Abstract
Volume resuscitation is an important early treatment for haemorrhagic shock. Haemoglobin-based oxygen carrier (HBOC) can expand the volume and provide oxygen for tissues. Vascular leakage is common complication in the process of haemorrhagic shock and resuscitation. The aim of this study was to observe the effects of HBOC (a bovine-derived, cross-linked tetramer haemoglobin oxygen-carrying solution, 0.5 g/L) on vascular leakage in rats after haemorrhagic shock. A haemorrhagic shock rat model and hypoxic vascular endothelial cells (VECs) were used. The role of intercellular junctions and endothelial glycocalyx in the protective effects of HBOC and the relationship with mitochondrial function were analysed. After haemorrhagic shock, the pulmonary vascular permeability to FITC-BSA, Evans Blue was increased, endothelial glycocalyx was destroyed and the expression of intercellular junction proteins was decreased. After haemorrhagic shock, a small volume of HBOC solution (6 ml/kg) protected pulmonary vascular permeability, increased structural thickness of endothelial glycocalyx, the levels of its components and increased expression levels of the intercellular junction proteins ZO-1, VE-cadherin and occludin. Moreover, HBOC significantly increased oxygen delivery and consumption in rats, improved VEC mitochondrial function and structure. In conclusion, HBOC mitigates endothelial leakage by protecting endothelial glycocalyx and intercellular junctions through improving mitochondrial function and tissue oxygen delivery.
Introduction
Haemorrhage is a significant cause of early death in trauma patients and is the leading cause of early death in both wartime and peacetime trauma [Citation1,Citation2]. Pulmonary vascular leakage is a common pathophysiological phenomenon after haemorrhagic shock and fluid resuscitation. Vascular leakage results in the leakage of macromolecules and proteins into tissue gaps and tissue edoema, which is an important cause of lung injury. Therefore, it is essential to choose an effective treatment for preventing pulmonary vascular leakage during fluid resuscitation [Citation3–5].
Fluid resuscitation is an important method to restore hemodynamics after haemorrhagic shock. Crystalloid and colloid fluids, which are commonly used for resuscitation [Citation6–8], have a capacity expansion effect, but they cannot effectively improve tissue hypoxia. Haemoglobin-based oxygen carriers (HBOCs) are a blood substitute for acellular haemoglobin. They have the characteristics of low infection risk, good dilatation effects and oxygen-carrying function [Citation9,Citation10]. Previous studies have shown that HBOCs are beneficial for animals with trauma and haemorrhagic shock and show a better prognosis [Citation11,Citation12]. However, whether HBOCs are beneficial to vascular permeability after haemorrhagic shock is not clear.
Many factors participate in the regulation of vascular permeability, with intercellular junctions being a key factor. Previous studies found that haemorrhagic shock and hypoxia could result in decreased expression of intercellular junction proteins, an increase in intercellular space and enhanced transmembrane transport of proteins and other macromolecules [Citation3]. Moreover, the endothelial glycocalyx plays an important role in maintaining the vascular barrier. The structure of the endothelial glycocalyx is destroyed and the levels of glycocalyx components are decreased after haemorrhagic shock and hypoxia, which contribute to haemorrhagic shock- or hypoxia-induced vascular permeabilization and vascular leakage [Citation13]. It remained to be elucidated whether the protective effects of HBOC on pulmonary vascular leakage are related to intercellular junctions and the endothelial glycocalyx.
In this study, a rat model of haemorrhagic shock and hypoxic vascular endothelial cells (VECs) was used. The protective effects of HBOC on pulmonary function and against pulmonary vascular leakage after haemorrhagic shock and the mechanisms by which HBOC protects against pulmonary vascular leakage were investigated, focussing on the role of the intercellular junction, the endothelial glycocalyx and the relationship with VEC mitochondrial structure and function.
Materials and methods
See the online supplement information for detailed methods.
Statistics analysis
All data are presented as the mean ± SD of the number of observations. The differences in data among groups were analysed by one-way ANOVA when normality (homogeneity of variance) assumptions were satisfied; otherwise, the equivalent non-parametric test was used in the SPSS version 19.0 package (IBM). p < .05 was considered to indicate a statistical difference.
Results
Protective effects of HBOC on pulmonary vascular permeability after haemorrhagic shock
To observe the protective effects of the HBOC on pulmonary vascular permeability after haemorrhagic shock, the FITC-BSA and Evans Blue permeabilities of pulmonary vessels were measured 2 h after infusion of HBOC or LR. The pulmonary vascular permeability increased significantly after haemorrhagic shock; the pulmonary vascular permeability to FITC-BSA and Evans Blue was increased by 90.99 and 208.97% compared with the sham operation group, respectively (P<0.01, P<0.01) (). LR infusion did not improve vascular permeability. HBOC resuscitation significantly ameliorated the pulmonary vascular leakage of FITC-BSA and Evans Blue, which was 37.69 and 36.54% lower than that in the LR group, respectively (P<0.01, P<0.01) ().
Figure 1. Effects of HBOC on pulmonary vascular permeability after haemorrhagic shock. (A, B) The effects of HBOC on the pulmonary vascular permeability were analysed by measuring the mean OD of FITC-BSA in vivo (bar = 75 μm) (n = 8 observations per group). (C, D) The effects of HBOC on the pulmonary vascular permeability were analysed by measuring the leakage of Evans Blue (n = 8 observations per group). (E, F) The effects of HBOC on the TER and the transmittance of FITC-BSA in monolayer VECs after hypoxia (n = 8 observations per group). Data are presented as mean ± SD, each group contains eight observations, *p < 0.05 and **p < 0.01 as compared with sham operation/normal group, #p < 0.05 and ##p < 0.01 as compared with shock/hypoxia group, @p < 0.05 and @@p < 0.01 as compared with LR group. HBOC: haemoglobin based oxygen carrier; FITC-BSA: fluorescein isothiocyanate-labeled Albumin Bovine V; LR: Lactated Ringer’s solution; Nor: normal group; Sham: sham operation group; TER: transendothelial electrical resistance; VEC: vascular endothelial cell.
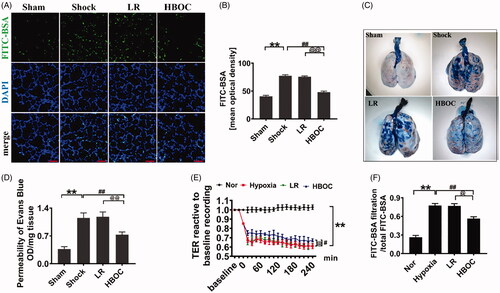
To further explore the protective effects of HBOC on vascular permeability, the changes in transendothelial electrical resistance (TER) and the transmittance of FITC-BSA after VEC incubation with HBOC (30 mg/ml) or LR for 2 h were measured. The barrier function of endothelial cells was destroyed after hypoxia; the transmittance of FITC-BSA in VECs was increased significantly compared with the control group (by 91.52%) (P<0.01), and the TER was decreased significantly (P<0.01) (). LR incubation did not improve TER or the transmittance of FITC-BSA of VECs. Incubation with HBOC (30 mg/ml) could ameliorate the hypoxia-induced increase of the transmittance of FITC-BSA, which was 16.09% lower than that in the LR group, and also antagonized the hypoxia-induced decrease in TER of VECs (P<0.01, P<0.01) (). These results indicate that HBOC could protect against vascular leakage after haemorrhagic shock.
The role of the endothelial glycocalyx in the protective effects of HBOC on vascular permeability
The structure of the endothelial glycocalyx in pulmonary veins was destroyed after haemorrhagic shock; the thickness of the endothelial glycocalyx was reduced by 80.79% compared with the sham operation group (P<0.01) (). LR infusion did not improve the structure of the endothelial glycocalyx. The structure of the pulmonary vascular endothelial glycocalyx was restored after HBOC infusion compared with the LR group; the thickness was increased 2.56 times (P<0.01) (). The levels of HA, HS and CS, the main components of the endothelial glycocalyx, decreased by 58.53, 78.12 and 52.12%, respectively, after haemorrhagic shock compared with the operation group (P<0.01,P<0.01, P<0.01) (). HBOC resuscitation resulted in increases in HA, HS and CS levels in the endothelial glycocalyx after haemorrhagic shock of 60.84, 145.71 and 54.32%, respectively, compared with the LR group (P<0.01,P<0.01, P<0.01). LR infusion did not increase the levels of HA, HS and CS in the endothelial glycocalyx (P<0.01,P<0.01, P<0.01) (). The plasma HA, HS and CS levels, which reflect the severity of the injury, increased significantly after haemorrhagic shock (). After HBOC resuscitation, the levels decreased by 47.30, 44.40 and 37.03%, respectively, compared with the LR group (P<0.01,P<0.01, P<0.01) ().
Figure 2. The protective effects of HBOC on the endothelial glycocalyx in pulmonary veins. (A) The structure of the endothelial glycocalyx in pulmonary veins after haemorrhagic shock was observed by transmission electron microscopy. The endothelial glycocalyx was enhanced by Alcian Blue staining before fixation (bar = 500 nm) (n = 8 observations per group). (B) Hyaluronic acid (HA, green), heparan sulphate (HS, green) and chondroitin sulphate (CS, green) levels in pulmonary veins after haemorrhagic shock (bar = 50 μm). (C) Plasma HA, HS and CS levels, as measured by ELISA (n = 8 observations per group). Data are presented as mean ± SD, each group contains eight observations, *p < 0.05 and **p < 0.01 as compared with sham operation group, #p < 0.05 and ##p < 0.01 as compared with shock group, @p < 0.05 and @@p < 0.01 as compared with LR group. CS: chondroitin sulphate; GCX: glycocalyx; HA: hyaluronic acid; HS: heparan sulphate; HBOC: haemoglobin-based oxygen carrier; LR: Lactated Ringer's solution; Sham: sham operation group.
The HA, HS and CS levels in endothelial glycocalyx VECs decreased by 61.91, 67.98 and 67.36%, respectively, after hypoxia compared with the normal group (). HBOC incubation increased these levels by 63.56, 55.92 and 88.90%, respectively, compared with the LR group. LR incubation did not increase these levels ().
Figure 3. The protective effects of HBOC on the endothelial glycocalyx in hypoxic VECs. (A–D) Hyaluronic acid (HA, green, A), heparan sulphate (HS, green, B) and chondroitin sulphate (CS, green, C) levels in VECs (bar = 25 μm) (n = 8 observations per group). Data are presented as mean ± SD, each group contains eight observations, *p < 0.05 and **p < 0.01 as compared with normal group, #p < 0.05 and ##p < 0.01 as compared with hypoxia group, @p < 0.05 and @@p < 0.01 as compared with LR group. CS: chondroitin sulphate; HBOC: haemoglobin-based oxygen carrier; HA: hyaluronic acid; HS: heparan sulphate; LR: Lactated Ringer's solution; Nor: normal group; VECs: vascular endothelial cells.
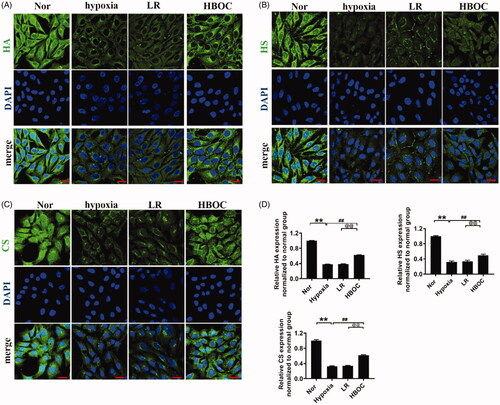
To further explore the role of the endothelial glycocalyx in the protective effects of HBOC against pulmonary vascular permeability after haemorrhagic shock, the endothelial glycocalyx components were degraded by incubation with heparanase III (2 U/ml), hyaluronidase (25 U/ml) and neuraminidase (0.83 U/ml) in serum-free medium at 37 °C for 6 h [Citation14]. This significantly antagonised the protective effects of HBOC on the transmittance of FITC-BSA of hypoxic VECs, which decreased by 18.25% (hyaluronidase + HBOC group), 15.08% (heparanase III + HBOC group) and 15.46% (neuraminidase + HBOC group) compared with the HBOC group (P<0.05, P<0.05, P<0.05) (). These results indicate that the endothelial glycocalyx plays an important role in the protective effects of HBOC against vascular permeability.
Figure 4. The role of glycocalyx in the protective effects of HBOC against vascular permeability. (A) Effects of endothelial glycocalyx inhibitors on TER of VECs after hypoxia and HBOC treatment (n = 8 observations per group). (B) Effects of endothelial glycocalyx inhibitors on the transmittance of FITC-BSA in monolayer VECs after hypoxia and HBOC treatment (n = 8 observations per group). Data are presented as mean ± SD, *p < 0.05 and **p < 0.01 as compared with HBOC group. HBOC: haemoglobin-based oxygen carrier; Hya: hyaluronidase; Hep: heparanase III; Neu: neuraminidase; Nor: normal group; TER: transendothelial electrical resistance; VEC: vascular endothelial cells.
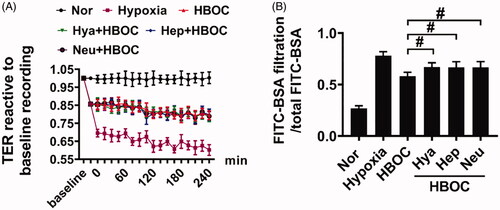
The roles of ZO-1, VE-cadherin and occludin in the protective effects of HBOC against vascular permeability
The expression levels of the intercellular junction proteins ZO-1, VE-cadherin and occludin in VECs were significantly decreased after haemorrhagic shock or hypoxia. They were reduced by 53.32, 63.07 and 64.39% in vivo (P<0.01,P<0.01, P<0.01) and by 60.52, 60.20 and 62.45% in vitro (P<0.01,P<0.01, P<0.01) (). HBOC treatment increased the expression of the intercellular junction proteins by 53.89, 61.36 and 60.81% in vivo (P<0.01,P<0.01, P<0.01) and by 71.86, 42.28 and 44.66% in vitro (P<0.01,P<0.01, P<0.01) compared with the LR group (). These results indicate that intercellular junctions mediate the protective effects of HBOC against vascular permeability.
Figure 5. Effects of HBOC on the expression of intercellular junction proteins in haemorrhagic shock/hypoxia. (A) ZO-1 levels (green) after haemorrhagic shock (bar = 50 μm). (B) VE-cadherin and occludin levels after haemorrhagic shock. (C) Quantification of ZO-1, VE-cadherin and occludin levels in vivo. (D) ZO-1 levels (green) after hypoxia treatment (bar = 10 μm). (E) VE-cadherin and occludin levels after hypoxia treatment. (F) Quantification of ZO-1, VE-cadherin and occludin levels in vitro. Data are presented as mean ± SD (n = 8 observations per group). Date are presented as mean ± SD, each group contains eight observations, *p < 0.05 and **p < 0.01 as compared with sham operation group/normal group, #p < 0.05 and ##p < 0.01 as compared with shock/hypoxia group, @p < 0.05 and @@p < 0.01 as compared with LR group. HBOC: haemoglobin based oxygen carrier; LR: lactated Ringer's solution; Nor: normal group; Sham: sham operation group.
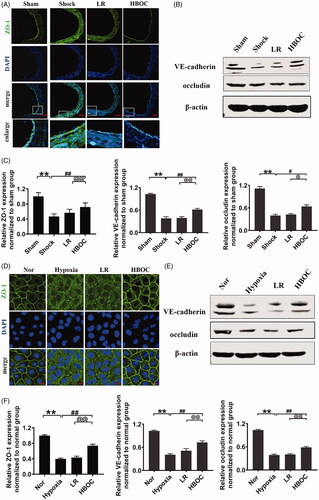
Protective effects of HBOC on VEC mitochondrial function and structure
The oxygen delivery and consumption in rats decreased by 76.14 and 72.61%, respectively, after haemorrhagic shock compared with the normal group (P<0.01, P<0.01). HBOC infusion increased oxygen delivery and consumption by 1.96 and 1.36 times, respectively, compared with the LR group (P<0.01, P<0.01) ().
Figure 6. The protective effects of HBOC on oxygen delivery and oxygen consumption in haemorrhagic shock rats (n = 8 observations per group). Data are presented as mean ± SD, *p < 0.05 and **p < 0.01 as compared with normal group/sham operation group, #p < 0.05 and ##p < 0.01 as compared with shock group/hypoxia group; @p < 0.05 and @@p < 0.01 as compared with LR group. DO2: oxygen delivery; hypoxia: hypoxia group; HBOC: haemoglobin-based oxygen carrier; LR: Lactated Ringer's solution; Nor: normal group; Sham: sham operation group; VO2: oxygen consumption.
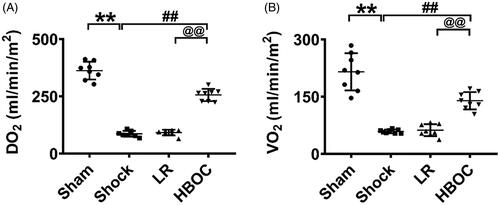
Mitochondria are essential organelles, and mitochondrial dysfunction plays a significant role in organ dysfunction induced by haemorrhagic shock. Mitochondrial function and structure of VECs after hypoxia and HBOC incubation were examined. Following hypoxia, the mitochondrial membrane potential in VECs was decreased by 95.42%, the ATP levels were decreased by 93.09% and the oxygen consumption rate was decreased by 28.60% (P<0.01, P<0.01) (Figure 7(A–C)). HBOC improved mitochondrial function (P<0.01, P<0.01), and LR infusion did not (). Moreover, mitochondria in VECs showed a fragmented structure after hypoxia. HBOC incubation abolished hypoxia-induced mitochondrial fragmentation (P<0.01), whereas LR did not improve mitochondrial structure (). These results indicate that HBOC could increase oxygen delivery and consumption by improving mitochondrial structure and function in VECs.
Figure 7. The protective effects of HBOC on mitochondria in hypoxic VECs. (A) The effects of HBOC on the membrane potential of mitochondria in hypoxic VECs (bar = 25 μm). (B) The effects of HBOC on the ATP levels in hypoxic VECs. (C) The effects of HBOC on the oxygen consumption rate in hypoxic VECs. (D) The protective effects of HBOC on the morphology of mitochondria in hypoxic VECs (bar = 25 μm). Mitochondria were considered short when the length of mitochondria < 7 μm and long when the length of mitochondria > 7 μm. Data are presented as mean ± SD (n = 8 observations per group). *p < 0.05 and **p < 0.01 as compared with normal group/sham operation group, #p < 0.05 and ##p < 0.01 as compared with shock group/hypoxia group; @p < 0.05 and @@p < 0.01 as compared with LR group. ATP: adenosine triphosphate; hypoxia: hypoxia group; HBOC: haemoglobin-based oxygen carrier; LR: lactated Ringer’s solution; Nor: normal group; Sham: sham operation group; VEC: vascular endothelial cells.
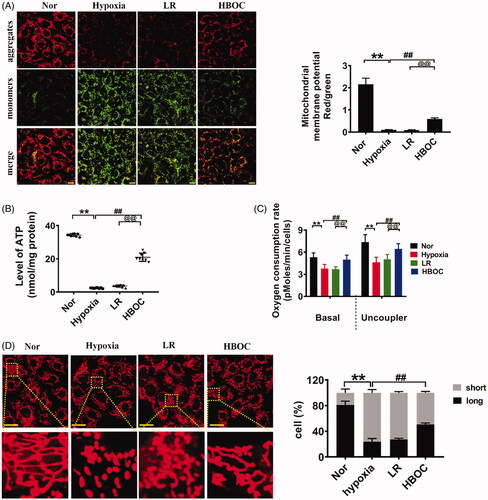
Discussion
In this study, we found that (i) after haemorrhagic shock, vascular leakage was significantly increased and (ii) HBOC had significant protective effects on pulmonary vascular leakage, protecting lung function. Further experiments revealed that the protective effects of HBOC on endothelial permeability were related to the endothelial glycocalyx and intercellular junctions. HBOC protected the endothelial glycocalyx and intercellular junctions by improving mitochondrial function and tissue oxygen delivery.
Haemorrhagic shock is the leading cause of death due to trauma, and massive haemorrhage may lead to systemic hypoxia and insufficient systemic organ perfusion. Fluid resuscitation is an important measure for the early treatment of traumatic haemorrhagic shock patients. Besides crystalloid and colloid fluids, HBOCs are considered to be ideal resuscitation fluids for traumatic haemorrhage and major surgical treatment because of their good volume expansion effect and oxygen-carrying properties [Citation15,Citation16]. Previous studies found that HBOC had excellent therapeutic effects on haemorrhagic shock. In a previous study, we found that HBOC could increase the survival rates of rats and pigs with a blood loss of 50% compared with the LR treatment group [Citation11]; other studies found that bovine polymerized haemoglobin significantly improved the 72-h survival rate in pigs compared with hydroxyethyl starch (HES) [Citation17]. Similar results were obtained in the study by Li et al., who showed that the survival rate of the HBOC group was significantly higher than that of the HES group in haemorrhagic shock Sprague Dawley rats [Citation18]. In addition to improving the survival rate, HBOC also has protective effects on organ function in various diseases. A study by Li and Kuang found that HBOC had excellent protective effects on the liver, kidney, brain and other important organs in rats with haemorrhagic shock and sepsis [Citation11,Citation19]; Li et al.’s study found that HBOC had protective effects on heart, lung, liver and kidney function of Beagle dogs after ischaemia–reperfusion [Citation16]. Masuno et al. [Citation20] also found that HBOC treatment could reduce the incidence of lung injury in rats with haemorrhagic shock. In this study, we found that HBOC had significant protective effects against pulmonary vascular leakage in haemorrhagic shock, and protected lung function by improving pulmonary vascular leakage. Our experimental results are based on animal experiments. The results will provide some evidence for the later use of HBOC in clinical research. Unfortunately, we did not carry out the research on the role of HBOC in related clinical problems.
Moreover, we found that HBOC could improve oxygen delivery and consumption and protect mitochondrial function after haemorrhagic shock. Previous studies found that HBOC improved oxygen delivery and consumption of the body by carrying oxygen. Our previous studies found that HBOC could improve oxygen delivery and consumption in rats with haemorrhagic shock and sepsis [Citation11,Citation19]; Li et al.’s [Citation16] study found that HBOC could very much improve the survival rate of haemorrhagic shock as well as the PaO2 of the body. The present study showed that HBOC could improve mitochondrial function after haemorrhagic shock. Mitochondria could undergo fission and fusion to adapt to changes in the extracellular environment. Under hypoxic conditions, mitochondria divide into smaller organelles, promoting mitochondrial autophagy, keeping the production of reactive oxygen species at a low physiological level and maintaining the integrity of mitochondria by reducing respiratory activity [Citation21]. The present study found that HBOC infusion could protect mitochondrial structure and restore hypoxia-induced structural damage. However, the mechanisms underlying these protective effects remain unclear.
Many studies on the morphological regulation of mitochondrial fragmentation have been published. Dynamin-related protein 1 (Drp1), mitochondrial fission 1 protein (Fis1), mitochondrial fission factor (mff), mitofusin 1 (Mfn1) and Mfn2 play important roles in the continuous mitochondrial fission and fusion under physiological conditions. Drp1 is abundantly expressed in adult skeletal muscle, heart, kidney and brain, and activated Drp1 is transferred to extracorporeal mitochondrial membranes to promote mitochondrial fission [Citation22]. It has been shown that the effects of Drp1 on mitochondrial fission are regulated by phosphorylation of proteins in the ERK, cyclin/CDK, MAPK and RhoA/ROCK pathways. For example, Javier et al. [Citation23] found that the phosphorylation of Drp1-S616 was induced by the activation of ERK1/2, which promoted mitochondrial fission in the early stage of cell recombination; inhibition of Drp1 activity by phosphorylation of Cdk5 at Ser616 in mature neurons could inhibit mitochondrial fission [Citation24]. However, how HBOC acts on Drp1 and Mfn after haemorrhagic shock remains unclear. Some studies found that haem oxygenase-1 (HO-1) could regulate mitochondrial fission. HO-1 could affect the dynamic balance of mitochondrial fission and fusion in the lung and heart [Citation25–27]. Yu et al. demonstrated that HO-1 could inhibit excessive mitochondrial fission induced by LPS and attenuate lung injury induced by endotoxin [Citation25]; further studies found that HO-1/carbon monoxide releasing molecule-2 (CORM-2) play an important role in the regulation of mitochondrial structure. Shi et al. [Citation28] found that in LPS-stimulated alveolar macrophages, HO-1/CORM-2 could reduce the mRNA and protein levels of Fis1 by upregulating carbon monoxide production, increase Mfn1/Mfn2 expression [Citation26], and reduce mitochondrial fission. Moreover, it has been reported that HBOC could increase HO-1 expression [Citation29,Citation30]. Sagar et al. found that HBOC could significantly increase HO-1 in a dose-dependent manner in cardiac cardiomyocytes and alveolar macrophages in a rat model of ischaemia–reperfusion [Citation31]. In addition, it has been found that HBOC could induce HO-1 expression in pulmonary vascular cells in vitro [Citation29]. Whether HBOC upregulates the expression of Drp1 and Mfn through the HO-1/CORM-2 pathway, thereby regulating mitochondrial fission and protecting mitochondrial function, is a question that needs further study.
Vascular leakage is a common complication after haemorrhagic shock and fluid resuscitation. The endothelial glycocalyx plays an important role in the vascular barrier. In this study, we found that HBOC could improve pulmonary vascular leakage induced by haemorrhagic shock by protecting the structure and composition of the endothelial glycocalyx. However, the mechanisms underlying these effects remain to be elucidated. On the one hand, HBOC increases tissue oxygen delivery and consumption, improves mitochondrial function and directly increases the metabolism of the endothelial glycocalyx. On the other hand, in the dynamic structure of the endothelial cell surface, many factors can result in structural changes, leading to endothelial glycocalyx shedding and ultimately loss of the physiological function of the endothelial glycocalyx [Citation4]. It has been shown that overexpression of matrix metalloproteinases (MMPs) under pathological conditions directly destroys the composition of endothelial glycocalyx, resulting in structural and functional damage of the endothelial glycocalyx. Kwon et al. reported that MMP-2, MMP-7 and MMP-9 directly affect the molecular structure of CS in the endothelial glycocalyx, resulting in an abnormal structure of the endothelial glycocalyx and reducing the function of the endothelial glycocalyx in colon cancer cells [Citation32,Citation33]. Another study found that MMP-1 reduced the HS content on the cell surface by acting on the anchor protein syndecan-1 [Citation33]; Kwon et al. found that IL-1α regulates the expression of MMP-7 in colon cancer cells, inducing the shedding of syndecan-2 on the HS chain, which in turn destroyed the HS chain [Citation34]. It remains unclear whether the protective effects of HBOC on the endothelial glycocalyx after haemorrhagic shock are mediated by MMPs. Recent studies found that HBOC reduces the activation of MMP-9 in rats with transient cerebral ischaemia [Citation35], indicating that the protective effects of HBOC on the endothelial glycocalyx may be mediated by MMPs.
Intercellular junctions play an important role in vascular leakage [Citation3]. In this study, we found that HBOC protects against vascular permeability by upregulating the expression of the intercellular junction proteins ZO-1, VE-cadherin and occludin after haemorrhagic shock; however, it remains unclear how these effects are achieved. Chi et al. [Citation36] found that an increase in HO-1 levels could restore the function of tight junctions and alleviate intestinal ischaemia–reperfusion injury in a rat liver transplantation model. In vitro experiments indicated that HO-1 could upregulate the expression of ZO-1 in human umbilical vein endothelial cells after oxidative stress [Citation37]. Further studies found that the HO-1/CORM-2 pathway participates in the regulation of intercellular junctions by HO-1 [Citation38,Citation39]. Interestingly, HBOC could regulate HO-1 expression [Citation29–31]. However, the question whether HBOC regulates the expression of intercellular junction proteins such as ZO-1 through HO-1 requires further study.
Limitations
In this study, we found that HBOC protects against pulmonary vascular leakage in haemorrhagic shock. However, we wish to mention several limitations. We did not study the mechanisms by which HBOC protects the endothelial glycocalyx and intercellular junctions. Moreover, the association of the endothelial glycocalyx and intercellular junctions with mitochondrial function is not clear. Thirdly, some findings and conclusions were based on results obtained in vitro, under conditions that do not fully reproduce the physiological conditions encountered in vivo.
Conclusion
HBOC has protective effects against pulmonary vascular leakage; the protective effects of HBOC against endothelial leakage are related to the endothelial glycocalyx and intercellular junctions. HBOC protects the endothelial glycocalyx and intercellular junctions by improving mitochondrial function and tissue oxygen delivery.
Authors’ contributions
LiangMing Liu and Tao Li contributed to the conception and design, revision of the article. Yu Zhu, Jie Zhang, Yue Wu, XinMing Xiang, QingGuang Yan, XiaoYong Peng, KunLun Tian contributed to animal experiment. Hongliang Zhao contributed to animal experiment, collection and processing of samples, drafting and revision of the article. All the authors read and approved the final article.
Supplymental_material.docx
Download MS Word (27.2 KB)Disclosure statement
The authors reported no proprietary or commercial interest in any product mentioned or concept discussed in this article.
Additional information
Funding
References
- Yang G, Peng X, Wu Y, et al. Involvement of connexin 43 phosphorylation and gap junctional communication between smooth muscle cells in vasopressin-induced ROCK-dependent vasoconstriction after hemorrhagic shock. Am J Physiol Cell Physiol. 2017;313(4):C362–C370.
- Thompson CL, Angelides KJ, Velazquez JL, et al. Distribution, mobility, and function of benzodiazepine receptors on primary cultures of vertebrate neurons. Adv Biochem Psychopharmacol. 1988;45:135–149.
- Duan CY, Zhang J, Wu HL, et al. Regulatory mechanisms, prophylaxis and treatment of vascular leakage following severe trauma and shock. Mil Med Res. 2017;4:11.
- Mehta D, Malik AB. Signaling mechanisms regulating endothelial permeability. Physiol Rev. 2006;86(1):279–367.
- Tsukita S, Furuse M. Pores in the wall: claudins constitute tight junction strands containing aqueous pores. J Cell Biol. 2000;149(1):13–16.
- Trieu M, van Meurs M, van Leeuwen A, et al. Vasculotide, an angiopoietin-1 mimetic, restores microcirculatory perfusion and microvascular leakage and decreases fluid resuscitation requirements in hemorrhagic shock. Anesthesiology. 2018;128(2):361–374.
- Bougle A, Harrois A, Duranteau J. Resuscitative strategies in traumatic hemorrhagic shock. Ann Intensive Care. 2013;3(1):1.
- Rizoli SB. Crystalloids and colloids in trauma resuscitation: a brief overview of the current debate. J Trauma. 2003;54(5 Suppl):S82–S88.
- Standl T, Freitag M, Burmeister MA, et al. Hemoglobin-based oxygen carrier HBOC-201 provides higher and faster increase in oxygen tension in skeletal muscle of anemic dogs than do stored red blood cells. J Vasc Surg. 2003;37(4):859–865.
- McNeil CJ, Smith LD, Jenkins LD, et al. Hypotensive resuscitation using a polymerized bovine hemoglobin-based oxygen-carrying solution (HBOC-201) leads to reversal of anaerobic metabolism. J Trauma. 2001;50:1063–1075.
- Li T, Yang G, Zhu Y, et al. Beneficial effects of novel cross-linked hemoglobin YQ23 on hemorrhagic shock in rats and pigs. J Surg Res. 2017;210:213–222.
- Zhu Y, Zhang J, Wu Y, et al. Beneficial effects of hemoglobin-based oxygen carriers on early resuscitation in rats with uncontrolled hemorrhagic shock. Zhonghua Wei Zhong Bing Ji Jiu Yi Xue. 2019;31:81–86.
- Alphonsus CS, Rodseth RN. The endothelial glycocalyx: a review of the vascular barrier. Anaesthesia. 2014;69(7):777–784.
- Mockl L, Hirn S, Torrano AA, et al. The glycocalyx regulates the uptake of nanoparticles by human endothelial cells in vitro. Nanomedicine (Lond). 2017;12:207–217.
- Elmer J, Alam HB, Wilcox SR. Hemoglobin-based oxygen carriers for hemorrhagic shock. Resuscitation. 2012;83(3):285–292.
- Li Q, Li S, Yang Q, et al. Hemoglobin-based oxygen carrier attenuates cerebral damage by improving tissue oxygen preload in a dog model of cardiopulmonary bypass. Artif Cells Nanomed Biotechnol. 2015;43(2):87–92.
- Philbin N, Rice J, Gurney J, et al. A hemoglobin-based oxygen carrier, bovine polymerized hemoglobin (HBOC-201) versus hetastarch (HEX) in a moderate severity hemorrhagic shock swine model with delayed evacuation. Resuscitation. 2005;66(3):367–378.
- Li Y, Yan D, Hao S, et al. Polymerized human placenta hemoglobin improves resuscitative efficacy of hydroxyethyl starch in a rat model of hemorrhagic shock. Artif Cells Nanomed Biotechnol. 2015;43(3):174–179.
- Kuang L, Zhu Y, Zhang J, et al. A novel cross-linked haemoglobin-based oxygen carrier is beneficial to sepsis in rats. Artif Cells Nanomed Biotechnol. 2019;47(1):1496–1504.
- Masuno T, Moore EE, Cheng AM, et al. Prehospital hemoglobin-based oxygen carrier resuscitation attenuates postinjury acute lung injury. Surgery. 2005;138(2):335–341.
- Fuhrmann DC, Brune B. Mitochondrial composition and function under the control of hypoxia. Redox Biol. 2017;12:208–215.
- Ikeda Y, Shirakabe A, Brady C, et al. Molecular mechanisms mediating mitochondrial dynamics and mitophagy and their functional roles in the cardiovascular system. J Mol Cell Cardiol. 2015;78:116–122.
- Prieto J, Leon M, Ponsoda X, et al. Early ERK1/2 activation promotes DRP1-dependent mitochondrial fission necessary for cell reprogramming. Nat Commun. 2016;7:11124.
- Taguchi N, Ishihara N, Jofuku A, et al. Mitotic phosphorylation of dynamin-related GTPase Drp1 participates in mitochondrial fission. J Biol Chem. 2007;282(15):11521–11529.
- Yu J, Shi J, Wang D, et al. Heme oxygenase-1/carbon monoxide-regulated mitochondrial dynamic equilibrium contributes to the attenuation of endotoxin-induced acute lung injury in rats and in lipopolysaccharide-activated macrophages. Anesthesiology. 2016;125(6):1190–1201.
- Hull TD, Boddu R, Guo L, et al. Heme oxygenase-1 regulates mitochondrial quality control in the heart. JCI Insight. 2016;1(2):e85817
- Hong JM, Lee SM. Heme oxygenase-1 protects liver against ischemia/reperfusion injury via phosphoglycerate mutase family member 5-mediated mitochondrial quality control. Life Sci. 2018;200:94–104.
- Shi J, Yu J, Zhang Y, et al. Phosphatidylinositol 3-kinase-mediated HO-1/CO represses Fis1 levels and alleviates lipopolysaccharide-induced oxidative injury in alveolar macrophages. Exp Ther Med. 2018;16(3):2735–2742.
- Cheng AM, Moore EE, Johnson JL, et al. Polymerized hemoglobin induces heme oxygenase-1 protein expression and inhibits intercellular adhesion molecule-1 protein expression in human lung microvascular endothelial cells. J Am Coll Surg. 2005;201(4):579–584.
- Babu AN, Damle SS, Moore EE, et al. Hemoglobin-based oxygen carrier induces hepatic heme oxygenase 1 expression in Kupffer cells. Surgery. 2007;142(2):289–294.
- Damle SS, Moore EE, Babu AN, et al. Hemoglobin-based oxygen carrier induces heme oxygenase-1 in the heart and lung but not brain. J Am Coll Surg. 2009;208(4):592–598.
- Gronski TJ, Martin RL, Kobayashi DK, et al. Hydrolysis of a broad spectrum of extracellular matrix proteins by human macrophage elastase. J Biol Chem. 1997;272(18):12189–12194.
- Kuehn R, Kaczensky P, Lkhagvasuren D, et al. Differentiation of meat samples from domestic horses (Equus caballus) and Asiatic Wild Asses (Equus hemionus) using a species-specific restriction site in the mitochondrial cytochrome b region. Mong J Biol Sci. 2006;4(2):57–62.
- Kwon MJ, Hong E, Choi Y, et al. Interleukin-1α promotes extracellular shedding of syndecan-2 via induction of matrix metalloproteinase-7 expression. Biochem Biophys Res Commun. 2014;446(2):487–492.
- Gekka M, Abumiya T, Komatsu T, et al. Novel hemoglobin-based oxygen carrier bound with albumin shows neuroprotection with possible antioxidant effects. Stroke. 2018;49(8):1960–1968.
- Chi X, Yao W, Xia H, et al. Elevation of HO-1 expression mitigates intestinal ischemia-reperfusion injury and restores tight junction function in a rat liver transplantation model. Oxid Med Cell Longev. 2015;2015(2015):986075.
- Li J, Zhou J, Tian B, et al. Activation of HO-1 protects placental cells function in oxidative stress via regulating ZO-1/occludin. Biochem Biophys Res Commun. 2019;511(4):903–909.
- Zhang L, Zhang Z, Liu B, et al. The protective effect of heme oxygenase-1 against intestinal barrier dysfunction in cholestatic liver injury is associated with NF-κB inhibition. Mol Med. 2017;23:215–224.
- Akagi R, Akagi M, Hatori Y, et al. Prevention of barrier disruption by heme oxygenase-1 in intestinal bleeding model. Biol Pharm Bull. 2016;39(6):1007–1012.