Abstract
Purpose
To explore the feasibility of constructing tissue-engineered vascularised oral mucosa-like structures with rabbit ACVM-0.25% HLC-I scaffold and human gingival fibroblasts (HGFs), human gingival epithelial cells (HGECs) and vascular endothelial-like cells (VEC-like cells).
Method
Haematoxylin and Eosin (H&E) staining, immunohistochemical, immunofluorescence, 5-ethynyl-2′-deoxyuridine (EdU) staining and scanning electron microscope (SEM) were performed to detect the growth status of cells on the scaffold complex. After the scaffold complex implanted into nude mice for 28 days, tissues were harvested to observe the cell viability and morphology by the same method as above. Additionally, biomechanical experiments were used to assess the stability of composite scaffold.
Results
Immunofluorescence and Immunohistochemistry showed positive expression of Vimentin, S100A4 and CK, and the induced VEC-like cells had the ability to form tubule-like structures. In vitro observation results showed that HGFs, HGECs and VEC-like had good compatibility with ACVM-0.25% HLC-I and could be layered and grow in the scaffold. After implanted, the mice had no immune rejection and no obvious scar repair on the body surface. The biomfechanical test results showed that the composite scaffold has strong stability.
Conclusion
The tissue-engineered vascularised complexes constructed by HGFs, HGECs, VEC-like cells and ACVM-0.25% HLC-I has good biocompatibility and considerable strength.
Introduction
Oral mucosa is the first barrier against various harmful substances from the outside and plays a very important role in protecting submucosal tissues [Citation1]. Tumour resection, mucosal lesions, trauma and other factors can cause large-scale oral mucosal defects. If a large-scale mucosal defect cannot be repaired in time, it will lead to granulation tissue contraction and scar tissue contraction, which will eventually affect the reconstruction of chewing, swallowing, mouth opening and other functions, and will greatly affect the patient's life [Citation1,Citation2]. Therefore, the study of restoring functional oral mucosa is a hot spot in clinical research. At present, the main repair methods are autologous free flap and myofascial membrane transplantation [Citation3,Citation4], but this repair method is at the expense of normal tissues, which will cause secondary trauma and bring great pain to patients. Recent studies have shown that tissue-engineered oral mucosa (TEOM) [Citation2,Citation5] is an ideal reconstruction material, which opens up a new approach to repair oral mucosal soft tissue defects.
The ideal oral mucosa is divided into epithelial layer, lamina propria, basement membrane and submucosa. The epithelial layer is mainly composed of epithelial cells; the lamina propria is mainly fibroblasts; the basement membrane is the connective tissue junction between the epithelial layer and the lamina propria [Citation6]. Studies have shown that fibroblasts and epithelial cells are the main conditions for the formation of oral mucosa to replace the in vitro [Citation7,Citation8], that is, the construction of oral mucosal tissues in vitro requires the patient's autologous epithelial cells to construct tissue-engineered oral mucosal epithelial cells, and autologous fibroblasts to construct the lamina propria of tissue engineering oral mucosa. However, it was found that the regeneration of normal tissues must be based on the reconstruction of capillary network, the regeneration of tissues also grows with the growth of blood vessels, that is, tissues need vascular nutrition to survive [Citation9]. Thus it can be seen that if the capillary network can grow into the tissue-engineered oral mucosa lamina propria, it will play an irreplaceable role in the growth and survival of the constructed tissue engineered oral mucosa [Citation10]. So, VEC become the core of constructing tissue-engineered vascularisation of oral mucosa in addition to fibroblasts and epithelial cells [Citation11].
In this study, human gingival fibroblasts (HGFs), human gingival epithelial cells (HGECs) and vascular endothelial-like cells (VEC-like cells) [Citation12] were used as seed cells in combination with ACVM-0.25% HLC-I [Citation13–16] to construct tissue-engineered vascularised oral mucosa-like structures in vitro and in vivo, and attempted to establish deep blood vessel connections through vascularisation, aiming at repairing tissue damage.
Material and method
Gingival tissue acquisition
Normal gingival tissue was collected from 10 patients with impacted mandibular wisdom tooth who underwent extraction surgery at Affiliated Stomatology Hospital of Southwest Medical University. Written informed consent was obtained from each patient. This study was approved by the ethics committee of the Southwest Medical University.
In the following study, HGFs, HGECs were isolated and cultured from gingival tissue. In addition, some HGFs are induced to VEC-like cells.
Laboratory animals
16 female New Zealand white rabbits weighting 1.8–2.0 kg (12 weeks old) were provided by the Animal Centre of Hebei Medical University. Four- Five week-old female BALB/C nude mice (weight 15 g) were purchased from the Experimental Animals of Chengdu dashuo (license number: SYXK 2018-065).
Cell isolation and culture of HGFs and HGECs
The gingival tissue was isolated, and gingival mucosa epithelium and connective tissue were eliminated by ophthalmic scissor. The gingival tissue was cut into 1 mm ×1 mm and then transferred into a culture flask with high-glucose DMEM containing with 18% foetal bovine serum (FBS; Gibco Corporation), and cultivated in an incubator at 37 °C, 5% CO2, and 95% relative humidity. The medium was changed every other day. The fibroblasts grew to achieve 80% confluence, and were harvested by trypsin (Sigma Biotechnology) digestion at 37 °C for 5 min, then continued to cultivate. The 3rd generation of HGFs and HGECs were used to experiments.
Induction of vascular endothelial cells and tubular formation assay
Preliminary experiments of our research group [Citation12] showed that the endothelium-like changes in HGFs were most obvious when VEGF165 concentration was 8 ng/mL and induction time was 35 days. On this basis, VEGF165 was directly administered at a concentration of 8 ng/mL for induction for 35 days.
Matrigel was loaded into each well of 24-well plate and allowed to polymerise for 1 h at 37 °C. HGFs (5 × 105 cells/mL) were added onto Matrigel and cultured in 5% Foetal Bovine Serum Endothelial Cell Medium containing VEGF165 only in 5% CO2 at 37 °C. After 24 h incubation, cell growth and organisation were observed through an OLYMPUS IX71 inverted microscope, and tubular-like structures were quantified by counting and averaging tubular length from five high-power fields.
Preparation of HGECs-HGFs-VECs-ACVM-0.25% HLC-I composite scaffold
An ACVM-0.25% HLC-I scaffold was prepared on the basis of previous studies [Citation14,Citation15]. The third-generation of HGFs with good growth condition was collected, digested at 0.25% trypsin (Sigma Biotechnology) 37 °C for 5 min, and then centrifuged at 1000 rpm/min to remove supernatant and adjust cell concentration to 1 × 106 cells/mL. HGFs were uniformly planted on the ACVM-0.25% HLC-I scaffold to construct the seed cell scaffold complex I.
VEC-like cells with good growth condition were collected, digested at 0.25% trypsin (Sigma Biotechnology) 37 °C for 5 min, and then centrifuged at 1000 rpm/min to remove supernatant and adjust cell concentration to 1 × 106 cells/mL. VEC-like cells were uniformly planted on the ACVM-0.25% HLC-I scaffold to construct the seed cell scaffold complex II.
Liquid Matrigel was added evenly to the seed cell scaffold complex I and allowed to polymerise for 30 min at 37 °C. Then the seed cell scaffold complex II was implanted on the seed cell scaffold complex I to construct the seed cell scaffold complex III.
Liquid Matrigel was added evenly to the seed cell scaffold complex III and allowed to polymerise for 30 min at 37 °C. Then HGECs, which was in good growth condition with the adjusted cell concentration of 1 × 106 cells/mL, were uniformly implanted on the seed cell scaffold complex III to construct the seed cell scaffold complex IV.
Immunofluorescence analysis
HGFs and HGECs were soaked in acetone and rinsed in PBS. These cells were then incubated in a humidified chamber with primary antibodies against Vimentin, S100A4, and CK, respectively. On the following day, the cells were washed with PBS followed by FITC/Rhodamine-conjugated secondary antibody. Following rinsing in PBS, they were mounted with a fluorescent mounting medium containing DAPI stain. Co-localization was observed by a blinded observer in a fluorescence microscope (Olympus, Tokyo, Japan).
For the identification of the seed cell scaffold complex IV, the composite scaffolds were incubated in a humidified chamber with primary antibodies directed against Vimentin, Tie-2 and CK.
Immunohistochemical analysis
Briefly, endogenous peroxidase activity within the sections was quenched by incubating the sections with 3% H2O2 for 10 min after dewaxing and hydration. HGECs were incubated in a humidified chamber with primary antibodies directed against CK followed by incubated with secondary antibody. In the negative controls, the primary antibody was replaced with CD34. Staining of the cells was performed by adding fresh diaminobenzidine (DAB) solution onto each slide. Counter staining was performed using haematoxylin for 10 min, and then the slides were rinsed with distilled water.
Haematoxylin and eosin staining
HGECs-HGFs-VECs-ACVM-0.25% HLC-I complex were deparaffinized in xylene and rehydrated in a series of alcohol solutions. After a brief wash in distilled water, the complex were stained with Harris haematoxylin solution for 5 min, washed in tap water and counterstained in eosin-phloxine solution for 2 min.
Scanning electron microscopy observation
HGECs-HGFs-VECs-ACVM-0.25% HLC-I complex was fixed in a metal and coated with a thin layer of gold, using an Edwards EXC 120 Turbo Pump Controller. A SEM (JSM-5310; JEOL, Ltd., Tokyo, Japan) was used to investigate the surface morphology of the four scaffolds.
EdU staining analysis
The 3rd generation of HGECs and VEC-like cells were incubated in DMEM medium containing 10 mM EdU for 24 h. After incubation, the HGECs, VEC-like cells and HGFs were fixed with 4% paraformaldehyde for 30 min and then neutralised with 2 mg/mL glycine for 5 min. Cells were washed three times with PBST for 10 min. Apollo reaction solution 488 was used to stain the HGECs, Apollo reaction solution 567 was used to stain the VEC-like cells and DAPI was used to stain the HGFs. A portion of the labelled cells were used for in vitro tracing and another portion was used for subsequent studies with ACVM-0.25% HLC-I.
Implantation
According to different experimental conditions, 18 female BALB/C nude mice aged 4–5 weeks were divided into three groups: (1) The experimental group; (2) Empty scaffold group; (3) Control group. First, the mice were anaesthetised with 10% chloral hydrate. Then, 10 mm × 10.0 mm incision was made on the dorsa, and one subcutaneous pocket was created on the right side of the incision using blunt dissection. The labelled HGFs-VECs-ACVM-0.25% HLC-I (EdU label) was implanted into the pocket. After placement of the implants, the incisions were covered with a polyurethane film and then sutured in place. In empty scaffold group, ACVM-0.25% HLC-I was implanted into the incision, while the control group was not treated. After 14 days, the polyurethane film was removed and the bioglue was applied to the wound surface. In the experimental group, HGECs (EdU label) were planted on the surface of the bioglue, and the control group still did not do any treatment.
Sample tissue acquisition
After 28 days of implantation, the mice were euthanized. Then, tissue resection was performed at the implantation site of the experimental group and the empty scaffold group, and the corresponding skin tissue was cut out in the control group. A portion of the cut tissue was fixed with 10% formaldehyde, and the other portion was stored at −80 °C. Formaldehyde-fixed tissue were used for HE staining, EdU trace staining, and Immunofluorescence staining, while fresh tissue were used in mechanical experiments. The steps of HE staining, EdU trace staining and immunofluorescence staining were the same as above.
Mechanical property of the scaffold
A Zwick/Roell Z020 mechanical testing machine (purchased from Zwick&Roell company, Germany) was utilised to determine the tensile strength, breaking strength, and elongation at break. The two ends of PBS wetted samples with a length of 1 cm were fixed to the mechanical testing machine. Stretch tests were performed at a speed of 10 mm/min until the sample ruptured. Stress and strain indices were recorded during this process. Stress-strain curves were obtained by plotting stress on the ordinate and strain on the abscissa, and stress-time curves were obtain by plotting stress on the ordinate and time on the abscissa.
Statistical analysis
SPSS 21.0 (IBM, New York, USA) was applied to analyse all data. After normality test and homogeneity test of variance, the data are consistent with normal variance and expressed as mean ± standard deviation. One-way ANOVA was used for statistical analysis, and SNK-q test was used for pairwise comparison between groups. Values of p < .05 were considered statistically significant.
Results
Cytoskeletal morphology of HGFs and HGECs
The first adherent HGFs cells appeared 7 d after initiation of the primary culture. At this time, fbroblast-like cells had a clear morphology, a clear outline, and the nucleus showed an elliptical shape (). When the primary cells reached 80% confluence, cell subculture was then conducted. After 15 days, the cells gradually tend to have consistent morphology in fusiform shape appearing fibroblast-like phenotype. The cells were tightly adhered and well-spread ().
Figure 1. Culture of HGFs and HGECs in vitro. (A) Primary culture of HGFs, the cells had a clear morphology, and the nucleus showed an elliptical shape; Scale bar = 500 µm. (B) Subculture of HGFs, the cells gradually tend to have consistent morphology in fusiform shape appearing fibroblast-like phenotype; Scale bar = 200 µm. (C) Primary culture of HGECs, the cells grew radially around the tissue block, and showed an oblate shape appearing fibroblast-like phenotype; Scale bar = 500 µm. (D) Subculture HGECs, the cells appeared in epithelioid shape, and presented a “paving stone” like cluster aggregation; Scale bar = 200 µm.
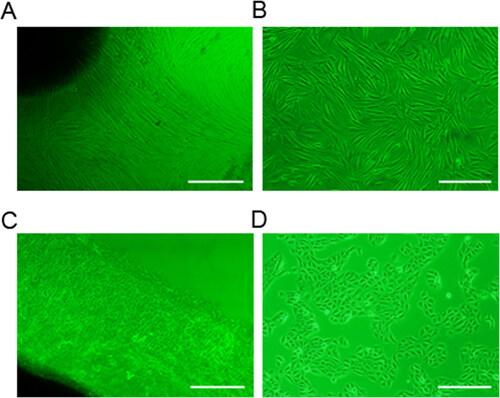
On the sixth day of primary culture, HGECs began to grow radially around the tissue mass. The cells were oblate, with a fibroblast-like phenotype, full cytoplasm and no protrusions. The nuclei were round or oval shape, with one to several (). After cell subculture, a large number of epithelioid shaped cells were obtained, and the cells presented a “paving stone” like cluster aggregation ().
Characterisation of HGFs
The characteristics of HGFs were identifed by Immunofluorescent staining of Vimentin and S100A4, markers for mesenchymal cells. It was observed that the protein expression of Vimentin and S100A4 was positive in the cytoplasm of HGFs ().
Characterisation of HGECs
Immunocytochemistry was used to detect the CK expression in the HGECs cells, while CD34 served as a negative control. Immunocytochemistry results showed that CK was positively expressed in the cytoplasm of HGECs, while CD34 was negatively expressed in the cytoplasm of cells (). To further identify the HGECs cells, we conducted immunofluorescence staining. The staining results were consistent with the above results, that is, CK protein was positively expressed in the HGECs cytoplasm, showing red fluorescence ().
Figure 3. Characterisation of HGECs. (A) Immunocytochemistry results showed that CK was positively expressed in the cytoplasm of HGECs, while CD34 was negatively expressed in the cytoplasm of cells. Scale bar = 50 µm. (B) CK was positively displayed in the cytoplasm of HGECs, showing red fluorescence. Scale bar = 200 µm.
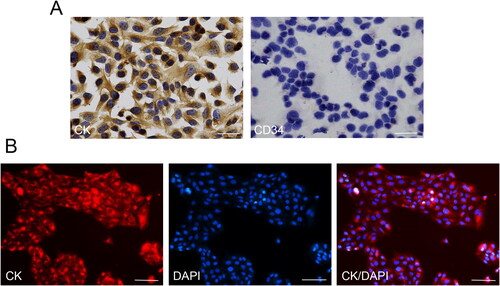
VEGF165 induced tube-forming ability test
Based on our previous studies, we used 8 ng/mL VEGF165 to directly induce HGFs for 35 days. To detect VEGF165-induced tube formation, we randomly selected five fields under an inverted microscope to observe the cell arrangement. Under the microscope, we found that VEC-like cells in VEGF165-induced group presented tubular structure, while no cells in the control group presented tubular structure, suggesting that HGFs cells had endothelial ductile function after induction of VEGF165 ().
Figure 4. Endothelial-like cells tubular formation assay. No tubular formation was observed when HGFs were cultured in Matrigel for 24 h; while tubular-like structures were observed when endothelial-like cells differentiated from HGFs were cultured in Matrigel for 24 h. Scale bar = 100 µm.
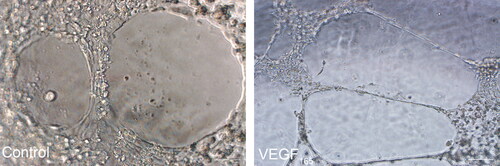
Observation of HGECs-HGFs-VECs-ACVM-0.25% HLC-I
To assess the implantation effect of HGECs, HGFs and VEC-like cells onto the ACVM-0.25% HLC-I scaffold, HE staining and immunofluorescence staining were performed to reveal the growth status of these cells. As shown in the HE staining of , the three seed cells grew well on the ACVM-0.25% HLC-I scaffold and these cells exhibited a process of expansion in different directions, indicating that they have potential migration and cell viability (). Results of immunofluorescence staining showed that Vimentin was positively expressed in HGFs, Tie-2 was positively expressed in VEC-like cells, and CK was positively expressed in HGECs ().
Figure 5. HE staining, Immunofluorescence staining and SEM observation of HGFs, HGECs and VEC-like cell on the surface and inside the ACVM-0.25% HLC-I scaffold. (A) HE results revealed that HGFs, HGECs and VEC-like cell grew well on the ACVM-0.25% HLC-I scaffold. (B) Vimentin, CK and Tie-2 were positively expressed in HGFs, HGECs and VEC-like cell, respectively. Scale bar = 100 µm. (C) When the scan magnification was 500, SEM images showed that oblate cells were clearly seen on the composite scaffold surface, while at 1200 magnification, the three types of cells were layered on the scaffold. The yellow arrow indicated HGECs, the blue arrow indicates VEC-like cells, and the green arrow indicated HGFs.
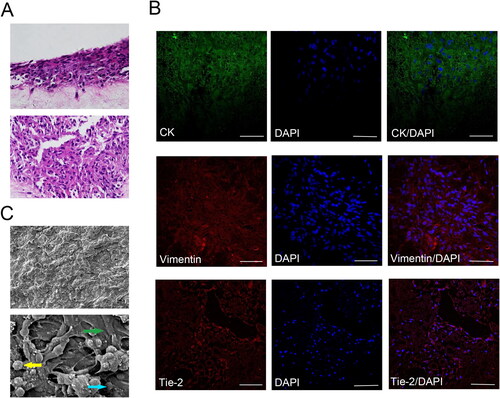
SEM images of the surfaces of the HGECs-HGFs-VECs-ACVM-0.25%HLC-I scaffolds were presented in . When the scan magnification was 500, the oblate cells on the scaffold were clearly visible. Interestingly, when the scan magnification was 1200, SEM observation revealed that the HGECs-HGFs-VECs-ACVM-0.25% HLC-I composite scaffold could be divided into three layers. The outermost layer was HGECs cells, the middle layer was
VEC-like cells, and the innermost layer was HGFs cells ().
In vitro tracing observation of HGECs, HGFs and VEC-like cells
More than 90% of induced VEC-like cells were marked by EdU Apollo 567, showing red fluorescence in both the cytoplasm and nucleus, while HGECs cells were marked by EdU Apollo 488, showing green fluorescence in both the cytoplasm and nucleus. Nearly all were HGFs cells stained by DAPI, showing blue fluorescence in the nucleus ().
Figure 6. Tracing observation of seeded cells in vitro. VEC-like cells were stained with EdU Apollo 567, showing red fluorescence in both the cytoplasm and nucleus; HGECs were stained by EdU Apollo 488, showing green fluorescence in both the cytoplasm and nucleus; HGFs were stained with DAPI, showing blue fluorescence in the nucleus. Scale bar = 100 µm.
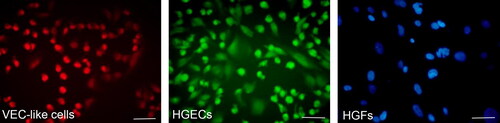
Observation of the state of nude mice after implantation of scaffolds complex
Postoperatively, we observed the growth of three groups of nude mice. Within 2 days after the surgery, all three groups of mice awakened. However, 2 nude mice in the empty scaffold group died, and no death was observed in the experimental group and control group. Subsequently, the status of each group of nude mice was observed daily, and no redness and ulceration occurred in the wounds of nude mice. On the 14th day after the surgery, polyurethane film was removed and bioglue was applied on the wound surface. In the third week, the wounds in the experimental group were completely repaired, with no obvious scar, suture discharge and no abnormal phenomenon, while no epithelial repair was observed in the empty scaffold group. No abnormality in the normal group.
Histological observation of scaffold complex in vivo
To assess the viability of scaffold complex implanted into nude mice, HE staining, Edu staining and immunofluorescence staining were conducted. The results of HE staining in the experimental group showed that epithelioid-like structure was formed, with clear cell layers and no skin attachments. The epithelioid-like structure was thinner than the normal epithelial layer of nude mice, with a large number of cells on the tissue. In the empty scaffold group, no epithelioid-like structure was formed and the number of cells on the tissue was small. In the control group, the upper cortex was thicker, the cell layers were clear, and the skin attachments were abundant ().
Figure 7. Histological observation of scaffold complex in vivo. (A) HE staining results displayed that epithelioid-like structure was formed in the experimental group and was thinner than the normal epithelial layer; No epithelioid-like structure was formed in the empty scaffold group and the number of cells on the tissue was small. (B) In vivo tracer experiments, EdU567 was used for VEC-like cells, EdU488 for HGECs and DAPI for HGFs. Under the microscope, the epithelioid-like structure was expressed in green fluorescence (×40), and the vascular cavity-like structure was expressed in red fluorescence. The DAPI-labelled HGFs were distributed between the epithelioid-like structure and the vascular-like structure (×200). Scale bar = 100µm.
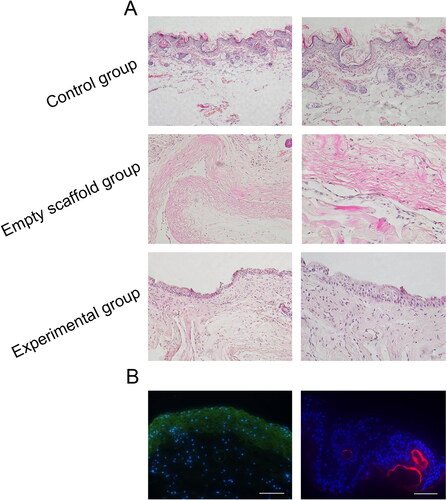
Further, in vivo tracer staining in nude mice indicated that the epithelioid-like structures in the constructed tissues showed green fluorescence expression, and the vascular cavity-like structure showed red fluorescence expression. The DAPI-labelled HGFs were distributed between the epithelioid-like structures and the vascular cavity-like structures (). A similar expression pattern was observed in immunofluorescence. In different fields of microscope, lamina propria-like structures, epithelioid-like structures and the vascular cavity-like structures were observed ().
Figure 8. Immunofluorescence staining in vivo. HGFs are positive for cytoplasmic Vimentin, Rhodamine stains its cytoplasm with red, FITC stains its cytoplasm with green, and DAPI stains all nuclei with blue. Under the microscope, epithelial-like cells were abundant in the epithelial-like area, DAPI staind the nucleus blue, and the epithelioid-like tissue migrates with the normal epithelium of nude mice. In addition, the vascular cavity-like structures can be seen under the field of view of VEC-like cell.
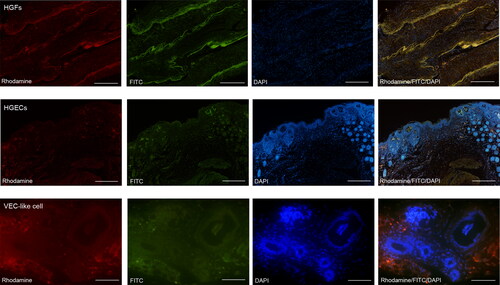
Mechanical test results of the scaffold complex
From the stress-time curve and the stress-strain curve, the tensile stress of the control group was the largest, reaching the peak value of 0.048 ± 0.003Mpa at 10.5 s; the experimental group reached the peak value of 0.045 ± 0.002Mpa at 9 s; the empty scaffold group was the smallest, at 8.5 s, the peak value was 0.040 ± 0.002Mpa (). Pairwise comparison of stress size between the three groups showed significant differences (p < .05, ).
Figure 9. The results of mechanical test of the scaffold complex. (A) Stress-time curve; (B) Stress-strain curve.
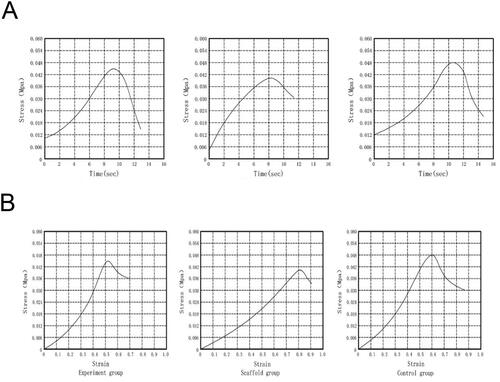
Table 1. Stress comparison of three groups of tissues.
Discussion
ACVM-0.25% -HLC-I scaffold is a tissue engineering composite scaffold material, which has the advantages of natural biological scaffold material and synthetic biodegradable polymer scaffold material. In other words, it provides three-dimensional structural space for cell growth and has certain biomechanical properties [Citation14,Citation16]. Our previous study [Citation16] has demonstrated that ACVM-0.25% HLC-I has good compatibility with cells, low cytotoxicity, can promote cell adhesion, cell proliferation and cell migration, and has sufficient mechanical properties to maintain the tensile strength of the material. For the oral mucosa-like structures to be constructed in this study, a good oral mucosa scaffold (ACVM-0.25% HLC-I) should be able to rapidly induce the regeneration of oral mucosa-like structures, so that the regenerated oral mucosa-like structures are similar to the morphology and function of normal oral mucosa structures to some extent.
In vitro studies, biocompatibility is the key to verifying whether the ACVM-0.25% HLC-I scaffold material is suitable for layering three seed cells and forming functional layers. Therefore, this experiment focuses on layered planting and layered construction. HGECs, HGFs, and VEC-like cells were implanted on the ACVM-0.25% HLC-I scaffold using the Matrigel method [Citation17,Citation18], the feasibility of layered construction of each functional layer was investigated. The results showed that the three seed cells could grow in layers on the ACVM-0.25% HLC-I scaffold, which further indicated that the ACVM-0.25% HLC-I scaffold had good biocompatibility. In vivo studies, the HGECs-HGFs-VECs-ACVM-0.25% HLC-I complex is ultimately required to be implanted into the organism to play the function of tissue engineering to construct tissues and organs, such as repairing the defect of oral mucosa to some extent. As expected, in vivo results indicated that HGECs-HGFs-VECs-ACVM-0.25% HLC-I could survive in animals, and the tissue engineered vascularised oral mucosa-like structure remains intact, which could guide tissue repair and regeneration to a certain extent.
In previous studies, researchers have used tissue-engineered oral mucosa (TEOM) as one of the methods of oral mucosal repair and reconstruction, that is, implanting HGFs and HGECs on the stent to construct the lamina propria and epithelial layer, respectively. Rouabhia et al. [Citation19] found that the constructed TEOM epithelial layer had clear differentiation layers, and the lamina propria had a large number of fibroblast aggregation. After 60 days of skin graft repair in nude mice, skin-like tissue structure could be formed on the TEOM surface without obvious scar contraction. Vinuela-prieto et al. [Citation5] also proved that TEOM could be a good oral mucosa substitute. Survival in vivo for TEOM mainly depends on angiogenesis and continuous oxygen supply. Tra et al. [Citation20] introduced hypertonic oxygen therapy after transplantation and found that keratinocytes growth factor (KGF), vascular endothelial growth factor (VEGF) and placental growth factor (PIGF) were significantly increased after 5 HBO cycles, among which VEGF can significantly promote angiogenesis and improve blood circulation. Based on previous studies, we considered whether it is possible to construct tissue-engineered oral mucosa-like structure, and establish a connection between the oral mucosa-like structure and deep tissue vessels through vascularisation, so as to better improve the survival rate of tissue-engineered oral mucosa-like structure and maintain the repair effect. This is also the focus of this experiment. HGFs have properties similar to stem cells and can induce trans-differentiation into VEC-like cells [Citation12]. Therefore, in this study, VEC-like cells were used as vascularised seed cells for tissue engineered vascularised oral mucosa. After adding 8 ng/ml VEGF165 for 35 days, the cell morphology changed significantly. The cells were arranged into a tubular structure with different lumen diameters, indicating that the cells had tube-forming ability similar to endothelial cells. This further validates the results of previous studies.
In the repair of oral mucosa, HGFs are involved in the repair of connective tissue and the formation of scar [Citation21], while the formation of connective tissue supports and protects the epithelial structure. It was found that in the early stage of tissue injury, HGFs proliferate in a large amount, and in a period of 4–5 days, a large amount of collagen fibres and extracellular matrix could be secreted, and these tissues and new capillaries form granulation tissues to initially fill the wound [Citation22]. During the repair of the injury, HGFs migrate to the damaged area, filling the damaged area and secreting the extracellular matrix. Studies have shown that secretion or addition of EGF, bFGF, TGF-β1 and TGF-β3 can significantly promote the migration and proliferation of HGFs [Citation21] and EGF has become more and more common as a therapeutic means to accelerate tissue repair of skin and oral mucosa wounds [Citation23,Citation24]. So, is there a corresponding inducing factor on the ACVM-0.25% HLC-I scaffold to promote the migration and proliferation of HGFs? ACVM is a cell-free scaffold containing a large number of matrix components, including a variety of growth factors. Therefore, in the future studies, we will further explore the promoting effect of growth factors on seed cells in ACVM-0.25% HLC-I scaffold and its mechanism. VEC-like cells can produce vascular-like structures under the action of vascular endothelial factors [Citation12]. As one of the major angiogenic growth factors, VEGF plays a pleiotropic role in tissue repair through neovascularization, regenerative epithelialization and regulation of extracellular matrix [Citation25]. In this study, we planted the VECs-ACVM-0.25% HLC-I annular complex on the HGFs-ACVM-0.25% HLC-I complex, and tried to achieve in vitro vascularisation first, laying a foundation for the subsequent realisation of vascularisation in vivo in nude mice. Only by establishing a good capillary network and realising a good blood supply can the tissues survive better.
The realisation of vascularisation in vivo depends on the bioreactor. Bioreactor is a new type of equipment that has appeared in the field of biological engineering in recent years, which was used in many fields due to their diverse functions [Citation26–28]. In this study, the skin of nude mice was used as a bioreactor to simulate human oral mucosa defect, and the HGECs-HGFs-VECs-ACVM-0.25% HLC-I complex was implanted in the skin defect of nude mice to induce the formation of new tissues, so as to explore the feasibility of constructing tissue-engineered vascularised oral mucosa after implantation of the tissue-engineered vascularised tissue complex in vitro. Enhancement experiments in vivo showed that no obvious scar repair was observed at the wound surface of the nude mice in the experimental group after 28 days, and the implanted tissue had a good connection with the surrounding normal skin. The presence of hard tissue of the scaffold could be sensed by pressure, but the scaffold could not be removed by pulling, indicating that the cellular scaffold complex had a good compatibility with the tissue of the nude mice. EDU tracer staining results indicated that the seed cells with epithelioid-like structure, lamina propria-like structure and vascular-like structure were derived from HGECs, HGFs and VEC-like cells cultured in vitro.
Scaffold materials used in tissue engineering should have certain biomechanical strength [Citation16], also known as mechanical strength, to ensure that they have certain tensile stress and shear stress when implanted in human or animal bodies. Therefore, the composite scaffold implanted in the damaged oral mucosa should also have certain tensile and shear stresses to withstand oral chewing activity and internal blood flow impact. Biomechanical experiments results showed that the tensile strength of HGECs-HGFs-VECs-ACVM-0.25% HLC-I complex implanted in nude mice is weaker than that of nude mice, but better than that of blank scaffold group. Although the mechanical properties of the experimental group were weaker than normal tissues, they were well connected to the tissues surrounding the defect. The formation of the epithelial structure fully demonstrates that it has basic defense functions, and its stable presence in the defect site indicates that the experimental group could resist certain tensile stress and shear stress.
Conclusion
The constructed tissue-engineered vascularized oral mucosa-like structure has good biocompatibility, and has certain compressive stress and shear stress, which can better promote the tissue wounds. In the follow-up study, we will further investigate the vascularisation effect and vasculogenesis mechanism of tissue-engineered oral mucosa-like structure.
Author contributions
Xuqian Liu conceived of the study, and participated in data analysis, interpretation of the results. Minhai Nie provided administrative support. He Chen, Yali Hou and Yaoqiang Liu contributed to samples collection. Minyue Zhou, Xiao Chen and Yanling Qiu contributed to data collection, analysis and interpretation of the results. All authors read and approved the final manuscript and consented to publish this manuscript.
Disclosure statement
No potential conflict of interest was reported by the author(s).
Data availability statement
The datasets used and/or analysed during the current study are available from the corresponding author on reasonable request.
Additional information
Funding
References
- Meng FH, Shao XL, Song Y, et al. Advances in tissue-engineered oral mucosa. Zhongguo Yi Xue Ke Xue Yuan Xue Bao. 2017;39(6):851–856.
- Kinikoglu B, Damour O, Hasirci V. Tissue engineering of oral mucosa: a shared concept with skin. J Artif Organs. 2015;18(1):8–19.
- Wada T, Nakatani K, Hiraishi Y, et al. Usefulness of myofascial flap without skin in contemporary oral and maxillofacial reconstruction. J Oral Maxillofac Surg. 2011;69(6):1815–1825.
- Kim D-Y, Alfadil LO, Ahn K-M, et al. Reconstruction of thin and pliable oral mucosa after wide excision of oral cancer using a trimmed anterolateral thigh free flap as an adipofascial flap. J Craniofac Surg. 2018;29(4):e394–e396.
- Vinuela-Prieto JM, et al. Sequential keratinocytic differentiation and maturation in a three-dimensional model of human artificial oral mucosa. J Periodontal Res. 2015;50(5):658–665.
- Winning TA, Townsend GC. Oral mucosal embryology and histology. Clin Dermatol. 2000;18(5):499–511.
- Sanchez-Quevedo MC, Alaminos M, Capitan LM, et al. Histological and histochemical evaluation of human oral mucosa constructs developed by tissue engineering. Histol Histopathol. 2007;22(6):631–640.
- Guzman-Uribe D, et al. Oral mucosa: an alternative epidermic cell source to develop autologous dermal-epidermal substitutes from diabetic subjects. J Appl Oral Sci. 2017;25(2):186–195.
- Phelps EA, Garcia AJ. Engineering more than a cell: vascularization strategies in tissue engineering. Curr Opin Biotechnol. 2010;21(5):704–709.
- Sun X, Altalhi W, Nunes SS. Vascularization strategies of engineered tissues and their application in cardiac regeneration. Adv Drug Deliv Rev. 2016;96:183–194.
- Głowińska-Olszewska B, Luczyński W, Bossowski A. Endothelial progenitor cells as a new marker of endothelial function with respect to risk of cardiovascular disorders. Postepy Hig Med Dosw. 2011;65:8–15.
- Liu X, Wang J, Dong F, et al. Human gingival fibroblasts induced and differentiated into vascular endothelial-like cells. Dev Growth Differ. 2016;58(9):702–713.
- Lalka SG, Oelker LM, Malone JM, et al. Acellular vascular matrix: a natural endothelial cell substrate. Ann Vasc Surg. 1989;3(2):108–117.
- Liu X, Wang J, Dong F, et al. Cytocompatibility and biologic characteristics of synthetic scaffold materials of rabbit acellular vascular matrix combining with human-like collagen I. J Biomater Appl. 2017;32(4):463–471.
- Liu X, Wang J, Dong F, et al. Study of composite vascular scaffold combining with differentiated VSMC- and VEC-like cells in vitro and in vivo. J Biomater Appl. 2017;32(2):219–229.
- Qiu YL, et al. Characterization of different biodegradable scaffolds in tissue engineering. Mol Med Rep. 2019;19(5):4043–4056.
- Kleinman HK, Martin GR. Matrigel: basement membrane matrix with biological activity. Semin Cancer Biol. 2005;15(5):378–386.
- Liu L-L, Zhang B-B, Gao Q-W, et al. Morphological observation of bone marrow mesenchymal stem cells under matrigel three dimensional culture conditions. Int J Morphol. 2019;37(1):54–58.,
- Rouabhia M, Allaire P. Gingival mucosa regeneration in athymic mice using in vitro engineered human oral mucosa. Biomaterials. 2010;31(22):5798–5804.
- Tra WMW, Spiegelberg L, Tuk B, et al. Hyperbaric oxygen treatment of tissue-engineered mucosa enhances secretion of angiogenic factors in vitro. Tissue Eng A. 2014;20(9–10):1523–1530.
- Nan L, et al. Mechanical force promotes the proliferation and extracellular matrix synthesis of human gingival fibroblasts cultured on 3D PLGA scaffolds via TGFbeta expression. Mol Med Rep. 2019;19(3):2107–2114.
- Wang L, Yang J, Ran B, et al. Small molecular TGF-β1-inhibitor-loaded electrospun fibrous scaffolds for preventing hypertrophic scars. ACS Appl Mater Interfaces. 2017;9(38):32545–32553.
- Ryu S-H, Moon SY, Yang Y-J, et al. Recombinant human epidermal growth factor accelerates the proliferation of irradiated human fibroblasts and keratinocytes in vitro and in vivo. J Radiat Res. 2009;50(6):545–552.
- Qi S, Yang C, Zhu M, et al. Effect of oral mucosal transplantation on the expression of EGF and VEGF-C during skin wound repair. Exp Ther Med. 2019;18(1):320–325.
- Keswani SG, Balaji S, Le LD, et al. Role of salivary vascular endothelial growth factor (VEGF) in palatal mucosal wound healing. Wound Repair Regen. 2013;21(4):554–562.
- Xu S, Gavin J, Jiang R, et al. Bioreactor productivity and media cost comparison for different intensified cell culture processes. Biotechnol Prog. 2017;33(4):867–878.
- Schuerlein S, et al. A versatile modular bioreactor platform for tissue engineering. Biotechnol J. 2017;12(2):1600326.
- Kajbafzadeh A-M, Sabetkish S, Sabetkish N, et al. In-vivo trachea regeneration: fabrication of a tissue-engineered trachea in nude mice using the body as a natural bioreactor. Surg Today. 2015;45(8):1040–1048.