Abstract
Graphene and its derivative materials present high potential towards medical and biological applications, including drug delivery and bioimaging, due to their exceptional properties such as thermal conductivity and high specific surface area. The main focus of this work is to review the current development of graphene materials and the derivatives for biocompatible, bioimaging and drug delivery applications. Also, the synthesis methods with variation of graphene nanocomposites and the functionalisation will be further explained. For the graphene approaches, chemical vapour deposition (CVD) is the best-known technique to make high-quality graphene sheet by growth route with mass production. By considering the organic graphene nanocomposites, the biocompatibility and cytotoxic effects against graphene nanocomposites were evaluated for biomedical employments such as high quality bioimaging and effective drug delivery for cancer treatments. For example, graphene oxide incorporated with PEG and loaded with SN 38 for camptothecin analolgue as anticancer drug and revealed high cytotoxicity has an effect of 1000 times better effect than CPT in HCT-116 cells. Their drug delivery ability for both in-vivo and in-vitro applications compared to the controlled drugs such as doxorubicin (DOX) will be discussed accordingly. The graphene and its deriavatives possess some intriguing properties, which will lead to drug delivery due to strong biocompatibility and cyctotoxic effect towards biomedicine applications.
Introduction
The nanoscience field has emerged into a wide range of efficient varieties over the last two decades and the emphasis of nanotechnology/nanomaterial is a circumfluence in various potential areas, like sensor, biomedical, and plenty of useful applications. The progressions in related fields are absolutely due to the ability of synthesising into nanoparticles from various materials, structures, as well as transforming the samples into complex nanoarchitectures [Citation1]. Due to this resason, the monolayer of two-dimensional graphene material with honeycomb lattice formation, which involves the inter-carbon bond length of approximately 0.142 nm is broadly applied for biomedical or nanomedicine applications due to its high conductivity properties [Citation2,Citation3]. The graphene-based materials could definitely help in biomedical applications that allows for the proliferation and maturation of cells, e.g. drug delivery and anticancer therapies. In addition, the materials can also interact with cellular components (e.g. DNA, membranes, or proteins). In the past, graphene/chitosan film has been explored as a scaffold materials in tissue engineering via solution casting method [Citation4,Citation5].
For instance, graphene has been credited for a variety of intriguing properties, such as exceptional thermal conductivity (5000 Wm−1 K−1), rapid electron mobility at ambient condition (250,000 cm2 V−1 s−1), and excellent mechanical properties (Young’s modulus up to 1 TPa) [Citation3,Citation6–8]. Many attempts have been explored over different derivatives and composites of graphene, whereas synthesised Graphene Oxide (GO) without any further functionalisation process has been revealed as a toxic material. However, GO derivatives with well-controlled biocompatibility and cytotoxicity surface coating have been tested with insignificant side effects either in-vitro (human cells) or in-vivo (animals) within the detected dose ranges [Citation6,Citation8].
Recently, functionalised nanographene and its composites have been aroused into biomedical applications due to its exclusive and consequantly enhanced physical and chemical properties. On the other hand, the GO and reduced graphene oxide (rGO) are capable of being functionalised using biocompatible polymers including PEGylated (PEG), which are achievable by both the conjugation (covalent) or surface coating (noncovalent) methods in order to attain more stability of physiological environments. Besides, other covalent polymer types [i.e. poly (L-lysine) (PLL), polyallylamine (PAA), polyvinyl alcohol (PVA)] should be considered for further surface modification with graphene. Still, non-covalent modifications of surface coating techniques for carbon-based nanomaterials are still required for further clarifications.
In view of noncovalent modifications, functional groups are advised to be added without further disruption of efficient network in graphene. In this context, it can be achieved with specific polymeric surfactants, aromatic hydrocarbon as well as compatible biomolecules. Variuos graphene-based bio-sensing terminals have been claimed during the past few years for detecting different biological molecules by using different mechanisms [Citation9–11]. Furthermore, tissue engineering fields determined that the graphene-containing composite or the graphene-coated substrates materials have shown promises regarding the improvements of the growth of human mesenchymal stem cells and hence neuronal differentiation of human neural stem cells [Citation12].
Graphene-based material product, known as a sensitive platform, is commonly used for scanning with biological cells in order to detect the shift mode in terms of electrical potential of human cell membrane [Citation13]. Due to its superior surface area (single sheet arranged with carbon atoms), the rich abundance of functionalised nanoscale rGO-based bio-conjugated nanocomposites has been extensively applied as drug or gene delivery systems. Furthermore, its related derivatives of functionalised graphene provided an excellent tumour ablation therapeutic effects due to its high near-IR (NIR) absorbance [Citation14]. In this case, targeting ligands as an antibody or peptide will be conjugated to functionalise nanographene for specific cell labelling, targeted drug delivery, and in-vivo tumour positron emission tomography (PET) imaging. Also, many methods have been used for the graphene produced in bulk (preference nanocomposite production). The most effective and promising routes is the exfoliation of graphite in a liquid medium to form colloidal suspensions (GO) of few-layered graphene sheets especially applied in clinical or biomedical applications [Citation15–17].
In this particular review, we reported the current focus on graphene as a remarkable platform for raw nanocomposites, which have become the prospects towards controlled and functionalised building blocks of graphene structure. Next, a wide range of synthesis methods for functionalised graphene and its nanocomposites, such as physical and chemical techniques have been summarised accordingly. Next, the controlled functionality of graphene and polymers utilised towards specific biomedical applications arebriefly discussed. Lastly, up-to-date applications of graphene-based materials in tissue engineering, regenerative medicine, biosensors and diagnostics, and antibacterials are also highlighted.
Synthesis methods and the structure of graphene nanocomposite
Various physical and chemical procedures have been established for GO and rGO synthesis in order to obtain high-yield with large scale production and defected-free graphene material. Generally, there are plenty of graphene preparation methods under both main procedures, which are applicable for example epitaxial growth, layer by layer assembly (LLB), chemical exloliation [Citation18], mechanical exfoliation from graphite, chemical vapour deposition (CVD), and chemical reduction method [Citation19,Citation20]. The schematic diagram in shows a brief synthesis of graphene via different techniques for biomedical applications. These synthesis methods of graphene will be briefly discussed in this section.
Figure 1. Schematic diagram of sysnthesis of GO/rGO/graphene and its outstanding properties that helps for biomedical applications.
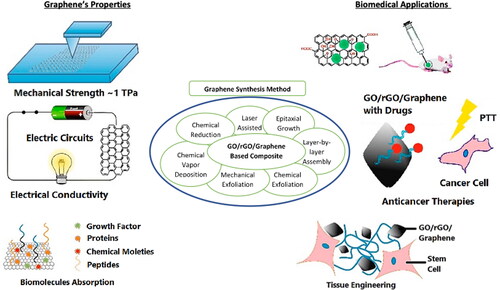
Expitaxial growth method
Epitaxial growth is considered a common technique that deposits graphene on a silicon carbide (SiC) substrate and hence results in the decomposition of SiC and the form of graphene layers. Therefore, the silicon is desorbed off the surface with highly pure defect-free graphene sheets [Citation21]. In fact, this process provides numerous advantages on the finalised product, from the metal substrate to the dielectric type substrate. The formation of graphene film is totally free from purity without transition or transportation, controlled initiation, and product growth, which can also be achieved through the right position of selected substrate. demonstrates the process flow of graphene via epitaxial growth at a high temperature. It could be monitored that the SiC is etched away under high temperature and the carbon atom is recombined after the non-carbon atoms are evaporated.
Figure 2. The schematic process flow of epitaxial growth method of graphene [Citation22]. Copyright (2018) MDPI.
![Figure 2. The schematic process flow of epitaxial growth method of graphene [Citation22]. Copyright (2018) MDPI.](/cms/asset/df7175de-c526-4531-8bde-c696b7e2b169/ianb_a_1817052_f0002_c.jpg)
Layer by layer assembly (LLB)
The LLB has been extensively employed for self-assembly processes in order to produce graphene nanocomposite thin films. The resulting composite structure is expected to produce well-aligned components as illustrated in [Citation23].
Figure 3. Dispersion of (a) reduced graphite oxide (r-GO) [left] and PB- functionalized graphene (PB′-G) [right] in water with concentration of 0.1 mg.mL−1 and (b) The synthesis route of amphiphilic reduced graphene oxide (PEG.OPE.rGO) from coil-rod-coil conjugated triblock copolymer [Citation24–26]. Copyright (2013) RSC Publishing.
![Figure 3. Dispersion of (a) reduced graphite oxide (r-GO) [left] and PB- functionalized graphene (PB′-G) [right] in water with concentration of 0.1 mg.mL−1 and (b) The synthesis route of amphiphilic reduced graphene oxide (PEG.OPE.rGO) from coil-rod-coil conjugated triblock copolymer [Citation24–26]. Copyright (2013) RSC Publishing.](/cms/asset/8537bcb1-b10e-40cf-9fcc-37f7eecf677d/ianb_a_1817052_f0003_c.jpg)
An example of modified graphene nanocomposite is provided in . The bovine serum albumin (BSA) is used as an adhesive agents in the preparation of graphene-nanoparticles composites. It describes that almost each type of nanocarbon family, graphene or its further composition with others metals or polymers could benefit the stimulation of scaffolds cells in the artificial way. Indeed, the physicochemical properties of graphene-based materials intimately influence the surface interactions of individual cells and cell populations [Citation27–29].
Figure 4. The preparation steps of graphene nanoparticles from BSA [Citation19]. Copyright (2013) RSC Publishing.
![Figure 4. The preparation steps of graphene nanoparticles from BSA [Citation19]. Copyright (2013) RSC Publishing.](/cms/asset/7faa6119-be07-4c81-b598-a2e86ee597da/ianb_a_1817052_f0004_c.jpg)
Chemical exfoliation method
Chemical exfoliation, a scalable technique, towards the production of reducing of GO (rGO) from GO, commonly sustains the solution at temperature between the range of 85–100 °C (moisture) [Citation30]. GO is an exfoliated form of graphite, which comprises various oxygen-containing functionalities and is usually synthesised via ultrasonication methods in deionised water condition. Furthermore, it has the capability to produce large quantities of GO in low-cast manner. For the experimental observe point, the conversion of GO to rGO is ordinarily identified by the colour change from bright yellow to dark colour, which indicates high contain of oxidised functional groups [Citation17]. In this reduction process, several reducing agents were used to reduce the GO into rGO, such as thiourea organosulfur compound, hydrazine hydrate, alkali metal borohydrides, hydrohalic acid solvent, and alkali metals [Citation31]. Such reducing agents are reported to be producing significant deoxygenate degrees of rGO. Nevertheless, the possibility of conversion from GO into rGO is very high when in thermal at high temperature (= >1000 °C) in a specific tube furnace in the presence of inert gas. Accordingly, it could create pressure towards graphene sheets in order to reduce the van der Waals force. However, of the large energy demand is one of the major drawbacks for thermal reduction process [Citation32]. Fortunately, a one-step facile route to form rGO from GO suspension on substrate has been identified by electrochemical reduction method, as known as cyclic voltammetry (CV) principle [Citation33].
Mechanical exfoliation method
The mechanical exfoliation is the simplest way to produce graphene or few-layered graphene (FLG) from the graphite source and considered as one of the most promising ways to obtain large-scale production at low cost [Citation34]. Azhar et al. produced the FLG from two sources, which are pencil lead and graphite disc, and found that graphite disc is able to produce high yield, higher aspect ratio, and also more homogenous compared with graphite lead [Citation35]. The overall experimental flow is displayed in .
Figure 5. Mechanical exfoliation process over glass surface with graphite lead (left) and graphite disc (right) for FLG production [Citation35]. Copyright (2019) MDPI.
![Figure 5. Mechanical exfoliation process over glass surface with graphite lead (left) and graphite disc (right) for FLG production [Citation35]. Copyright (2019) MDPI.](/cms/asset/b2795805-2bf0-4d41-bc5b-f3682f8fc6b9/ianb_a_1817052_f0005_c.jpg)
Chemical vapour deposition (CVD) method
Chemical vapour deposition (CVD) is one of the most popular way and widely used in forming monolayered graphene and producing relatively high-quality graphene in large scale production. Typically, CVD is a simple chemical reaction process that uses carbon source (gas, liquid, or solid) and decomposes at high-temperature condition, in which resulted in carbon atoms in the metal surface which eventually is graphene membrane [Citation36]. Besides, the deposition method comprises homogeneous gas-phase reaction and chemical reaction.
Chemical reduction method
Chemical reduction of GO is often synthesised at room temperature and it could be defined as chemical reagent reduction, solvothermal reduction, photocatalyst reduction, and electrochemical reduction [Citation37]. It is considered one of the conventional ways to produce graphene or rGO in mass quantities with low-cost production. Theoretically, chemical exfoliation of graphite flakes causes weak van der Waals force attraction between the layers. The chemical exfoliation processes, such as the Conventional Hummer’s method, Modified Hummer’s method, and Improved Hummer’s method are invented to produce GO with large size or aspect ratio, high yield, and less toxic gasses [Citation15,Citation38,Citation39].
Laser-assisted method
The laser-assisted method is one of the recent advanced techniques and could deliver efficient rGO films [Citation40]. It is well prepared under laser beam radiation for the in-situ and nonthermal (at room tempeature) reduction condition of solution-based GO. In this context, this method will remain silimar charateristics as the conventional way in terms of lattice and physical properties. In addition, sheet resistance is much lower compared to other syntesis method at ∼700 Ω/sq [Citation41]. demonstrates the GO and graphene produced with different synthetic approaches, advantages/disadvantages, limitation, and its respective biomedical applications.
Table 1. Summaries of GO/graphene formation based on variety of approaches.
Graphene-based nanocomposites for biomedical applications
Notably, nanocomposites structured contributed their greatest parts on biomedical systems. Acrylic-based nano-conjuctant systems have found great interest in polymer- and protein-based nanotechnologies platform for cancer theranostics efficiency [Citation42]. Conprehensively, Zheng and his collabrators have looked at pH-sensitive polymers like poly(acrylic acid), poly(N,N-dialkyl aminoethyl methacrylates) or poly(methacrylic acid), drug delivery systems, stimuli-responsive hydrogels, and even dental restorative materials [Citation23]. In fact, the progress of resin-based filling composites technology has been reformed towards clinical science over the past three decades. For example, the nanocomposites for amalgams compound in dental field possess better aesthetic properties, enhanced safety guard matter, and provided reasonably satisfactory clinical results [Citation43]. Over the years, the properties and performance of these materials have been improved by modifications according to their formulation like new green/organic monomers and fillers approaches [Citation3].
Graphene nanocomposite indicates the substantial enhancement in their multifunctional aspect at very low loading, in comparison to conventional composites and their materials. The introduction of graphene not only manufactures the finalised production to become lighter but also simplify makes the processing stronger for various multifunctional biomedical applications [Citation27]. Recently, the antibacterial activity affecting the type of water-dispersible using graphene-based against E. coli bacteria with minimisedcytotoxicity results on humans was intensively studied [Citation44]. It was reviewed that the GO paper could be effectively used in the field of biotechnology and environmental remediations [Citation28]. Besides, the cytotoxicity effects against GO or graphene material particularly human erythrocytes that measured the mitochondrial activity for skin fibroblasts were under control by the physicochemical properties and were demonstrated [Citation45]. The results proved that GO was more attractive in haemolytic activity than graphene when produced in extremely small size. However, the GO-coated chitosan sample demonstrated that the haemolytic activity disappeared and indicated the biocompatibility of composite for human erythrocytes. It was also concluded that materials in terms of particle size, surface charge, purity, and oxygen threshold are crucial for the biological and toxicological responses [Citation29].
In addition, several types of graphene materials included graphite, graphite oxide, GO, and rGO were prepared and tested against E. coli bacteria and toxicity effects. The intensity of toxicity was measured by membrane stress and oxidative signals. They found that GO had less toxic than others samples (rGO > graphite > graphite oxide) [Citation46]. While exploring the fabrication and design for antimicrobial application, graphene-poly-N vinyl carbazole (PVK) nanocomposites were biased towards a broad array of bacteria. This showed significant microbial inhibition and toxicity results (>80%) [Citation47]. After that, the PVK-GO nanocomposite was explored for toxicity test and investigated under planktonic microbial cells, metallidurans B. subtilis E. coli, C. and R. opacus, mammalian fibroblast cells (NIH 3T3), and biofilms, respectively. The results revealed that PVK-GO possessed stronger antimicrobial effect rather than raw GO. It was also indicated that the PVK-GO exhibited neutral preferences on fibroblast cells, leading to further investigations on biomedical applications [Citation48].
Some researchers illustrated that the Mn-ferrite (MnFe2O4) decorated GO nanocomposites for clinical applications [Citation49]. It was revealed that the magnetic properties of the ferrites could be effectively used as an superlative hyperthermia and T2 contrast MRI agent. Also, this PEG nanocomposite showed brilliant biocompatibility match with human cells [Citation50]. Recently, hydroxyapatite-GO nanocomposite was synthesised as a biocompatible prosthetic agent. It was revealed that the (300) and (002) orientation plane hydroxyapatite nanorods in the graphene matrix play an essential role in sustaining the mechanical properties of nanocomposite [Citation51]. A brief schematic view of GO nanocomposite applications is presented in .
Figure 6. Graphene-based nanomaterials and its derivatives for various biomedical usage purposes [Citation28]. Copyright (2014) Elsevier.
![Figure 6. Graphene-based nanomaterials and its derivatives for various biomedical usage purposes [Citation28]. Copyright (2014) Elsevier.](/cms/asset/47f3b55f-4c08-4751-b72c-4dd87a433832/ianb_a_1817052_f0006_c.jpg)
Bioimaging
Although the quantum yield of nGO–PEG on fluorescence effects is relatively low, the cell imaging of intrinsic fluorescence is still capable to workout at IR-A region because of the extremely low auto-fluorescence background of biological tissues. For cell imaging of nGO–PEG, the near-infra-red (NIR) photoluminescence (PL) indicated that GO and nGO–PEG presented fluorescence broadly from visible to infra-red (IR) range [Citation52]. This shows that the IR-A part was lately identified for cell imaging with diminutive backgrounds [Citation53]. Therefore, rituxan as an anti-CD20 antibody (recombinant antibodies) was conjugated to nGO–PEG for B cell-specific diversion of glucose labelling covalently. The cell imaging appeared by spotting the IR-A PL from nGO–PEG in the range within 1100–2200 nm with InGaAs detection by 658 nm laser stimulation. Meanwhile Raji B-cells showed obvious fluorescent signals (), the negative CEM T-cells showed minor signals and nGO–PEG was labelled with a NIR fluorescent dyes by lumiprobe, since the conjugation of Cyanine-7 (Cy7) dye molecules was kept at the terminus of PEG chains in order to avoid the substantial fluorescent quenching [Citation54].
Figure 7. Fluorescence imaging with functionalised nano-graphene for in-vitro and in-vivo (a) anti-CD20 antibody conjugated NGO–PEG in order to aim human B lymphocyte or Burkitt’s lymphoma (Raji B cells) and (b) CD-20 positive with Raji B cells (left) and CD-20 negative with CEM cells (right) under PL inspection [Citation55]. Copyright (2008) Springer.
![Figure 7. Fluorescence imaging with functionalised nano-graphene for in-vitro and in-vivo (a) anti-CD20 antibody conjugated NGO–PEG in order to aim human B lymphocyte or Burkitt’s lymphoma (Raji B cells) and (b) CD-20 positive with Raji B cells (left) and CD-20 negative with CEM cells (right) under PL inspection [Citation55]. Copyright (2008) Springer.](/cms/asset/33c65b20-48d7-4e2f-8d3b-7452aa6141f6/ianb_a_1817052_f0007_c.jpg)
For improved results, Mice et al. reported that injected with different tumour markers including nGO–PEG–Cy7 under Maestro EX setup for in-vivo fluorescence imaging has capability to enhance the detected signals of the tumour over the time (refer ) [Citation56]. These results suggested that the efficient passive targeting of nGO–PEG is detected in numerous xenograft tumour types.
Figure 8. (a) A graphical of NGO–PEG labelled with Cy7 dye molecules structure and (b) Fluorescence in-vivo imaging of mice test with 4T1, KB, and U87MG tumours accordingly at various time periods after containing NGO–PEG–Cy7 dye moleculs is injected [Citation57]. Copyright (2004) American Association for Cancer Research.
![Figure 8. (a) A graphical of NGO–PEG labelled with Cy7 dye molecules structure and (b) Fluorescence in-vivo imaging of mice test with 4T1, KB, and U87MG tumours accordingly at various time periods after containing NGO–PEG–Cy7 dye moleculs is injected [Citation57]. Copyright (2004) American Association for Cancer Research.](/cms/asset/ce875abb-f9c2-4e62-b5de-258de9bbe645/ianb_a_1817052_f0008_c.jpg)
Gelatine exhibited suitable chemico‐physical properties for scaffolds production for nerve tissue engineering as tabulated in . These properties were mostly biocompatible, biodegradable, in-vivo resorbable, and toxic free processing. Gelatine also revealed that graphene and GO as composites can be applied for cellular imaging and drug delivery purposes [Citation62]. Besides, these composites could also be assisted from exfoliated natural graphite in water-dispersible solutions [Citation63]. Wan et al. reported the production of GO nanocomposites with gelatine by putting it under test on a solution mixed with several GO contents [Citation64]. It was discovered that 1 wt% GO sample exhibited higher tensile strength, Young's modulus, and energy when breaking the gelatine than other samples. In addition, it did not only response as an effective reinforcement filler but also to the biological activator in hydrophilic biopolymers field especially in biomedical applications [Citation65–67].
Table 2. Summarised of graphene-based composites for in tissue engineering and its related biomedical applications.
Drug delivery systems
Widely applied GO for drug delivery systems normally consists of a few multilayers with ∼1–3 layers, thickess of ∼1–2 nm, and particle size ranging from a few nanometre scale up to hundreds of nanometres. Furthermore, it has several unique properties with eligible biocompatibility and stability in physiological solubility, especially in loading drugs/genes by chemical conjugation or physisorption response [Citation68]. Also, the reactive functional groups of carboxyl and hydroxy (–COOH and –OH) on its surface would facilitate conjugation with various systems and tremendously impart the multi-functional GO for diverse biological and clinical medical imaging applications [Citation69–71]. Nonetheless, natural polymers incorporated graphene not only provides biocompatible wise but also assists in reducing the toxic effects of specimen. For example, gelatine works as a functionalising agent in the DOX deposited graphene nanosheets [Citation72]. Since gelatine-graphene has a large surface area and high P interactions, thus this phenomena leads to enhancing the drug loading capacity. A few years ago, gelatine–Graphene–DOX complex had revealed that high toxicity towards MCF-7 could stimulate the responsive nanocarrier system for the specific DOX targeted drug delivery into the cytosol [Citation73].
Next, nano GO (NGO) sheets were modified with PEG and loaded with SN 38 for camptothecin (CPT) analogue as an anticancer drug [Citation74]. The NGO–PEG–SN38 complex presented a suitable water solubility level and retained the high vitro cyctoxicity of SN38. Results also revealed that high cytotoxicity effect was almost 1000 times better than CPT in HCT-116 cells [Citation75]. In another study, Sun et al. reported that the targeted delivery of rituxan (CD20+ antibody) drugs conjugated PEG–NGO was achieved [Citation72]. Furthermore, DOX was inserted onto a PEG–NGO conjugate using non-covalent p–p stacking. It also indicated that the GO was pH dependent when the drug was released from GO’s surface. In other words, it was treated like the probable pH-controlled drug release. Accordingly, the achieved pH-sensitive drug release activity was indicated with others GO-based drug delivery systems as well [Citation76]. In order to identify multiple drug therapies in cancer treatment (DOX and CPT), two anticancer agents were loaded with folic acid while SO3H conjugated GO in the sense of p–p stacking in a controllable action [Citation77]. The introduction of GO with folic acid ligand in drug loading system was presented with precise targeting activity and a relatively higher cytotoxicity than MCF-7 cells in human breast cancer cells with the presence of folic acid receptors. It is noticeable that a remarkable higher cytotoxic effect was observed on the composite rather than raw GO loaded self-governing route.
Furthermore, an efficient route with the functionalised graphene sheets (GS) with well established poly(N-isopropyl acrylamide) (PNIPAM) composite was conducted [Citation78]. Throughout this investigation, PNIPAM-GS was generally applied making poor water-soluble anticancer drug, CPT, and the discharging of it from PNIPAM–GS–CPT complex in water condition and PBS at 37 °C. The change of phase transition from hydrophilic to hydrophobic was at 33 °C, suggesting it to be lower than the PNIPAM homopolymer at 37.8 °C. However, an optimised CPT loading at ∼18.5% was achieved, which attributed to its p–p stacking and hydrophobic behaviour that reacted against graphene sheets and demonstrated a higher anticancer activity than CPT specimen. This showed that the PNIPAM–GS sheets were practically non-toxic for human’s health and possessed an outstanding capability of CPT interaction.
Recently, an in-vivo application of heptapeptide ATWLPPR (A7R) glioma-targeted drug delivery system based on GO was recently developed [Citation79,Citation80]. DOX was loaded onto chlorotoxin-conjugated GO (CTX–GO containing DOX with CTX–GO in ratio of 570 mg/g) and pertained high efficiency under non-covalent interactions [Citation81]. Since DOX is pH a dependent state with introduced GO, the CTX–GO/DOX interceded the highest demonstration rate for glioma cells. Besides, the conjugation of chlorotoxin improved DOX glioma cells accumulation. The overall morphologies of cellular circulation and DOX, GO/DOX, and CTX–GO/DOX are illustrated in . The cellular circulation of CTX-GO/DOX and DOX, GO/DOX, in C6 cells with the comparable concentration of DOX (0.5 mg/ml) was in incubation for 24 h. Wang et al. introduced a novel nanoparticles based on the galactosylated chitosan/GO/doxorubicin (GC–GO–DOX) drug for therapeutic cancer treatment [Citation82]. The working drug delivery system is majorly contributed by GC and GO carriers. In this context, the drug delivery could be delivered at a maximum rate of 1.08 mg/mg (drug/polymer). The GC–GO–DOX drug remained stable under physiological conditions, whereas the drug was responsive when released at a low pH environment (tumour environment). Cells uptook the experiment on a cell propagation analysis in which the nanoparticles had a cytotoxicity rate in the sequence of HepG2 > SMMC-7721 > CS–GO–DOX. Other than the CS–GO–DOX complexity, HepG2 and SMMC-7721 exhibited higher fluorescence intensity in tumour cells.
Figure 9. Surface morphologies of CTX-GO/DOX and DOX, GO/DOX, in C6 cells with the comparable concentration of DOX (0.5 mg/ml) for 24 h of incubation time period [Citation81]. Copyright (2016) Royal Society of Chemistry.
![Figure 9. Surface morphologies of CTX-GO/DOX and DOX, GO/DOX, in C6 cells with the comparable concentration of DOX (0.5 mg/ml) for 24 h of incubation time period [Citation81]. Copyright (2016) Royal Society of Chemistry.](/cms/asset/5fb8eff9-a1ad-4b5d-a2a9-4b3e591e1468/ianb_a_1817052_f0009_b.jpg)
Recently, an innovative GO-based carrier was introduced with chitosan (CHI) powder to enhance the biocompatibility and conjugated with hyaluronic acid (HA) to form GO–CHI–HA complex under CD44 target ligands. In addition, this complex has the capability to determine more specific tumour cells recognition and tremendously increase their efficiency as anti-tumour drug delivery system [Citation83]. Then, the resultant final product of GO–CHI–HA was incorporated with SNX-2112 as an anti-cancer drug (Hsp90 inhibitor). Apparently, the overall released amount rate of SNX-2112 was significantly active in acidic as compared to the physiological condition. Moreover, the low concentration of GO–CHI–HA brings a small impact towards lysis and coagulation of red blood cells (RBCs). Furthermore, GO–CHI–HA/SNX-2112 had been proven in killing A549 cells while having lower cytotoxicity against the normal human bronchial NHBE cells (Epithelial). In addition, there are several available nanostructures that have their biocompatibilities complex with GO or graphene to improve anticancer activities, such as Rh2-treated GO (GO-Rh2), lysine-treated highly porous graphene (Gr-Lys), arginine-treated Gr (Gr-Arg), Rh2-treated Gr-Lys (Gr-Lys-Rh2) and Rh2-treated Gr-Arg (Gr-Arg-Rh2) [Citation69]. Most of the nanostructures listed could act as an anti-tumour drug agent, where the binding of drugs is irresolvable after being invaded.
In other implementation, graphene-reinforced curcumin composites contributing to anti-tumour effects were stated [Citation84]. Particularly, graphene quantum dots (GQDs) were treated as the most convenient derivatives of GO for composites, as they composed of bundles of curcumin and operated as bioprobes for tumour imaging. In this moment, the graphene is required, allowing oxygen-containing functional groups to boost the drug loading rate. Noticeably, GQDs revealed an excellent drug-loading capacity up to ∼40,800 mg/g for cancer therapy treatment [Citation85,Citation86]. This study proves that curcumin has the authority to control the growth of tumour. In other words, the tumour size is experiencing a rapid growth with free curcumin sample (refer ). In a sharp contrast, both double-oxidised of GO, DGO with curcumin and GQD-curcumin were used to test the inhibition of the tumour growth on mice surviving more than 14 days. There were no observable increases in tumour size (refer and ). Wang et al. [Citation53] have developed a novel NIR light-responsive indocyanine green/DOX/graphene oxide with polyamidoamine-pluronic F68 (ICG/DOX/GO-PPF68) nanocomposite. This nanocomposite is conjugated by F68 and GO via diselenide bond encapsulated with the NIR photosensitiser ICG and chemotherapeutic DOX drug as payloads. The nanocomposite detected escapred lysosomal, controlled drug release, and nuclear trafficking of DOX inside multi-drug resistant (MDR) MCF-7/ADR cells under NIR light. Interestingly, this nanocomposite effectively addressed down-regulated ABCB1 gene P-glycoprotein of MCF-7/ADR cells that attributed to significant cytotoxicity effect. In-vivo anti-tumour study demonstrated an effective accumulation and superior therapeutic efficacy of this multifunctional nanocomposite, corresponding to MCF-7/ADR tumours and becoming a promising method for clinical treatment of MDR cancer. Before the end of this session, the variable studies related to drug delivery of DOX using GO or graphene-based composites were summarised accordingly in .
Figure 10. (a) Relative tumour volumes of mice (n = 5, 6) treated with several specimens (b). Growth of tumour weights in mice body (n = 6) [Citation84]. Copyright (2009) Royal Society of Chemistry.
![Figure 10. (a) Relative tumour volumes of mice (n = 5, 6) treated with several specimens (b). Growth of tumour weights in mice body (n = 6) [Citation84]. Copyright (2009) Royal Society of Chemistry.](/cms/asset/bd509314-47f9-4a73-8bb1-c3bdfee08aa2/ianb_a_1817052_f0010_c.jpg)
Figure 11. After 14 days of mice body observation treated with PBS, DGO, GQD, DGO Cur, GQD–Cur, and Cur specimens [Citation71]. Copyright (2014) Nature.
![Figure 11. After 14 days of mice body observation treated with PBS, DGO, GQD, DGO Cur, GQD–Cur, and Cur specimens [Citation71]. Copyright (2014) Nature.](/cms/asset/857a1f4d-68f1-4ff9-b75b-d81d76a2a9e4/ianb_a_1817052_f0011_c.jpg)
Figure 12. In-vivo imaging inspection of tumour bearing mice after injecting 10 mg/kg dosages of GQDs and GQD–Cur [Citation71]. Copyright (2014) Nature.
![Figure 12. In-vivo imaging inspection of tumour bearing mice after injecting 10 mg/kg dosages of GQDs and GQD–Cur [Citation71]. Copyright (2014) Nature.](/cms/asset/f76ae907-d2ff-428f-a9fc-0ce0215139c6/ianb_a_1817052_f0012_c.jpg)
Table 3. Graphene and its derivatives for drugs and genes delivery applications.
Tissue engineering
Tissue engineering fabricates biological substitutes and safe materials for repairing or replacing the damaged human tissue or scaffold. Therefore, designing a perfect composite that includes all the desired properties is important. Today, graphene nanomaterial is actively involved in the medical and tissue engineering fields due to its potential in reacting with other biomolecules, such as DNA, enzymes, proteins and peptides for regenerative medicine. Recently, graphene and its byproducts have been attractred novelly for invention and applied in biocompatible systems. In a recent study, CHI/Poly(vinyl alcohol) (PVA)/GO composite nanofibre was modified via electrospinning method [Citation98]. The results presented that 6 wt% CHI/PVA/GO was more environmentally friendly against ATDC5 cells growth compared to 4 wt% CHI/PVA/GO composite. Due to this reason, they credited that 6 wt% CHI/PVA/GO nanofibre could be further investigated for artificial cartilage [Citation98]. In another study, GO-enriched PCL fibrous scaffolds were well fabricated using the electrospinning method [Citation99]. During the porosity measurement test, the result of the entire samples was higher than 95%, which resembles the perfect answer for tissue regeneration and the fibres adsorbing more protein while incorporated with GO. Also, regarding in-vitro cell studies, it was demonstrated that the adhesion and propagation of MG-63 cells were noticeably enhanced with added graphene oxide nanosheets. Therefore, graphene reinforced polycaprolactone (GO–PCL) biocomposites revealed that scaffolds could enhance physico-mechanical properties and achieve significantly improved biological characteristics in comparison to bulk PCL via electrospun fibres in bone tissue engineering.
Recently, Nasrin et al. found that graphene had the ability to strengthen the biological properties of scaffold as the latest therapy for bone regeneration [Citation100]. They also summarised that graphene nanocomposites could lead the development of scaffolds for specific target organ/tissues. The involvement of both GO and graphene-based on their attachment, growth, proliferation, and differentiation mostly due to the aromatic scaffold nature [Citation101,Citation102]. Nair et al. found that the contain of 0.5 and 1.0 wt% of GO nanoflakes will enhanced the mechanical strength and osteogenic differentiation when incorporated with gelatine-hydroxyapatite (GHA) matrix after the freeze-dried process [Citation103]. Apart from this, ∼50–60% of GO was unrestricted in linear manner when scaffolds immersed in phosphate-buffered saline for 60 days.
Besides scaffolds for bone tissue regeneration, graphene and its deriviates also had the biocompatibility and cytotoxicity against their bioactive molecules for the dentistry field. In the current technology, dental implants are the best substitutes for missing teeth. However, the fibrous tissue issue led to failure of implantation [Citation104]. Some researchers tried to modify the implant surfaces in order to obtain better osseointegration. Until recently, graphene was invented as implant coating for hard tissue for the purpose of bone regeneration acceleration [Citation105].
Biosensors and diagnostics
Basic biosensor upgrading with graphene, it will be greatly beneficial to the sensor functions with additional superior properties in improving surface-to-volume ratio, optical characteristic, density, thermal conductivity, electrical conductivity as well as enhancing their carrier mobility [Citation106–108]. Since graphene provides large surface area properties, then it could improve the surface loading and create a great platform for biomolecules compatibility. In addition, graphene would also be a greater and beneficial conductivity, since it exhibits narrow band gap to allow electron charge carriers flow rapidly between biomolecules complex and the electrode surface. The graphene-based biosensors structure is shown in .
Figure 13. Graphene integrated biosensors structure [Citation109]. Copyright (2018) Springer.
![Figure 13. Graphene integrated biosensors structure [Citation109]. Copyright (2018) Springer.](/cms/asset/807d3ede-2ca9-4529-9105-a217c680ab14/ianb_a_1817052_f0013_c.jpg)
However, graphene not only assists in biosensors but also involves in diagnostics. The graphene could rapidly lead biomolecules for disease diagnosis and therapy detection. In contrast, biosensors can be used for diagnostic glucose, glutamate, cholesterol, and haemoglobin with minimal pain. Furthermore, graphene will also enable the biosensors to detect smaller/lighter markers easily. In other words, comparable sensors reinforced graphene, which eventually shortens the procedure with fast detection and more sensitivity with a low-cost process. Numerous biologically active Field Effect Transistor (BioFET) are designed using graphene-based technology [Citation110–112]. However, graphene-based materials have also been exploited for nanoelectronic biosensors devices, like DNA sensors (detection of nucleobases and nucleotides), gas sensors (detection of different gases), PH sensors, environmental contamination sensors, and strain and pressure sensors.
Antibacterial
Graphene and its byproducts exhibited an superior antibacterial agent against numerous bacteria types. These materials have attained to antibacterial properties towards combined mechanisms of bacterial membrane perturbation with graphene due to its sharp edges and oxidative stress induction. Recent advances in nanomaterials have allowed room for applications by utilising graphene as an antibacterial agent in biomedical sciences work [Citation113–117].
Liu and collaborators recently studied in the wound recovery and infection control investigation using graphene oxide-quaternary ammonium (GO-QAS) nanocomposites [Citation118]. The GO-QAS nanocomposite exhibits excellent biocompatibility and synergistic antibacterial ability against multi-drug bacteria resistance on both of mechanical membrane perturbation and oxidative stress induction. Notably, GO-QAS has a promising efficient of re-epithelialisation and highly granulation tissue formation towards wounds infections and injuries and accelerating the recovery process. Undeniably, GO-QAS nanocomposite probably could be synthesised in antimicrobial agent for wounds treatments and also antibacterial wound dressing.
Karthikeyan et al. recently reported the antibacterial activity in graphene after being incorporated with GO nanosheets synthesised by chemical reduction process [Citation119]. They studied the Gram-positive/negative bacteria and E. coli by using GO nanosheets. Among these bacteria, the sample of Gram-positive bacteria is the most effective and sensitive with GO nanosheets. They claimed that GO could be an antibacterial agent in the medical field in future. Besides, Federica and her collaborators also focussed their study on antibacterial and cytotocity factor by using functionalised graphene [Citation120]. These were used to synthesise GO chemically and electrochemically (GO(LiCl)) route and the bacterial against Gram-positive Staphylococcus aureus, Gram-negative, and E. coli microorganisms. They reported that GO(LiCl) possesses more antimicrobial activity and suggests its application for anticancer drugs in future research.
Conclusion and future prospects
The interest in graphene has been increasing during the past few years, with reference to a novel substitute for old biomedical applications. A huge numbers of reports indicates that graphene exhibits superior abilities combined with other green materials and converted into graphene nanocomposite, as biodegradability, biocompatibility, cytotoxic effects among others. Typically, the bio-functionality of graphene is comprehensively considered in clinical fields, such as drugs delivery and bio-imaging applications. Notably, graphene has been reviewed that the efficient targeted drugs delivery and pH-dependent methods could be widely applied in cancer theraphies, such as doxorubicin and other cancer therapy drugs. This further indicates that graphene and its derivatives will be further developed in biomedical fields in the view of drug loading capacity, solubility, and given its effectiveness in cell membrane penetration. However, functionalised of graphene via physical and chemical routes is also facing the possibilities and challenges of tailoring either the properties of nanostructures for in-vitro human cells or in-vivo living organism. In fact, graphene is officially listed under hazardous materials and recognised by the Scientific Committee on Emerging and Newly Identified Health Risks (SCENIHR); considered as a toxic product. The statement on some physicochemical information, like shape, size dimension, agglomeration rate, lateral dimension, and also layer thickness based on different types of graphene (GO, rGO, graphane, graphone, graphyne, graphdiyne, fluorographene and their doped versions) still remains unclear on evaluating toxicity. The contradictory outcomes of synthesis route against biological still lack of reproducibility potential. Yet, there are many rooms for improvement since it does not have a standard protocol or regulatory guidelines to avoid the conflicts. It is suggested that graphene derivatives should go through safety validations before being considered for biomedical use.
Disclosure statement
The authors declare that there is no conflict of interest regarding the publication of this paper.
Additional information
Funding
References
- Mousavi SM, Soroshnia S, Hashemi SA, et al. Graphene nano-ribbon based high potential and efficiency for DNA, cancer therapy and drug delivery applications. Drug Metab Rev. 2019;51(1):91–104.
- Slonczewski J, Weiss P. Band structure of graphite. Phys Rev. 1958;109(2):272–279.
- Zakeri A, Kouhbanani MAJ, Beheshtkhoo N, et al. Polyethylenimine-based nanocarriers in co-delivery of drug and gene: a developing horizon. Nano Rev Exp. 2018;9(1):1488497
- Fan H, Wang L, Zhao K, et al. Fabrication, mechanical properties, and biocompatibility of graphene-reinforced chitosan composites. Biomacromolecules. 2010;11(9):2345–2351.
- Singh DP, Herrera CE, Singh B, et al. Graphene oxide: an efficient material and recent approach for biotechnological and biomedical applications. Mater Sci Eng C Mater Biol Appl. 2018;86:173–197.
- Mousavi SM, Hashemi SA, Ghasemi Y, et al. Green synthesis of silver nanoparticles toward bio and medical applications: review study. Artif Cells Nanomed Biotechnol. 2018;46(sup3):S855–S872.
- Ravanshad R, Karimi Zadeh A, Amani AM, et al. Application of nanoparticles in cancer detection by Raman scattering based techniques. Nano Rev Exp. 2018;9(1):1373551.
- Mousavi S, Hashemi S, Amani A. Modification of polypropylene-starch blend by eggshell nano-particle, EVA and maleic anhydride to improve biodegradability and thermal properties. Int J Chem Sci. 2017;15(4):225.
- Anghileri E, Marconi S, Pignatelli A, et al. Neuronal differentiation potential of human adipose-derived mesenchymal stem cells. Stem Cells Dev. 2008;17(5):909–916.
- Bai RG, et al. Graphene: a versatile platform for nanotheranostics and tissue engineering. Prog Mater Sci. 2018;91:24–69.
- Muazim K, Hussain Z. Graphene oxide – a platform towards theranostics. Mater Sci Eng C Mater Biol Appl. 2017;76:1274–1288.
- Kumar R, Singh RK, Singh DP. Natural and waste hydrocarbon precursors for the synthesis of carbon based nanomaterials: graphene and CNTs. Renewable Sustainable Energy Rev. 2016;58:976–1006.
- Li Y, Gao C, Long R, et al. Materials today chemistry. Mater Today. 2019;11:197–e216.
- Robinson JT, Tabakman SM, Liang Y, et al. Ultrasmall reduced graphene oxide with high near-infrared absorbance for photothermal therapy. J Am Chem Soc. 2011;133(17):6825–6831.
- Low FW, Lai CW, Hamid SBA. Easy preparation of ultrathin reduced graphene oxide sheets at a high stirring speed. Ceram Int. 2015;41(4):5798–5806.
- Park S, Ruoff RS. Chemical methods for the production of graphenes. Nat Nanotechnol. 2009;4(4):217–224.
- Low FW, Lai CW, Abd Hamid SB. Facile synthesis of high quality graphene oxide from graphite flakes using improved Hummer's Technique. J Nanosci Nanotechnol. 2015;15(9):6769–6773.
- Singh RK, Kumar R, Singh DP. Graphene oxide: strategies for synthesis, reduction and frontier applications. RSC Adv. 2016;6(69):64993–65011.
- Chang H, Wu H. Graphene-based nanocomposites: preparation, functionalization, and energy and environmental applications. Energy Environ Sci. 2013;6(12):3483–3507.
- Dhand V, Rhee KY, Ju Kim H, et al. A comprehensive review of graphene nanocomposites: research status and trends. J Nanomater. 2013;2013:1–14.
- de Heer WA, Berger C, Wu X, et al. Epitaxial graphene. Solid State Commun. 2007;143(1-2):92–100.
- Tan H, Wang D, Guo Y. Thermal growth of graphene: a review. Coatings. 2018;8(1):40.
- Zheng Q, Ip WH, Lin X, et al. Transparent conductive films consisting of ultralarge graphene sheets produced by Langmuir-Blodgett assembly. Acs Nano. 2011;5(7):6039–6051.
- Xu Y, Bai H, Lu G, et al. Flexible graphene films via the filtration of water-soluble noncovalent functionalized graphene sheets. J Am Chem Soc. 2008;130(18):5856–5857.
- Qi X, Pu K-Y, Li H, et al. Amphiphilic graphene composites. Angew Chem Int Ed Engl. 2010;49(49):9426–9429.
- Choi E-Y, Han TH, Hong J, et al. Noncovalent functionalization of graphene with end-functional polymers. J Mater Chem. 2010;20(10):1907–1912.
- Dubey N, Bentini R, Islam I, et al. Graphene: a versatile carbon-based material for bone tissue engineering. Stem Cells Int. 2015;2015:804213.
- Goenka S, Sant V, Sant S. Graphene-based nanomaterials for drug delivery and tissue engineering. J Control Release. 2014;173:75–88.
- Teradal NL, Jelinek R. Carbon nanomaterials in biological studies and biomedicine. Adv Healthcare Mater. 2017;6(17):1700574.
- Piñas JAV, Andrade TS, Oliveira AT, et al. Production of reduced graphene oxide platelets from graphite flakes using the fenton reaction as an alternative to harmful oxidizing agents. J Nanomater. 2019;2019:1–8.,
- Chua CK, Pumera M. Chemical reduction of graphene oxide: a synthetic chemistry viewpoint. Chem Soc Rev. 2014;43(1):291–312.
- Zhang H-B, Wang J-W, Yan Q, et al. Vacuum-assisted synthesis of graphene from thermal exfoliation and reduction of graphite oxide. J Mater Chem. 2011;21(14):5392–5397.
- Zhang Y, Hao H, Wang L. Effect of morphology and defect density on electron transfer of electrochemically reduced graphene oxide. Appl Surf Sci. 2016;390:385–392.
- Yi M, Shen Z. A review on mechanical exfoliation for the scalable production of graphene. J Mater Chem A. 2015;3(22):11700–11715.
- Pirzado A, Le Normand F, Romero T, et al. Few-layer graphene from mechanical exfoliation of graphite-based materials: structure-dependent characteristics. ChemEngineering. 2019;3(2):37.
- Wang C, Vinodgopal K, Dai G-P. Large-area synthesis and growth mechanism of graphene by chemical vapor deposition. In: Mandracci P, editor. Chemical vapor deposition for nanotechnology. London: IntechOpen; 2018.
- El-Hallag IS, El-Nahass MN, Youssry SM, et al. Facile in-situ simultaneous electrochemical reduction and deposition of reduced graphene oxide embedded palladium nanoparticles as high performance electrode materials for supercapacitor with excellent rate capability. Electrochim Acta. 2019;314:124–134.
- Zaaba NI, Foo KL, Hashim U, et al. Synthesis of graphene oxide using modified hummers method: solvent influence. Procedia Eng. 2017;184:469–477.
- Chong SW, Lai CW, Hamid SBA. Green preparation of reduced graphene oxide using a natural reducing agent. Ceram Int. 2015;41(8):9505–9513.
- Kumar R, Singh RK, Singh DP, et al. Laser-assisted synthesis, reduction and micro-patterning of graphene: recent progress and applications. Coord Chem Rev. 2017;342:34–79.
- Kymakis E, Petridis C, Anthopoulos TD, et al. Laser-assisted reduction of graphene oxide for flexible, large-area optoelectronics. IEEE J Select Topics Quantum Electron. 2014;20(1):106–115.
- Zhu X, et al. Polymer-and protein-based nanotechnologies for cancer theranostics. In: Chen X, Wong S, editors. Cancer theranostics. Amsterdam: Elsevier; 2014. p. 419–436.
- Ma M, Chen H, Chen Y, et al. Au capped magnetic core/mesoporous silica shell nanoparticles for combined photothermo-/chemo-therapy and multimodal imaging. Biomaterials. 2012;33(3):989–998.
- Liu S, Zeng TH, Hofmann M, et al. Antibacterial activity of graphite, graphite oxide, graphene oxide, and reduced graphene oxide: membrane and oxidative stress. ACS Nano. 2011;5(9):6971–6980.
- Liao K-H, Lin Y-S, Macosko CW, et al. Cytotoxicity of graphene oxide and graphene in human erythrocytes and skin fibroblasts. ACS Appl Mater Interfaces. 2011;3(7):2607–2615.
- Shin SR, Li Y-C, Jang HL, et al. Graphene-based materials for tissue engineering. Adv Drug Deliv Rev. 2016;105(Pt B):255–274.
- Ma X, Tao H, Yang K, et al. A functionalized graphene oxide-iron oxide nanocomposite for magnetically targeted drug delivery, photothermal therapy, and magnetic resonance imaging. Nano Res. 2012;5(3):199–212.
- Shen A-J, Li D-L, Cai X-J, et al. Multifunctional nanocomposite based on graphene oxide for in vitro hepatocarcinoma diagnosis and treatment. J Biomed Mater Res A. 2012;100(9):2499–2506.
- Yang Y, Shi H, Wang Y, et al. Graphene oxide/manganese ferrite nanohybrids for magnetic resonance imaging, photothermal therapy and drug delivery. J Biomater Appl. 2016;30(6):810–822.
- Wate PS, Banerjee SS, Jalota-Badhwar A, et al. Cellular imaging using biocompatible dendrimer-functionalized graphene oxide-based fluorescent probe anchored with magnetic nanoparticles. Nanotechnology. 2012;23(41):415101.
- Yang K, Zhang S, Zhang G, et al. Graphene in mice: ultrahigh in vivo tumor uptake and efficient photothermal therapy. Nano Lett. 2010;10(9):3318–3323.
- Depan D, Girase B, Shah JS, et al. Structure-process-property relationship of the polar graphene oxide-mediated cellular response and stimulated growth of osteoblasts on hybrid chitosan network structure nanocomposite scaffolds. Acta Biomater. 2011;7(9):3432–3445.
- Lu B, Li T, Zhao H, et al. Graphene-based composite materials beneficial to wound healing. Nanoscale. 2012;4(9):2978–2982.
- Chen G-Y, Pang DW-P, Hwang S-M, et al. A graphene-based platform for induced pluripotent stem cells culture and differentiation. Biomaterials. 2012;33(2):418–427.
- Liu H, Zhang L, Yan M, et al. Carbon nanostructures in biology and medicine. J Mater Chem B. 2017;5(32):6437–6450.
- Wang Y, Lee WC, Manga KK, et al. Fluorinated graphene for promoting neuro-induction of stem cells. Adv Mater Weinheim. 2012;24(31):4285–4290.
- Citrin D, Lee AK, Scott T, et al. In vivo tumor imaging in mice with near-infrared labeled endostatin. Mol Cancer Ther. 2004;3(4):481–488.
- Yun JM, Kim KN, Kim JY, et al. DNA origami nanopatterning on chemically modified graphene. Angew Chem Int Ed Engl. 2012;51(4):912–915.
- Cohen-Karni T, Langer R, Kohane DS. The smartest materials: the future of nanoelectronics in medicine. ACS Nano. 2012;6(8):6541–6545.
- Kasry A, Afzali AA, Oida S, et al. Detection of biomolecules via benign surface modification of graphene. Chem Mater. 2011;23(22):4879–4881.
- Li C, Adamcik J, Mezzenga R. Biodegradable nanocomposites of amyloid fibrils and graphene with shape-memory and enzyme-sensing properties. Nat Nanotechnol. 2012;7(7):421–427.
- Lian M, Fan J, Shi Z, et al. Gelatin-assisted fabrication of graphene-based nacre with high strength, toughness, and electrical conductivity. Carbon. 2015;89:279–289.
- Ge Y, Wang J, Shi Z, et al. Gelatin-assisted fabrication of water-dispersible graphene and its inorganic analogues. J Mater Chem. 2012;22(34):17619–17624.
- Wan C, Frydrych M, Chen B. Strong and bioactive gelatin–graphene oxide nanocomposites. Soft Matter. 2011;7(13):6159–6166.
- Jang S-C, Kang S-M, Lee JY, et al. Nano-graphene oxide composite for in vivo imaging. Int J Nanomedicine. 2018;13:221–234.
- Kim H, Namgung R, Singha K, et al. Graphene oxide-polyethylenimine nanoconstruct as a gene delivery vector and bioimaging tool. Bioconjug Chem. 2011;22(12):2558–2567.
- Silva M, Alves NM, Paiva MC. Graphene‐polymer nanocomposites for biomedical applications. Polym Adv Technol. 2018;29(2):687–700.
- McCallion C, Burthem J, Rees-Unwin K, et al. Graphene in therapeutics delivery: problems, solutions and future opportunities. Eur J Pharm Biopharm. 2016;104:235–250.
- Zare-Zardini H, Taheri-Kafrani A, Amiri A, et al. New generation of drug delivery systems based on ginsenoside Rh2-, Lysine- and Arginine-treated highly porous graphene for improving anticancer activity. Sci Rep. 2018;8(1):586.
- Pattnaik S, Swain K, Lin Z. Graphene and graphene-based nanocomposites: biomedical applications and biosafety. J Mater Chem B. 2016;4(48):7813–7831.
- Wang M, Wu J, Li Y, et al. A tumor targeted near-infrared light-controlled nanocomposite to combat with multidrug resistance of cancer. J Control Release. 2018;288:34–44.
- Sun X, Liu Z, Welsher K, et al. Nano-graphene oxide for cellular imaging and drug delivery. Nano Res. 2008;1(3):203–212.
- Kim H, Lee D, Kim J, et al. Photothermally triggered cytosolic drug delivery via endosome disruption using a functionalized reduced graphene oxide. ACS Nano. 2013;7(8):6735–6746.
- Liu Z, Robinson JT, Sun X, et al. PEGylated nanographene oxide for delivery of water-insoluble cancer drugs. J Am Chem Soc. 2008;130(33):10876–10877.
- Dembereldorj U, Kim M, Kim S, et al. A spatiotemporal anticancer drug release platform of PEGylated graphene oxide triggered by glutathione in vitro and in vivo. J Mater Chem. 2012;22(45):23845–23851.
- Depan D, Shah J, Misra R. Controlled release of drug from folate-decorated and graphene mediated drug delivery system: synthesis, loading efficiency, and drug release response. Mater Sci Eng C. 2011;31(7):1305–1312.
- Wen H, Dong C, Dong H, et al. Engineered redox-responsive PEG detachment mechanism in PEGylated nano-graphene oxide for intracellular drug delivery. Small. 2012;8(5):760–769.
- Yang Y, Zhang Y-M, Chen Y, et al. Construction of a graphene oxide based noncovalent multiple nanosupramolecular assembly as a scaffold for drug delivery. Chemistry. 2012;18(14):4208–4215.
- Liu K, Zhang J-J, Cheng F-F, et al. Green and facile synthesis of highly biocompatible graphene nanosheets and its application for cellular imaging and drug delivery. J Mater Chem. 2011;21(32):12034–12040.
- Wang H, Wang X, Xie C, et al. Nanodisk-based glioma-targeted drug delivery enabled by a stable glycopeptide. J Control Release. 2018;284:26–38.
- Wang H, Gu W, Xiao N, et al. Chlorotoxin-conjugated graphene oxide for targeted delivery of an anticancer drug. Int J Nanomedicine. 2014;9:1433–1442.
- Wang C, Zhang Z, Chen B, et al. Design and evaluation of galactosylated chitosan/graphene oxide nanoparticles as a drug delivery system. J Colloid Interface Sci. 2018;516:332–341.
- Liu X, Cheng X, Wang F, et al. Targeted delivery of SNX-2112 by polysaccharide-modified graphene oxide nanocomposites for treatment of lung cancer. Carbohydr Polym. 2018;185:85–95.
- Yang X, Zhang X, Ma Y, et al. Superparamagnetic graphene oxide–Fe3O4 nanoparticles hybrid for controlled targeted drug carriers. J Mater Chem. 2009;19(18):2710–2714.
- Iannazzo D, Pistone A, Salamò M, et al. Graphene quantum dots for cancer targeted drug delivery. Int J Pharm. 2017;518(1–2):185–192.
- Some S, Gwon A-R, Hwang E, et al. Cancer therapy using ultrahigh hydrophobic drug-loaded graphene derivatives. Sci Rep. 2014;4:6314.
- Sherlock SP, Tabakman SM, Xie L, et al. Photothermally enhanced drug delivery by ultrasmall multifunctional FeCo/graphitic shell nanocrystals. Acs Nano. 2011;5(2):1505–1512.
- Zhang L, Xia J, Zhao Q, et al. Functional graphene oxide as a nanocarrier for controlled loading and targeted delivery of mixed anticancer drugs. Small. 2010;6(4):537–544.
- Pan Y, Bao H, Sahoo NG, et al. Water‐soluble poly (N‐isopropylacrylamide)–graphene sheets synthesized via click chemistry for drug delivery. Adv Funct Mater. 2011;21(14):2754–2763.
- Zhao D, Yu S, Sun B, et al. Biomedical applications of chitosan and its derivative nanoparticles. Polymers. 2018;10(4):462.
- Wu J, Wang Y-s, Yang X-y, et al. Graphene oxide used as a carrier for adriamycin can reverse drug resistance in breast cancer cells. Nanotechnology. 2012;23(35):355101.
- Fan X, Jiao G, Gao L, et al. The preparation and drug delivery of a graphene-carbon nanotube-Fe3O4 nanoparticle hybrid. J Mater Chem B. 2013;1(20):2658–2664.
- Tian B, Wang C, Zhang S, et al. Photothermally enhanced photodynamic therapy delivered by nano-graphene oxide. ACS Nano. 2011;5(9):7000–7009.
- Kim H, Kim WJ. Photothermally controlled gene delivery by reduced graphene oxide-polyethylenimine nanocomposite. Small. 2014;10(1):117–126.
- Feng L, Zhang S, Liu Z. Graphene based gene transfection. Nanoscale. 2011;3(3):1252–1257.
- Shen H, Liu M, He H, et al. PEGylated graphene oxide-mediated protein delivery for cell function regulation. ACS Appl Mater Interfaces. 2012;4(11):6317–6323.
- La W-G, Park S, Yoon H-H, et al. Delivery of a therapeutic protein for bone regeneration from a substrate coated with graphene oxide. Small. 2013;9(23):4051–4060.
- Cao L, Zhang F, Wang Q, et al. Fabrication of chitosan/graphene oxide polymer nanofiber and its biocompatibility for cartilage tissue engineering. Mater Sci Eng C Mater Biol Appl. 2017;79:697–701.
- Mohammadi S, Shafiei SS, Asadi-Eydivand M, et al. Graphene oxide-enriched poly (ε-caprolactone) electrospun nanocomposite scaffold for bone tissue engineering applications. J Bioactive Compat Polym. 2017;32(3):325–342.
- Shadjou N, Hasanzadeh M, Khalilzadeh B. Graphene based scaffolds on bone tissue engineering. Bioengineered. 2018;9(1):38–47.
- Ryoo S-R, Kim Y-K, Kim M-H, et al. Behaviors of NIH-3T3 fibroblasts on graphene/carbon nanotubes: proliferation, focal adhesion, and gene transfection studies. ACS Nano. 2010;4(11):6587–6598.
- Wang K, Ruan J, Song H, et al. Biocompatibility of graphene oxide. Nanoscale Res Lett. 2011;6(1):8.
- Nair M, Nancy D, Krishnan AG, et al. Graphene oxide nanoflakes incorporated gelatin-hydroxyapatite scaffolds enhance osteogenic differentiation of human mesenchymal stem cells. Nanotechnology. 2015;26(16):161001.
- Ren N, Li J, Qiu J, et al. Growth and accelerated differentiation of mesenchymal stem cells on graphene-oxide-coated titanate with dexamethasone on surface of titanium implants. Dent Mater. 2017;33(5):525–535.
- Jung HS, Choi Y-j, Jeong J, et al. Nanoscale graphene coating on commercially pure titanium for accelerated bone regeneration. RSC Adv. 2016;6(32):26719–26724.
- Morales‐Narváez E, et al. Graphene‐based biosensors: going simple. Adv Mater. 2017;29(7):1604905.
- Chauhan N, Maekawa T, Kumar DNS. Graphene based biosensors—accelerating medical diagnostics to new-dimensions. J Mater Res. 2017;32(15):2860–2882.
- Janegitz BC, Silva TA, Wong A, et al. The application of graphene for in vitro and in vivo electrochemical biosensing. Biosens Bioelectron. 2017;89(Pt 1):224–233.
- Peña-Bahamonde J, Nguyen HN, Fanourakis SK, et al. Recent advances in graphene-based biosensor technology with applications in life sciences. J Nanobiotechnology. 2018;16(1):75.
- Tamanaha CR. A survey of graphene-based field effect transistors for bio-sensing. In: Kranz C, editor. Carbon-based nanosensor technology. Cham: Springer; 2017. p. 165–200.
- Vicarelli L, Vitiello MS, Coquillat D, et al. Graphene field-effect transistors as room-temperature terahertz detectors. Nat Mater. 2012;11(10):865–871.
- Suvarnaphaet P, Pechprasarn S. Graphene-based materials for biosensors: a review. Sensors. 2017;17(10):2161.
- Yang Z, Hao X, Chen S, et al. Long-term antibacterial stable reduced graphene oxide nanocomposites loaded with cuprous oxide nanoparticles. J Colloid Interface Sci. 2019;533:13–23.
- Li J, Wang G, Zhu H, et al. Antibacterial activity of large-area monolayer graphene film manipulated by charge transfer. Sci Rep. 2014;4:4359.
- Yang S, Lei P, Shan Y, et al. Preparation and characterization of antibacterial electrospun chitosan/poly (vinyl alcohol)/graphene oxide composite nanofibrous membrane. Appl Surf Sci. 2018;435:832–840.
- Shuai C, Guo W, Wu P, et al. A graphene oxide-Ag co-dispersing nanosystem: dual synergistic effects on antibacterial activities and mechanical properties of polymer scaffolds. Chem Eng J. 2018;347:322–333.
- Shao W, Liu X, Min H, et al. Preparation, characterization, and antibacterial activity of silver nanoparticle-decorated graphene oxide nanocomposite. ACS Appl Mater Interfaces. 2015;7(12):6966–6973.
- Liu T, Liu Y, Liu M, et al. Synthesis of graphene oxide-quaternary ammonium nanocomposite with synergistic antibacterial activity to promote infected wound healing. Burns Trauma. 2018;6(1):16.
- Krishnamoorthy K, Umasuthan N, Mohan R, et al. Antibacterial activity of graphene oxide nanosheets. Sci Adv Mat. 2012;4(11):1111–1117.
- Valentini F, Calcaterra A, Ruggiero V, et al. Functionalized graphene derivatives: antibacterial properties and cytotoxicity. J Nanomater. 2019;2019:1–14.