102–112.
- Wahlstrom B, Blennow G. A study on the fate of curcumin in the rat. Acta Pharmacol Toxicol. 1978;43(2):86–92.
- Cuomo F, Perugini L, Marconi E, et al. Enhanced curcumin bioavailability through nonionic surfactant/caseinate mixed nanoemulsions. J Food Sci. 2019;84(9):2584–2591.
- Briskey D, Sax A, Mallard AR, et al. Increased bioavailability of curcumin using a novel dispersion technology system (LipiSperse®). Eur J Nutr. 2019;58(5):2087–2097.
- Ban C, Jo M, Park YH, et al. Enhancing the oral bioavailability of curcumin using solid lipid nanoparticles. Food Chem. 2020;302:125328.
- Padrela L, Rodrigues MA, Duarte A, et al. Supercritical carbon dioxide-based technologies for the production of drug nanoparticles/nanocrystals – a comprehensive review. Adv Drug Deliv Rev. 2018;131:22–78.
- Chen BQ, Kankala RK, Chen AZ, et al. Investigation of silk fibroin nanoparticle-decorated poly(l-lactic acid) composite scaffolds for osteoblast growth and differentiation. Int J Nanomedicine. 2017;12:1877–1890.
- Margulis K, Magdassi S, Lee HS, et al. Formation of curcumin nanoparticles by flash nanoprecipitation from emulsions. J Colloid Interface Sci. 2014;434:65–70.
- Zheng Z, Zhang X, Carbo D, et al. Sonication-assisted synthesis of polyelectrolyte-coated curcumin nanoparticles. Langmuir. 2010;26(11):7679–7681.
- Abuzar SM, Hyun SM, Kim JH, et al. Enhancing the solubility and bioavailability of poorly water-soluble drugs using supercritical antisolvent (SAS) process. Int J Pharm. 2018;538(1–2):1–13.
- Cai CF, Liu MH, Li Y, et al. A silica-supported solid dispersion of bifendate using supercritical carbon dioxide method with enhanced dissolution rate and oral bioavailability. Drug Dev Ind Pharm. 2016;42(3):412–417.
- Kankala RK, Zhang YS, Wang SB, et al. Supercritical fluid technology: an emphasis on drug delivery and related biomedical applications. Adv Healthc Mater. 2017;6:1700433 .
- Zhao Z, Xie MB, Li Y, et al. Formation of curcumin nanoparticles via solution-enhanced dispersion by supercritical CO2. Int J Nanomedicine. 2015;10:3171–3181.
- Xie MB, Fan DJ, Chen YF, et al. An implantable and controlled drug-release silk fibroin nanofibrous matrix to advance the treatment of solid tumour cancers. Biomaterials. 2016;103:33–43.
- Chen AZ, Kang YQ, Pu XM, et al. Development of Fe3O4-poly(L-lactide) magnetic microparticles in supercritical CO2. J Colloid Interface Sci. 2009;330(2):317–322.
- Xie MB, Li Y, Zhao Z, et al. Solubility enhancement of curcumin via supercritical CO2 based silk fibroin carrier. J Supercrit Fluids. 2015;103:1–9.
- Dong N, Ma QQ, Shan AS, et al. Strand length-dependent antimicrobial activity and membrane-active mechanism of arginine- and valine-rich β-hairpin-like antimicrobial peptides. Antimicrob Agents Chemother. 2012;56(6):2994–3003.
- Martins CVB, da Silva DL, Neres ATM, et al. Curcumin as a promising antifungal of clinical interest. J Antimicrob Chemother. 2009;63(2):337–339.
- Hawser SP, Douglas LJ. Biofilm formation by Candida species on the surface of catheter materials in vitro. Infect Immun. 1994;62(3):915–921.
- Duse LL, Baghdan E, Pinnapireddy SR, et al. Preparation and characterization of curcumin loaded chitosan nanoparticles for photodynamic therapy. Phys Status Solidi A Appl Mat. 2018;215(5):1700709.
- Xie X, Tao Q, Zou Y, et al. PLGA nanoparticles improve the oral bioavailability of curcumin in rats: characterizations and mechanisms. J Agric Food Chem. 2011;59(17):9280–9289.
- Nagahama K, Kumano T, Oyama N, et al. Curcumisome nanovesicles generated by self-assembly of curcumin amphiphiles toward cancer theranostics. Biomater Sci. 2015;3(12):1566–1578.
- Xie MB, Fan DJ, Li Y, et al. Supercritical carbon dioxide-developed silk fibroin nanoplatform for smart colon cancer therapy. Int J Nanomedicine. 2017;12:7751–7761.
- Basniwal RK, Buttar HS, Jain VK, et al. Curcumin nanoparticles: preparation, characterization, and antimicrobial study. J Agric Food Chem. 2011;59:2056–2061.
- Kumar A, Dhamgaye S, Maurya IK, et al. Curcumin targets cell wall integrity via calcineurin-mediated signaling in Candida albicans. Antimicrob Agents Chemother. 2014;58(1):167–175.
- Jauvert E, Palleau E, Dague E, et al. Directed assembly of living Pseudomonas aeruginosa bacteria on PEI patterns generated by nanoxerography for statistical AFM bioexperiments. ACS Appl Mater Interfaces. 2014;6(23):21230–21236.
- Formosa-Dague C, Duval RE, Dague E. Cell biology of microbes and pharmacology of antimicrobial drugs explored by atomic force microscopy. Semin Cell Dev Biol. 2018;73:165–176.
- Hong W, Liu LP, Zhang ZH, et al. Insights into the antibacterial mechanism of PEGylated nano-bacitracin A against Streptococcus pneumonia: both penicillin-sensitive and penicillin-resistant strains. Int J Nanomedicine. 2018;13:6297–6309.
- de Oliveira HC, Michaloski JS, da Silva JF, et al. Peptides derived from a phage display library inhibit adhesion and protect the host against infection by Paracoccidioides brasiliensis and Paracoccidioides lutzii. Front Pharmacol. 2016;7:509.
- Zhao K, Tseng BS, Beckerman B, et al. Psl trails guide exploration and microcolony formation in Pseudomonas aeruginosa biofilms. Nature. 2013;497(7449):388–391.
- Nobile CJ, Andes DR, Nett JE, et al. Critical role of Bcr1-dependent adhesins in C. albicans biofilm formation in vitro and in vivo. PLOS Pathog. 2006;2(7):e63.
- Nobile CJ, Nett JE, Andes DR, et al. Function of Candida albicans adhesin Hwp1 in biofilm formation. Eukaryotic Cell. 2006;5(10):1604–1610.