Abstract
Severe acute respiratory syndrome coronavirus 2 (SARS-CoV-2) is a zoo tonic, highly pathogenic virus. The new type of coronavirus with contagious nature spread from Wuhan (China) to the whole world in a very short time and caused the new coronavirus disease (COVID-19). COVID-19 has turned into a global public health crisis due to spreading by close person-to-person contact with high transmission capacity. Thus, research about the treatment of the damages caused by the virus or prevention from infection increases everyday. Besides, there is still no approved and definitive, standardized treatment for COVID-19. However, this disaster experienced by human beings has made us realize the significance of having a system ready for use to prevent humanity from viral attacks without wasting time. As is known, nanocarriers can be targeted to the desired cells in vitro and in vivo. The nano-carrier system targeting a specific protein, containing the enzyme inhibiting the action of the virus can be developed. The system can be used by simple modifications when we encounter another virus epidemic in the future. In this review, we present a potential treatment method consisting of a nanoparticle–ribozyme conjugate, targeting ACE-2 receptors by reviewing the virus-associated ribozymes, their structures, types and working mechanisms.
Introduction
Since the earlier twenty-first century, three kinds of coronaviruses have affected humanity through deadly pneumonia: in 2003, SARS-CoV appeared in the Guangdong province in China affected 29 countries around the world and 8098 people were infected with a 9% mortality rate. In 2012, MERS-CoV emerged in Saudi Arabia, spread to 27 countries, and reported a total number of 2494 laboratory-confirmed cases with a 35% mortality rate [Citation1,Citation2]. In the last month of 2019, the first case of coronavirus disease 2019 (COVID-19) was confirmed by a new form of SARS-CoV, named severe acute respiratory syndrome coronavirus 2 (SARS-CoV-2), reported in the Wuhan city of China. The source of the virus has not confirmed yet; however, sequence-based studies showed high similarities with the bats’ sequence and the SARS-CoV-2 [Citation3]. At the end of 2020, scientists have found a new SARS-CoV-2 variant with multiple mutations in the UK and named as “VUI − 202012/01” (variant under investigation) [Citation4].
WHO declared the COVID-19 outbreak as an Internationally Significant Public Health Emergency on 30 January 2020, taking into account the disease danger and the rate of spreading. There is no doubt that many countries are suffering from COVID-19 and fighting against the pandemic. Currently, SARS-CoV-2 has affected more than 100 million people with a 4.5% mortality rate in 222 countries worldwide (data obtained by WHO from national authorities at 10:00 CEST, 31 January 2021) and the number of cases is still increasing.
Meanwhile, the value of R0 is 2 and 5 for SARS and 0.3–0.8 for MERS. Moreover, the estimated R0 value for COVID-19 was obtained from the evaluation of 12 separate studies published between January and February. The R0 value of COVID-19 was found between 1.4 and 6.49. Depending on the R values, the contagion effect of SARS-CoV-2 is significantly rapid than SARS-CoV or MERS-CoV [Citation5–7]. The incubation period for SARS-CoV-2 is on average 5–6 days up to 14 days. According to related published articles, people infected with SARS-CoV-2 spread the virus to others through close contact or respiratory droplets or contaminated various surfaces and people who had contact with these surfaces were infected by the virus [Citation8–11]. Asymptomatic infections are critical for the spreading of COVID-19 [Citation12–14]. WHO defines an asymptomatic case as “A laboratory-confirmed infected person without clear symptoms” (data obtained by WHO from national authorities at 10:00 CEST, 2 April 2020). The estimating proportion of asymptomatic cases ranges from 8 to 80% [Citation15]. The reason for the wide range is the lack of sufficient studies yet.
COVID-19 presents with symptoms ranging from asymptomatic/mild symptoms to severe illness and death. The symptoms were mostly related to respiratory symptoms including fever, cough, difficulty in breathing, loss of sense of smell or taste, gasping, fatigue and diarrhoea. In more severe cases, symptoms such as acute respiratory failure, pneumonia, persistent chest pain or pressure have been observed [Citation6,Citation16,Citation17]. A meta-analysis study on the prevalence of COVID-19 symptoms in adults demonstrated the prevalence of symptoms in the systemic, respiratory, ear, nose, throat, gastrointestinal, eyes and central nervous system [Citation6].
SARS-CoV-2 has several proteins: structural spike (S) protein, membrane (M) protein, nucleocapsid (N) protein and envelope (E) protein. PP1a and PP1b, two viral replicase polyproteins, are produced that are processed into 16 mature non-structural proteins (NSPs). The Spike protein is a glycoprotein found on the outer surface of the virus and responsible for the recognition, attachment and entry to host cells [Citation18]. shows the structure of the spike (S) protein. Overall, the perspective on anti-viral treatment strategies for COVID-19 infections is targeting spike (S) protein inhibition. Inhibitors are commonly used in anti-viral treatments. However, repeated virus mutations and viruses escaping immune system cells may be ineffective in binding viral inhibitors to their targets and cause toxicity and serious side effects due to non-specific binding [Citation19–23]. At the same time, the interaction of inhibitors with other molecules can lead to low efficacy [Citation24].
Figure 1. Structure of coronavirus spike glycoprotein and spike glycoprotein-ACE2 receptor complex spike glycoprotein mediates SARS-CoV-2 entry into the host cells. SARS-CoV-2 spike protein binds to its receptor ACE2 through its receptor-binding domain (RBD). The figure shows the closed and open conformations of the spike glycoprotein structure and spike protein–ACE2 receptor complex, respectively. The RBD (receptor binding domain) region binds to the ACE2 receptor, allowing the closed conformation to transition into an open conformation (created with BioRender.com).
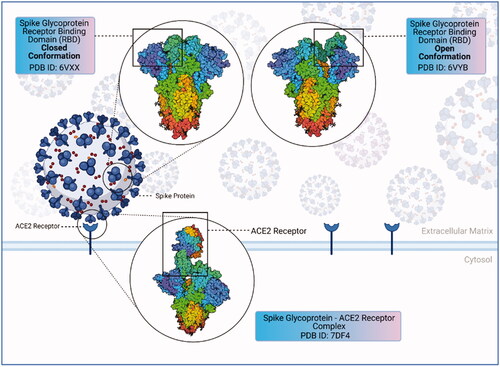
There are ongoing research studies to combat the virus and some clinical trials with efficient results were completed [Citation25–29]. To date, several vaccines against SARS-CoV-2 have developed as DNA-mRNA based, via viral vectors, recombinant protein, inactivated virus and other vaccine types [Citation30]. The U.S. Food and Drug Administration (FDA) has issued an emergency use authorization (EUA) for the drugs and vaccine candidates against COVID-19. Both Pfizer-BioNTech’s and Moderna’s lipid nanoparticle-encapsulated oligonucleotide based mRNA vaccines BNT162b2 and mRNA-1273 are among these candidates [Citation31]. The vaccine formulation of mRNAs encoding the SARS-CoV-2 Spike glycoprotein-receptor binding domain (RBD) in a trimerized manner was carried out by the company Pfizer-BioNTech [Citation32]. The mRNAs transferred via lipid nanoparticles have been modified with nucleoside. Both of the vaccines’ phase III results revealed efficacy of approximately 95% [Citation30,Citation33]. Besides, baricitinib in combination with remdesivir has been shown in clinical studies to abbreviate the recovery time compared to patients receiving remdesivir-placebo. The safety and effectiveness of this research therapy for use in the treatment of COVID-19 continue to be evaluated [Citation34–36].
Nevertheless, new methods have to develop for the treatment of SARS-CoV-2. The nucleic acid-based drugs as antisense oligonucleotide based systems, siRNA based drugs or ribozymes are promising agents. The development of a specific antiviral therapy against SARS-CoV-2 would be a promising therapy. Recently, the mentioned technologies that include ribozymes and their therapeutic potentials have been tested. [Citation37–40].
RNA interference
RNA interference (RNAi) is a post-transcriptional gene silencing mechanism resulting from the breakdown of the complementary mRNA sequence when double-stranded RNA (dsRNA) enters the cell [Citation41]. RNAi is a biological process inhibiting gene expression by targeting specific RNA molecules. RNA interference helps to defend the cell genome by protecting it against the invasion of virus genetic material or transposons from jumping to different locations within the genome [Citation42]. RNAi is a favourable system compared to other antisense oligonucleotide treatments with targeting specificity and ease of modifying target regions [Citation43]. In 2001, RNAi was selected as the "molecule of the year" and "the most important scientific move of 2002" by Science magazine. Besides, in 2006, researchers Andrew Z. Fire and Craig C. Mello received the Nobel Prize in Physiology/Medicine for their work on RNAi [Citation44].
The main RNAi agents responsible for RNAi can be listed as follows:
miRNAs
Twenty to 30 base length miRNAs are RNAi elements formed by the cleavage of the secondary structured miRNA precursor by endoribonucleases. They inhibit the translation process of mRNA by binding specifically to the ribosome binding site of the mRNA [Citation45]. miRNAs have been used to attenuate viruses that are the main component of attenuated vaccines [Citation46]. These approaches, which have the potential to increase vaccine safety, suggest that they may be useful in controlling viral attenuation and pathogenesis [Citation47,Citation48]. There are identified miRNAs that bind to specific regions of the SARS-CoV-2, SARS-CoV and MERS-CoV genomes and inhibit viral infections caused by the viruses. Additionally, specific miRNAs have been developed against the S protein of SARS-CoV-2 and, in the meantime miRNA inhibitors that target viral miRNAs are also used for this process [Citation49].
Small interference RNA
Twenty to 25 nucleotide length small interference RNA (siRNA) molecules are highly similar to the miRNA structure. siRNAs inhibit protein formation via inhibiting the translation process by breaking down specific nucleotides of mRNA [Citation50]. siRNAs with over 70% interference ability against the expression of M, N and E gene regions in the SARS-CoV-2 genome have been discovered. Besides, six siRNAs targeting the replicase 1A gene region with 50–90% interference ability have been obtained to date [Citation51].
Short-hairpin RNA
Short-hairpin RNA (shRNA) molecules are artificial synthetic RNAs in a short-hairpin structure. shRNA molecules are the precursors of siRNAs and, they cleaved by endoribonucleases to form siRNAs [Citation52]. It is aimed to prevent and treat viral infections with shRNA targeting protected gene regions between ORF3 and mutant strains in the SARS-CoV genome [Citation53].
Antisense RNAs are the molecules initiating the RNAi process. Single chain, naturally synthesizable or synthetically produced anti-sense RNA molecules bind to the target transcript molecule by specific base coupling [Citation54]. The ability of siRNA to target and silence any related gene has made siRNA a powerful tool, especially for the treatment of viral infections. Besides, detecting SARS-CoV-2 in samples by targeting gene regions associated with replication or transcription via anti-sense RNA molecules is also possible [Citation55]. The use of the molecules can be effective in reducing the severity of infection in the body and preventing SARS-CoV-2 related diseases. For example, targeting the ORF1a and ORF1b regions with anti-sense RNA molecules can be evaluated within therapeutic approaches. Studies have shown that siRNAs directed at various tissues are successful in silencing target gene regions [Citation56]. RNA aptamers are molecules involved in the RNAi process. RNA aptamers bind specifically to target molecules by hybridization, initiating the RNAi process and thus inhibiting subsequent steps [Citation57]. RNA aptamers developed specifically against SARS-CoV can be used to detect SARS-CoV in different samples and help to develop alternative therapy against infections [Citation58].
Ribozymes
Ribozymes are single-stranded non-encoded RNA molecules that bind to the targeted region by the Watson–Crick coupling. They catalyse the hydrolysis of phosphodiester bonds and cause degradation of the target RNA. It was first discovered as a naturally produced molecule in Tetrahymena thermophilia and, Thomas R. Cech and his colleagues received the Nobel Prize in Chemistry with this discovery in 1989 [Citation59]. In addition to the hydrolysis of phosphodiester bonds, ribozymes are also responsible for the formation of peptide bonds in rRNAs, catalysis of intracellular activities such as polymerization and ligation [Citation60].
Ribozymes are hybrid RNA molecules that break down the specifically targeted RNA. They hybridize with the target RNA to reveal a single secondary structure. Once the target has been disrupted, the ribozyme detaches from the disrupted transcript. The mechanism continues by binding the ribozyme to another RNA molecule. Ribozymes present cis or trans activity. They either suppress transcription during cis cutting activity or suppress the transcription during trans activity [Citation61]. The main advantage of ribozymes is the ability to recognize and bind specifically to multiple target mRNA in contrast to the other antisense molecules in RNAi systems [Citation61]. The unique properties of ribozymes in specific and direct inactivation of other RNAs have made them a potential gene suppression tool as promising molecular agents in recent studies [Citation62].
Seven catalytic RNAs have been identified to date that occur naturally. Ribozymes are divided into three groups in terms of function and shape: self-splicing introns, ribonuclease P (RNase P) and small catalytic RNAs [Citation61]. The hammerhead, hairpin, Neurospora Varkud satellite (VS) and hepatitis delta virus (HDV) ribozymes are small RNAs of 50–150 nucleotides that perform site-specific self-cleavage [Citation63]. They are found in RNA genomes as viral, virusoid or satellite a subviral agent and integrate into the genome by rolling ring replication [Citation62].
Although the ribozymes in this group appear to be the same as many other proteinase enzymes, ribozymes only affect specific phosphodiester bonds using their base mapping and other interactions to determine the cut site within their active region [Citation64]. Several hundred nucleotides length group I and II introns and RNase P are larger, structurally more complex ribozymes. These two groups can be distinguished according to their class of introns, splicing mechanisms and secondary structures [Citation65]. Group I introns are part of the precursor rRNA transcripts and do not require a separate component to be removed from the construct. The source of the catalytic activity is in the intron itself. Group II introns are part of the precursor mRNA and tRNA transcripts produced in mitochondria and chloroplasts, and unlike group I introns, they do not require a guanosine cofactor for catalytic activity [Citation66]. We have summarized the types of ribozymes below.
Ribonuclease P
Ribonuclease P is a protected endonuclease found in bacteria, eukaryotes and archaea that catalyse maturation by cleavage of the 5′ end of the tRNA [Citation67]. RNase P in all the domains contains protein subunits together [Citation68]. In the absence of the protein component of RNase P in bacteria and some archaea, the RNA component can catalyse tRNA maturation. Additionally, in 2008, Holzmann et al. showed that human mitochondrial RNase P is only a protein enzyme required for RNA catalysis [Citation69]. Archael RNase Ps contain the catalytic core of bacterial RNase P but lack some stabilizing elements [Citation70]. shows the Escherichia coli RNase P complex.
Hammerhead ribozymes
The hammerhead RNA is a single-stranded RNA motif that undergoes autocatalytic self-cutting, and because of its feature, it is not a real enzyme in the biological context [Citation71]. The terms “hammerhead ribozyme” and “hammerhead RNA” can be used interchangeably [Citation72]. The structure of hammerhead ribozymes consists of three helices called I, II and III attached to an 11-nucleotide length catalytic core. The catalytic core has a substrate recognition area containing two bodies on either side of the dividing region [Citation73]. Hammerhead and hairpin ribozymes are small, sequence-specific RNA endonucleases. They can be manipulated easily for maintaining specificity and regulate catalytic activity [Citation74]. However, a relatively insignificant small connection gains catalytic properties by removing the loop region from the strand and gives the ability to react with multiple substrates. Hammerhead ribozymes are the most remarkable catalytic RNA motifs for gene editing activities [Citation75,Citation76]. Besides, ribozymes have been frequently used in various viral inhibition studies [Citation77–79].
Studies have revealed hammerhead ribozymes are located in 3′-UTR regions of C-type lectin type II (CLEC2) in mammals. Twelve different types of hammerhead ribozymes have been found in three types of rats, two types of mice and tree shrews, hedgehogs, horses, elephants, cows, dogs and platypus [Citation80]. They all have the type III hammerhead ribozyme structure and contain non-conserved intervening sequence regions in the loop I region. Alignment in the sequence and the presence of conserved sequences in the catalytic core indicate that tertiary interactions are critical for catalytic activity [Citation72]. Hammerhead is one of several structural motifs of ribozymes that were initially detected in RNA molecules of several plant viroids and viruses [Citation81]. It has been reported self-division by these RNAs requires only a minimum of 50 nucleotide sequences that can be modelled into a secondary structure resembling a hammerhead [Citation82]. The catalytic sequence contains most of the conserved sequences of the hammerhead. The only requirement for the substrate-cleavage is the presence of a "GUC" sequence. shows the secondary structure of a trans-cleaving hammerhead ribozyme and the cleavage site. A target-specific ribozyme is obtained by designing the root I and III sequences after the target RNA according to simple Watson Crick base matching rules. Under optimal conditions, ribozymes bind only to RNAs with sequences perfectly complementing the root I and root III sequences surrounding the GUC trio [Citation83]. The adjustability of the triple base region allows them to be designed to attack any target RNA. In a study in 2007, ribozymes were used as a drug for therapeutic purposes against the SARS-CoV epidemic wave. Ribozyme containing a complementary base sequence has been designed by using common base sequences in viruses such as SARS-CoV and MHV [Citation84].
Hepatitis delta virus ribozymes
Hepatitis delta virus has circular, genomic and antigenomic 1.7 kb RNA. It replicates its genome with the circular replication mechanism. At the same time, this reaction separates the mRNA for the delta antigen (HDAg), the only encoded protein by the HDV genome, and performs its processing [Citation85]. It performs this separation via an 85-nucleotide ribozyme with digest pseudo therapy in the virus. For this reason, this ribozyme is called the HDV ribozyme [Citation86]. The catalytic activity of the HDV ribozyme is required for viral replication and viral particle assembly within host cells. HDV ribozymes have self-scission property using a combination of metal ion and general acid–base catalysis, with a reaction in which a cytosine nucleobase C75 acts as a general acid and a divalent metal ion as a Lewis acid [Citation87]. The structure of the ribozyme was obtained from crystals in the amodized form of the genomic ribozyme. No physical structure exists for antigenomic ribozyme, but since the secondary structures of genomic and antigenomic ribozymes are very similar, three-dimensional structures are likely to be similar. Each ribozyme contains five duplexes or pairs called as P1, P2, P3, P4 and P1.1. The wide duplex formation and a nested arrangement of the duplex elements form a compact structure. The cleavage site is located at the 5′ end of P1, and embedded in an active pocket between two parallel coaxial stacks of P1 – P1.1 – P4 and P2 – P3 [Citation88]. The short sequence connecting P4 and P2 also forms part of this pocket and contributes to and positions the Cyt75. An exact requirement for the 5′ sequence to the cleavage site has not been defined () [Citation86].
Hairpin ribozymes
The hairpin ribozymes are called due to their shape resembling a hairpin. They catalyse a reversible cleavage reaction that produces 2′,3′-cyclic phosphate, and 5′-hydroxyl tip, like other self-cutting small ribozymes. First, it was observed that the tobacco ring stain virus was found in the negative strand of the satellite RNA [Citation89]. Although self-cutting small ribozyme motifs can catalyse the same chemical reactions, all these small ribozymes adopt a different structure and use a different catalytic mechanism. The hairpin ribozyme, unlike others, is unique in not requiring direct coordination of metal cations with phosphate or water oxygen for cleavage and ligation reactions. The functional groups in the motif itself carry out the reaction. Despite the importance of this functional group in the hairpin ribozyme was not determined in the first years. The necessity of G8 nucleobase for catalytic activity was found in the following years [Citation90]. In the secondary structure of the hairpin ribozyme, it contains five loops separated by four paired spirals from H1 to H4 [Citation91]. Thus, the shape of the ribozyme can be divided into two domains. Domain 1: besides the spiral 1 and 2, the loop covers 1 and 5, while domain 2: contains the spiral 3 and 4, the loop consists of 2, 3 and 4. The formation of the helical 1 and 2 between the ribozyme and the substrate as the catalytic zone allows the formation of two symmetrical loops directly to each other. The other three loops remain inside the ribozyme due to coils 3 and 4. The entire complex consists of 18 base pairs with non-standard A:G matching [Citation92]. Targeting with hairpin ribozyme requires the BN * GUC gene sequence and match the bases between helical 1 and 2. The base shown as B can be G, U or C, but it should not be A (). In a study, it was determined that the substrate cutting reaction does not occur with A base [Citation91]. Also, hairpin ribozyme has been proven in previous studies to suppress gene regulation in hepatitis C, hepatitis B and human papillomavirus [Citation93].
Twister ribozymes
A new type called twister ribozyme entered to the ribozyme diversity in the last 5 years [Citation94]. The name of ribozyme was given "twister" due to the structure resembling a "twisted flax" in hieroglyphs used in Ancient Egypt [Citation95]. Although twister ribozymes are found in all areas of life, it is quite common in animals. It has a highly preserved secondary structure, and one core contains a circular structure cut by two inner loops. It is approximately the same size as the hammerhead ribozymes but has an orthogonal fold for a similar catalytic speed, unlike the hammerhead ribozyme [Citation95]. Although its biological significance cannot be determined precisely, metal ions are critical for their folding but do not play a direct role in catalysis [Citation96]. presents the secondary structure of a twister ribozyme.
Neurospora VS ribozymes
Collins et al. have found that Neurosporas mitochondrial DNA, a kind of fungus, contains the VS RNA. The RNA transcript has the self-dividing ability and is thought to play a role in the processing of replication intermediates. Rounding in the Neurospora mitochondria leads to a multimeric RNA production that includes a self-disintegrating motif involved in the processing of intermediate products during circle replication [Citation97]. VS ribozymes are the largest of the ribozymes in which they are in the same group as about 150 nucleotides () [Citation98].
GlmS bacterial ribozymes
GlmS ribozyme was discovered as an RNA domain that catalyses region-specific RNA cut in the presence of a small molecule metabolite called glucosamine-6-phosphate (GlcN6P) [Citation99]. The RNA domain is the part of mRNA, encoding the essential enzyme that expresses GlcN6P in gram-positive bacteria [Citation100]. Riboswitch motifs act as a biosensor sensitive to intracellular concentrations of certain metabolites. GlmS ribozyme is a riboswitch motif preserved in a large number of gram-positive bacteria [Citation101]. Also, the GlmS ribozyme is the first self-degrading RNA using an exogenous acid-based catalyst [Citation102]. shows the secondary structure of a trans-cleavage GlmS ribozyme.
Administration of ribozymes
Hammerhead ribozymes catalyse cleavage reactions through the Watson–Crick base pairing upon target mRNA molecules. Thus, gene expression can be down-regulated. Ribozymes can be targeted against dominant mutations due to flexible targeting and arrangement properties on their arms [Citation103]. Mutant protein production causes some genetic diseases. Therefore, gene expression can be down-regulated by ribozymes against the mutant transcript due to specific base-pairing properties of ribozymes to target genes [Citation104]. Although ribozymes interfere gene expression, the RNA trans-splicing process enables them to repair the mutant RNAs. The process demonstrates the genetic information can be revised. The mutant-RNA repair mechanism performed by trans-splicing group I intron ribozymes, and includes the replacement of the wild-type sequence to the wild type designed to be carried in the ribozyme. [Citation105]. Group I intron ribozyme mediated RNA-trans splicing method is an alternative gene therapy for the treatment of genetic disorders with revision without eliminating mutant transcript permanently [Citation106]. Even though strong evidence exists about the method as usable, there are some disadvantages such as the short length of the ribozyme binding region and low sequence specificity. Ribozymes designed to use against viral infections targeting viral RNA molecules are a potential anti-viral agent. For instance, studies have shown the inhibition of viral gene replication and expression in various cell lines by the hammerhead and hairpin ribozymes designed against the human immunodeficiency virus known as HIV [Citation107].
Ribozymes also can be identified as foreign particle by various defence mechanisms and immune system components. Three bacterial ribozymes have been studied for their ability to activate human RNA-activated protein kinase (PKR): Vc2 riboswitch, the GlmS riboswitch-ribozyme and the twister ribozyme. However, RNA modifications such as m6A and 7mG cap have been shown to abolish PKR activation [Citation108].
Exogenous RNA components can affect the original RNAi components within the cell [Citation109]. Some toxic effects have been observed and called RNAi saturation. In a study, siRNAs encapsulated in lipid nanoparticles, did not inhibit endogenous miRNA activity when administered to mice [Citation110]. In another study, ribozyme was added to the 3′ end of siRNA (Ago2-processed interfering RNA) designed as an alternative siRNA precursor, and the modified conjugate increased the activity of both the targeted gene and shRNAs. The addition of ribozyme to the structure, reduced off-target effects and less competition was observed with endogenous miRNAs. This study shows that ribozymes are critical and reliable for preventing RNAi saturation [Citation111].
The off-target effect is the alteration of regions other than the targeted gene and can cause cellular dysfunction. Sequencing and selection of ribozymes are critical for, an undesirable off-target effect which will cause toxicity [Citation112]. Monitoring cellular activities such as selecting ribozymes with acceptable sequences and protein expression in vivo should be performed by bioinformatics [Citation113,Citation114].
Clinical trials and experimental design
RNAi applications against different types of coronaviruses have been proposed by various studies. The RNAi technology has been specifically designed and targeted against SARS and MERS coronaviruses gene regions such as S, M, N, E, RdRp, viral helicase, replicase, ORF1 and ORF3. shows the protected gene regions of SARS-CoV-2, SARS-CoV and MERS-CoV.
Figure 9. Light blue boxes indicate the open reading frame (ORF1a/b) of 5′UTR encoding the non-structural proteins required for viral replication. S box: spike protein; E box: envelope protein; M box: membrane protein; N box: nucleocapsid protein; yellow boxes: other ORFs.
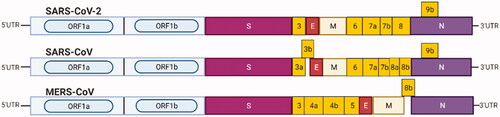
In clinical studies, ribozymes targeting the poliovirus chimera of the hepatitis C virus such as HEPTAZYME, or ribozyme targeting mRNA of vascular endothelial growth factor (VEGF) receptors’ such as ANGIOZYME (anti-Flt-1) have been used [Citation115]. In the mentioned studies, two basic strategies were applied to transfer the target-specific ribozymes into the cell. The first one is the endogenous expression performed by transferring the ribozyme via vectors (viral and non-viral vectors) to the cells (). The second strategy is transferring the previously synthesized ribozymes directly into the cells [Citation116].
Figure 10. “Endogenous Expression": transcription of ribozyme-expressing vectors and synthesis of ribozymes (created with BioRender.com).
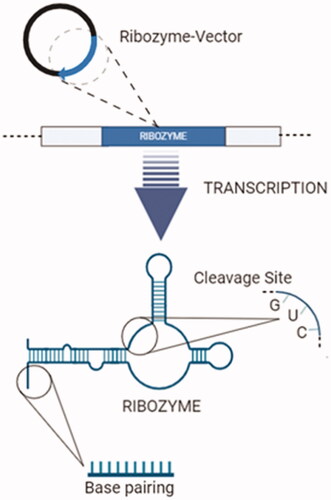
Although ribozyme-vectors are introduced to cells by transduction, non-viral vectors have also been used to obtain more efficient cell uptake ratios. The delivery of a naked ribozyme to a target cell has some limitations, due to its negative charged nature and high molecular weight. Due to their polyanionic nature, ribozymes have difficulty passing through the target cell membrane. The non-viral vector systems as liposomes, solid lipid nanoparticles and polymers have been used to overcome the limitations [Citation117].
There has been considerable interest for the use of non-viral vectors for systemic delivery of ribozymes in gene therapy for cancer. The hammerhead ribozymes targeting different genes were delivered to lung cancer models using the cationic liposome [Citation118]. Moreover, one of the methods for the cellular transfection of ribozymes is the complexation of ribozymes with low-molecular weight polyethylenimine (LMW-PEI). Low molecular weight polyethyleneimines are synthetic polymers frequently used for the delivery of nucleic acids. LMW-PEIs have the ability to compress nucleic acids. It has been emphasized that these polymers are efficient in DNA or RNAi-based gene therapy [Citation119]. This method enabled the ribozymes to be stabilized and to obtain high transfection efficiency.
Transport of non-toxic LMW-PEI-mediated ribozymes resulted in a significant reduction in tumour growth [Citation120]. Besides, nanoparticular systems for the delivery of siRNA, ribozymes and/or aptamers containings were developed [Citation121]. The packaging RNA (pRNA) monomer sequence containing a central 3-way junction (3WJ) domain contains ribozymes, siRNA and folate (FA). The pRNA-3WJ motif has stable properties and this leads to take different RNA molecules to its structure and mediates the folding of RNAs in accordance with the original 3D structure [Citation122].
Vehicles for the transportation of therapeutic agents such as nanoparticles have been developed to achieve an enhanced cellular uptake. The vehicles help to keep therapeutic agents like the ribozyme enzyme, stable in the cytoplasmic pathway [Citation123–125]. The shape, size, charge, morphology and components of a nanocarrier are among the main parameters affecting the toxicity of nanocarriers. In the literature, enhanced toxicities of nanocarriers after surface modifications are available [Citation126]. The immunogenicity of a nanocarrier can be detected by observing hypersensitivity reactions and inflammatory responses [Citation127]. It is well-known that carbon nanotubes genotoxic and toxic effect cause ROS in the microenvironment [Citation128]. In general, nanocarriers can cause inflammatory responses and toxicity in some cases [Citation129]. However, some strategies are applied to reduce the cytotoxicity of the nanocarrier-RNA conjugates. The toxicity of non-viral vectors is arranged by the selection of the biomaterial, optimization of physical properties, and by active targeting strategies [Citation130]. Various targeting molecules can be attached on the vector to deliver RNA–nanoparticle conjugates, to target cells [Citation125]. The proper folding of RNA structure is required to ensure the function of RNA–nanoparticles. Online resources have been developed to overcome this challenge in folding RNA structures. The RNA structure is provided from the web server for these programs. These are the examples of resources that enable the prediction and analysis of RNA structure and folding [Citation131]. Another challenge is to ensure the stability of ribozymes during their journey in vivo. This challenge can be overcome by incorporating stable nucleotides to ribozymes [Citation132,Citation133]. Besides, the endosomal escape of RNA encapsulated nanoparticles poses another problem. Despite this problem, many solution proposals have been developed such as facilitating the separation of components from the endosome by introducing chemical groups such as acetal, hydrazine and maleic amide into cells in a complex form with RNA nanoparticles [Citation134].
Despite these drawbacks associated with nanocarriers, there are currently approximately 35 commercial products based on non-viral vectors (nanoparticles) approved by FDA that have overcome the drawbacks [Citation135].
A nanoparticle system can be used to transfer a ribozyme to a target cell as shown in . The surface modifications of nanoparticles such as attaching a targeting agent to the ACE-2 receptor improves the transfection efficiency.
Figure 11. Transfer of ribozymes to the target cell via nanoparticle, a non-viral transfection tool. (1) Plasmid vectors that will express ribozyme. (2) Pre-synthesized ribozyme. (3) Synthesis of nanoparticles targeted to the ACE-2 receptor. (4) Conjugates formed by nanoparticles with ribozyme-vector and ribozymes. Conjugates are ready for target cell-specific transfection (created with BioRender.com).
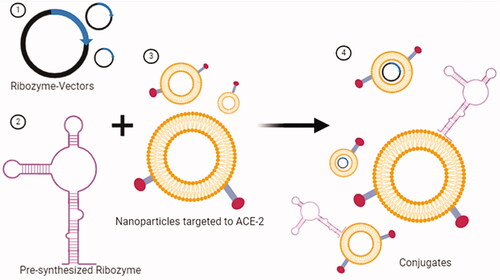
The other effective method against SARS-CoV has been developed using chimeric DNA-RNA hammerhead ribozymes. It was found the ribozymes specifically synthesized against SARS-CoV significantly reduced RNA expression and inhibited viral activity in SARS-CoV infected cells. The results have revealed the method can provide an alternative treatment for SARS [Citation61].
In a study, the ribozyme loaded vector was transferred to isolated cells from asymptomatic HIV-1 seropositive individuals. Transfection was carried out to peripheral blood T lymphocyte or haematopoietic precursor cells. Transfection was aimed to obtain cell strains expressing the target ribozyme. The data obtained from the study showed that in contrast to the control group, modified cells expressing ribozyme survived longer [Citation136]. The clinical trials of using ribozymes against HIV-1 are listed in .
Table 1. Clinical trials and results using anti-HIV-1 OZ1 ribozymes.
In another study, lung fibroblast cell or progenitor lung cell isolation has been performed from SARS-CoV-2 infected asymptomatic individuals. The isolated cells were transfected with a specific ribozyme-vector or pre-synthesized ribozymes targeting SARS-CoV-2 gene regions. The survival and functionality of the cells introduced back to the patient have been monitored. The ribozyme activity has been evaluated against the virus. This study was based on the clinical use of ribozymes created against HIV-1. Besides, the critical point in this situation is SARS-CoV-2 enters host cells through the ACE-2 receptor [Citation137]. The nanocarrier systems can be modified to target ACE-2 receptor by attaching a ligand on the vector system and the ribozymes can be introduced into the cells, by targeting receptors specifically as shown in and . The use of non-viral carriers for transferring ribozymes to the target cell is a critical issue to consider, due to their high selectivity during the transfection process [Citation138]. Serious side effects of ribozymes developed against HIV are not reported yet. Ribozymes are found to be well tolerated in the human body [Citation139].
Figure 12. RNA replication of SARS-CoV-2 and target cleavage mechanism of target-specific ribozymes. (A) SARS-CoV-2 and intracellular replication process. (1) SARS-CoV-2 enters the cell through the endocytic pathway by binding to the ACE2 receptor. (2) The viral polymerase is translated by the ribosomes, following by the viral genome released. (3) Viral RNA is replicated by viral polymerase. (4) Genomic and subgenomic (nucleocapsid, spike, membrane, envelope) RNAs are produced. (B) Binding and entry of targeted ribozyme–nanoparticle conjugates to the target cell. (5) Ribozyme vectors that form the ribozyme–nanoparticle conjugate replicate inside the cell. (C) Cleavage reaction mechanism of ribozymes. (6) Genomic/subgenomic RNAs replicating in the cell contain specific regions to ribozyme binding. (7) These ribozyme-specific regions are cleaved after specific pairing. (8) Target RNAs are fragmented with the cleavage reaction. (9) Viral proteins are not formed due to fragmented transcripts cannot be translated (created with BioRender.com).
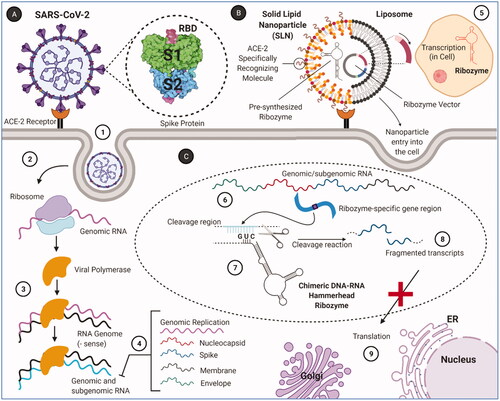
It is possible to design a ribozyme with the selection of ribozymes suitable for different gene regions [Citation140]. Based on the clinical studies and strategies applied, it shows that the basic methods can also be used for SARS-CoV-2. The ribozyme-based treatment approach to SARS-CoV-2 provides a great advantage in comparing to DNA viruses upon the positive RNA structure of the SARS-CoV-2 genome [Citation141]. Ribozymes designed by targeting gene regions in the RNA structure will cleavage gene regions of the virus.
Some key critical points determine the success of candidate ribozymes likely to be developed against SARS-CoV-2 in clinical trials. First, ribozymes have to be transferred to the target lung cells with high cell uptake efficiency. One of the proposed methods is to target the nanoparticles against the ACE-2 receptor as described above. Thus, the transfection efficiency of vectors will be higher compared to a non-targeted vector systems. Non-viral vectors, nanosystems allow us to target a specific receptor on a cell surface. The ligands react with the target cell’s surface specifically, present a high binding affinity to the target cell and protect the nanoparticles cargo from biodegradation. Antibodies, peptides and aptamers are the most widely used ligands to target nanoparticles. The transport of a biological agent via nanoparticle to the target cell increases its uptake efficiency, protects the biological agent from the effects of proteins, enzymes and other components that can cause oxidation, thus ensuring the activity of biological agents [Citation142]. The second critical point is the inhibition level and duration of the target gene according to disease pathophysiology. Ribozyme and vector ratios should be adjusted according to viral gene expression levels [Citation136]. Prepared ribozyme or vectors have a limiting effect in some cases. Ribozymes stimulate the immune system and have some off-target effects. Also, conditions such as the saturation of the native RNAi are the limiting effect of the ribozymes. It is possible to overcome the limiting effect by changing the vector or ribozyme, target gene or by chemical modifications of ribozymes. For example, ribozyme can be combined with a chemotherapeutic agent [Citation143].
Targeting nanoparticles with ribozymes against SARS-CoV-2
There are three main methods of targeting nanoparticles: passive, active and physical targeting [Citation144]. Passive targeting of nanoparticles is usually achieved by optimization of the nanoparticle size [Citation145]. Active targeting systems are the systems that direct the nano-carrier and increase their selectivity in target cells by using ligands on the surfaces of nanoparticles. These ligands can react with the target cells, show high binding affinity to the target cell, and protect the nanoparticles from enzyme damage. The basic approach in active targeting is the use of ligands according to those molecules based on one or more molecules that are overexpressed in the target cell and have specific properties [Citation146]. Although antibodies are the most commonly used ligands, they are difficult to conjugate with nanoparticles due to their short circulation time. However, peptides are among the frequently preferred alternative ligands because of their simpler and smaller structure and easier production. Aptamer ligands that combine the properties of antibodies and peptides can be degraded in a short time [Citation147].
The targeting of nanoparticles with the use of radiation or magnetic fields is carried out within physical targeting systems. Gold nanoparticles with low toxicity are among the physical targeting products widely used in most research, such as photothermal therapy [Citation148].
Although intranasal application of therapeutic agents against viral pulmonary diseases is recommended, targeting nanoparticle-mediated biological agents, and transferring them to the cells is also effective in diversifying treatments. Intranasal application of nanoparticles for the alleviation of viral load, inhibition of viral replication, suppression of cytokine storm caused by a viral infection, and post-disease cell repair are among the recommended studies [Citation149]. Protein and synthetic-based nanoparticles are among the basic and new approaches used in vaccine technology. Besides drug targeting, nanoparticles containing DNA and RNA conjugated nanoparticles or antigen expression/encapsulation can induce antibody production, which forms the basis of vaccine studies [Citation150]. Besides, nanoparticle technology helps to protect the loss of function of the biological agent in the body [Citation142]. These advantages offered by nanoparticle technology can be used to target the pathogenesis of SARS-CoV-2 and the inflammation mechanisms caused by SARS-CoV-2. The main purpose of the presented study is to develop ribozymes specific to the genome of SARS-CoV-2 and introduce them to the target cell with targeted nanoparticles.
Conclusions
In this review, we aimed to discuss the ribozymes against SARS-CoV-2 and suggest that developing ribozymes specific to SARS-CoV-2 and introduce them via targeted nanocarriers as an alternative treatment for COVID-19. First, we suggested that hammerhead ribozymes used against SARS-CoV can also be used against SARS-CoV-2 based on the genome similarity of SARS-CoV and SARS-CoV-2. Second, using bioinformatics applications, the synthesis and characterization of specific ribozymes can be achieved, against RNA gene regions of SARS-CoV-2. Viral vectors are used for the transfection of pre-synthesized ribozymes or ribozyme vectors.
Conventional vaccines expose the immune system to viral proteins or peptides derived from surface proteins. Often highly mutated surface proteins provide viral avoidance. In contrast, ribozymes can target highly conserved regions [Citation151,Citation152]. Here, we suggested the usage of non-viral vectors for the introduction of pre-synthesized ribozymes to a target cell, and we pointed out the benefits of targeting nanoparticles. A targeted transfer of ribozymes to the cell will increase both the specificity and transfection efficiency. The example given here is the delivery of nanoparticle–ribozyme conjugates targeting ACE-2 receptors to cells and specific cleavage of RNA gene regions of ribozymes, SARS-CoV-2. In the presented ribozyme-based treatment method, lipid nanoparticles can be used for encapsulation of RNA products similar to the mRNA vaccine. While the mRNA vaccine provides an immunity, the mentioned ribozyme-based targeting system is a therapy system against the virus. The mRNA vaccines available in the Phase III trials of BioNTech/Pfizer and Moderna require conditions of −80 °C and −20 °C, respectively [Citation32]. Nevertheless, this condition brings some limitations for application such as storage and transportation. However, previous mRNA vaccine studies have shown that vaccines can be stored in an unfrozen environment. Vaccines can keep their function at 4 °C for 10 months, with stabilizing agents [Citation153]. The proposed ribozyme system will be able to store at milder temperatures compared to the storage conditions of mRNA-based vaccines. Besides, the cell uptake and transfection efficiency of the nanoparticles can be increased easily by chemical modifications and optimizations.
The ability of ribozymes to be rapidly designed and delivered by nanocarriers offers a promising strategy in RNA-based pharmaceutical treatments against SARS-CoV-2 in the future applications.
Abbreviations | ||
CLEC2 | = | C-type lectin type II |
COVID-19 | = | coronavirus disease 2019 |
dsRNA | = | double-stranded RNA |
E | = | envelope |
GlcN6P | = | glucosamine-6-phosphate |
HDV | = | hepatitis delta virus |
M | = | membrane |
MERS-CoV | = | Middle-East respiratory syndrome coronavirus |
N | = | nucleoside |
nCoV | = | novel coronavirus |
ORF | = | open reading frame |
RNAi | = | RNA interference |
RNase P | = | ribonuclease P |
S | = | spike |
SARS-CoV-2 | = | severe acute respiratory syndrome coronavirus 2 |
shRNA | = | short-hairpin RNA |
siRNAs | = | small interference RNA |
VEGF | = | vascular endothelial growth factor |
VS | = | Neurospora Varkud satellite ribozymes |
WHO | = | World Health Organisation. |
Disclosure statement
No potential conflict of interest was reported by the author(s).
References
- Lam WK, Zhong NS, Tan WC. Overview on SARS in Asia and the world. Respirology. 2003;8(s1):S2–S5.
- Al Hajjar S, Memish ZA, McIntosh K. Middle East respiratory syndrome coronavirus (MERS-CoV): a perpetual challenge. Ann Saudi Med. 2013;33(5):427–436.
- Shereen MA, Khan S, Kazmi A, et al. COVID-19 infection: origin, transmission, and characteristics of human coronaviruses. J Adv Res. 2020;24:91–98.
- Tang JW, Tambyah PA, Hui DS. Emergence of a new SARS-CoV-2 variant in the UK. J Infect. 2020.
- Bauch CT. Estimating the COVID-19 R number: a bargain with the devil? Lancet Infect Dis. 2020;21(2):151–153.
- Grant MC, Geoghegan L, Arbyn M, et al. The prevalence of symptoms in 24,410 adults infected by the novel coronavirus (SARS-CoV-2; COVID-19): a systematic review and meta-analysis of 148 studies from 9 countries. PLOS One. 2020;15(6):e0234765.
- Turan C, Hacımustafaoğlu M. What is the R0 number and clinical significance in infectious diseases? Cocuk Enfeksiyon Derg. 2020;14(1):e47–e48.
- Li Q, Guan X, Wu P, et al. Early transmission dynamics in Wuhan, China, of novel coronavirus-infected pneumonia. N Engl J Med. 2020;382(13):1199–1207.
- Liu J, Liao X, Qian S, et al. Community transmission of severe acute respiratory syndrome coronavirus 2, Shenzhen, China, 2020. Emerg Infect Dis. 2020;26(6):1320–1323.
- Chan JFW, Yuan S, Kok K-H, et al. A familial cluster of pneumonia associated with the 2019 novel coronavirus indicating person-to-person transmission: a study of a family cluster. Lancet. 2020;395(10223):514–523
- Huang C, Wang Y, Li X, et al. Clinical features of patients infected with 2019 novel coronavirus in Wuhan, China. Lancet. 2020;395(10223): 497–506.
- Oran DP, Topol EJ. Prevalence of asymptomatic SARS-CoV-2 infection: a narrative review. Ann Intern Med. 2020;173(5):362–367.
- Nikolai LA, Meyer CG, Kremsner PG, et al. Asymptomatic SARS coronavirus 2 infection: invisible yet invincible. Int J Infect Dis. 2020;100:112–116.
- Yanes-Lane M, Winters N, Fregonese F, et al. Proportion of asymptomatic infection among COVID-19 positive persons and their transmission potential: a systematic review and meta-analysis. PLOS One. 2020;15(11):e0241536.
- Byambasuren O, Cardona M, Bell K, et al. Estimating the extent of asymptomatic COVID-19 and its potential for community transmission: systematic review and meta-analysis. Off J Assoc Med Microbiol Infect Dis Canada. 2020;5(4): 223–234.
- Mair M, Singhavi H, Pai A, et al. A meta‐analysis of 67 studies with presenting symptoms and laboratory tests of COVID‐19 patients. Laryngoscope. 2020.
- Alimohamadi Y, Sepandi M, Taghdir M, et al. Determine the most common clinical symptoms in COVID-19 patients: a systematic review and meta-analysis. J Prev Med Hyg. 2020;61(3):E304.
- Yesudhas D, Srivastava A, Gromiha MM. COVID-19 outbreak: history, mechanism, transmission, structural studies and therapeutics. Infection. 2020;1-15.
- Razonable RR. Antiviral drugs for viruses other than human immunodeficiency virus. Mayo Clin Proc. 2011;86(10):1009–1026.
- Marascio N, Torti C, Liberto MC, et al. Update on different aspects of HCV variability: focus on NS5B polymerase. BMC Infect Dis. 2014;14(Suppl. 5):S1.
- Afrasiabi S, Pourhajibagher M, Raoofian R, et al. Therapeutic applications of nucleic acid aptamers in microbial infections. J Biomed Sci. 2020;27(1):1–13.
- Wei ZX, Tang TT, Jiang SP. The antiviral mechanisms, effects, safety and adverse effects of chloroquine. Eur Rev Med Pharmacol Sci. 2020;24(12):7164-7172..
- Kayaaslan B, Guner R. Adverse effects of oral antiviral therapy in chronic hepatitis B. World J Hepatol. 2017;9(5):227.
- Soriano V, Labarga P, Barreiro P, et al. Drug interactions with new hepatitis C oral drugs. Expert Opin Drug Metab Toxicol. 2015;11(3):333–341.
- Corrall-Gudino L, Bahamonde A, de las Revillas FA, et al. GLUCOCOVID: a controlled trial of methylprednisolone in adults hospitalized with COVID-19 pneumonia. medRxiv. 2020.
- Gautret P, Lagier J-C, Parola P, et al. Hydroxychloroquine and azithromycin as a treatment of COVID-19: results of an open-label non-randomized clinical trial. Int J Antimicrob Agents. 2020;56(1):105949.
- Thammathiwat T, Tungsanga S, Tiankanon K, et al. A case of successful treatment of severe COVID-19 pneumonia with favipiravir and tocilizumab in post-kidney transplant recipient. Transpl Infect Dis. 2020; e13388.
- Mallmann G, O’Neill HSC. The crystal/melt partitioning of V during mantle melting as a function of oxygen fugacity compared with some other elements (Al, P, Ca, Sc, Ti, Cr, Fe, Ga, Y, Zr and Nb). Journal of Petrology. 2009; 50(9):1765–1794.
- Xu X, Han M, Li T, et al. Effective treatment of severe COVID-19 patients with tocilizumab. Proc Natl Acad Sci U S A. 2020;117(20):10970–10975.
- Li D-D, Li Q-H. SARS-CoV-2: vaccines in the pandemic era. Mil Med Res. 2021;8(1):1–15.
- Mullard A. 2020 FDA drug approvals. Nat Rev Drug Discov. 2021; 20(2):85–90
- Vaccines & Immunizations. Centers for Disease Control and Prevention. 2020. [Online]; [cited 2020 Jan 18]. Available from: www.cdc.gov/ncird/overview/index.html
- Mahase E. Covid-19: what do we know about the late stage vaccine candidates? BMJ. 2020:371:m4576 .
- Cusinato J, Cau Y, Calvani AM, et al. Repurposing drugs for the management of COVID-19. Expert Opin Ther Pat. 2020;10: 1–13.
- Titanji BK, Farley MM, Mehta A, et al. Use of baricitinib in patients with moderate to severe coronavirus disease 2019. Clin Infect Dis. 2020;ciaa879.
- Lamb YN. Remdesivir: first approval. Drugs. 2020;80(13):1355–1359.
- Bajan S, Hutvagner G. RNA-based therapeutics: from antisense oligonucleotides to miRNAs. Cells. 2020;9(1):137.
- Leier A, Bedwell DM, Chen AT, et al. Mutation-directed therapeutics for neurofibromatosis type I. Mol Ther Nucleic Acids. 2020;20:739–753.
- Jain S, Kaur J, Prasad S, et al. Nucleic acid therapeutics: a focus on the development of aptamers. Expert Opin Drug Discov. 2020;1-20.
- Micura R, Höbartner C. Fundamental studies of functional nucleic acids: aptamers, riboswitches, ribozymes and DNAzymes. Chem Soc Rev. 2020;49(20):7331–7353.
- Chen X, Mangala LS, Rodriguez-Aguayo C, et al. RNA interference-based therapy and its delivery systems. Cancer Metastasis Rev. 2018;37(1):107–124.
- Bobbin ML, Rossi JJ. RNA interference (RNAi)-based therapeutics: delivering on the promise? Annu Rev Pharmacol Toxicol. 2016;56:103–122.
- Setten RL, Rossi JJ, Ping Han S. The current state and future directions of RNAi-based therapeutics. Nat Rev Drug Discov. 2019;18(6):421–446.
- Gündoğdu R, Çelik,V. RNA interferans (RNAi). Erciyes Üniversitesi Fen Bilimleri Enstitüsü Fen Bilimleri Dergisi.2009; 25(1): 34-47.
- Deiters A. Small molecule modifiers of the microRNA and RNA interference pathway. AAPS J. 2010;12(1):51–60.
- He F, Yao H, Wang J, et al. Coxsackievirus B3 engineered to contain MicroRNA targets for muscle-specific MicroRNAs displays attenuated cardiotropic virulence in mice. J Virol. 2015;89(2):908–916.
- Mulligan MJ, Lyke KE, Kitchin N, et al. Phase I/II study of COVID-19 RNA vaccine BNT162b1 in adults. Nature. 2020;586(7830):589–593.
- Polack FP, Thomas SJ, Kitchin N, et al. Safety and efficacy of the BNT162b2 mRNA Covid-19 vaccine. N Engl J Med. 2020.
- Liu Z, Wang J, Xu Y, et al. Implications of the virus-encoded miRNA and host miRNA in the pathogenicity of SARS-CoV-2. rXiv preprint arXiv:2004.04874. 2020.
- Ma X, Kim E-J, Kook I, et al. Small interfering RNA-mediated translation repression alters ribosome sensitivity to inhibition by cycloheximide in Chlamydomonas reinhardtii. Plant Cell. 2013;25(3):985–998.
- Ghosh S, Firdous SM, Nath A. siRNA could be a potential therapy for covid-19. EXCLI J. 2020;19:528–531.
- Subramanya S, Kim SS, Manjunath N, et al. RNA interference-based therapeutics for human immunodeficiency virus HIV-1 treatment: synthetic siRNA or vector-based shRNA? Expert Opin Biol Ther. 2010;10(2):201–213.
- Chen W, Feng P, Liu K, et al. Computational identification of small interfering RNA targets in SARS-CoV-2. Virol Sin. 2020;35(3):359–361.
- Zhong Xu J, Lan Zhang J, Guo Zhang W. Antisense RNA: the new favorite in genetic research. J Zhejiang Univ Sci B. 2018;19(10):739–749.
- Pasquier C, Robichon A. SARS-CoV-2 might manipulate against its host the immunity RNAi/Dicer/Ago system. Does mitochondria collapse upon COVID-19 infection? 2020. 1–15.
- Barrey E, Burzio V, Dhorne-Pollet S, et al. Think different with RNA therapy: can antisense oligonucleotides be used to inhibit replication and transcription of SARS-Cov-2? . Preprints. 2020. 1–23.
- Germer K, Leonard M, Zhang X. RNA aptamers and their therapeutic and diagnostic applications. Int J Biochem Mol Biol. 2013;4(1):27–40.
- Ahn D-G, Jeon I-J, Kim JD, et al. RNA aptamer-based sensitive detection of SARS coronavirus nucleocapsid protein. Analyst. 2009;134(9):1896–1901.
- Kruger K, Grabowski PJ, Zaug AJ, et al. Self-splicing RNA: autoexcision and autocyclization of the ribosomal RNA intervening sequence of tetrahymena. Cell. 1982;31(1):147–157.
- Puerta-Fernández E, Romero-López C, Barroso-delJesus A, et al. Ribozymes: recent advances in the development of RNA tools. FEMS Microbiol Rev. 2003;27(1):75–97.
- Fukushima A, Fukuda N, Lai Y, et al. Development of a chimeric DNA–RNA hammerhead ribozyme targeting SARS virus. Intervirology. 2009;52(2):92–99.
- Doudna JA, Cech TR. The chemical repertoire of natural ribozymes. Nature. 2002;418(6894):222–228.
- Tanner MA, Cech TR. An important RNA tertiary interaction of group I and group II introns is implicated in gram-positive RNase P RNAs. RNA. 1995;1(4):349–350.
- Fedor MJ, Williamson JR. The catalytic diversity of RNAs. Nat Rev Mol Cell Biol. 2005;6(5):399–412.
- Doherty EA, Doudna JA. Ribozyme structures and mechanisms. Annu Rev Biophys Biomol Struct. 2001;30(1):457–475.
- Elliott D, Ladomery M. Molecular biology of RNA. Oxford University Press UK. 2017.
- Kazantsev AV, Pace NR. Bacterial RNase P: a new view of an ancient enzyme. Nat Rev Microbiol. 2006;4(10):729–740.
- Evans D, Marquez SM, Pace NR. RNase P: interface of the RNA and protein worlds. Trends Biochem Sci. 2006;31(6):333–341.
- Holzmann J, Frank P, Löffler E, et al. RNase P without RNA: identification and functional reconstitution of the human mitochondrial tRNA processing enzyme. Cell. 2008;135(3):462–474.
- Pannucci JA, Haas ES, Hall TA, et al. RNase P RNAs from some Archaea are catalytically active. Proc Natl Acad Sci USA. 1999;96(14):7803–7808.
- Huang X, Zhao Y, Pu Q, et al. Intracellular selection of trans-cleaving hammerhead ribozymes. Nucleic Acids Res. 2019;47(5):2514–2522.
- Scott WG, Horan LH, Martick M. The hammerhead ribozyme: structure, catalysis, and gene regulation. Vol. 120, 1st ed. Elsevier Inc.; 2013;1-23.
- Lucier JF, Bergeron LJ, Brière FP, et al. RiboSubstrates: a web application addressing the cleavage specificities of ribozymes in designated genomes. BMC Bioinformatics. 2006;7(1):480.
- Zhang Z, Burke JM. Inhibition of viral replication by ribozyme: mutational analysis of the site and mechanism of antiviral activity. J Virol. 2005;79(6):3728–3736.
- Stobart MJ, Simon SLR, Plews M, et al. Efficient knockdown of human prnp mRNA expression levels using hybrid hammerhead ribozymes. J Toxicol Environ Health A. 2009;72(17–18):1034–1039.
- Nazari R, Ma XZ, Joshi S. Inhibition of human immunodeficiency virus-1 entry using vectors expressing a multimeric hammerhead ribozyme targeting the CCR5 mRNA. J Gen Virol. 2008;89(Pt 9):2252–2261.
- Feng Y, Leavitt M, Tritz R, et al. Inhibition of CCR5-dependent HIV-1 infection by hairpin ribozyme gene therapy against CC-chemokine receptor 5. Virology. 2000;276(2):271–278.
- Mitsuyasu RT, Merigan TC, Carr A, et al. Safety and efficacy of autologous CD34+ hematopoietic progenitor cells transduced with an anti-tat ribozyme in a multi-center, randomized, placebo-controlled, phase II gene therapy trial for the human immunodeficiency virus. Nat Med. 2009;15(3):285–292.
- Tang X, Hobom G, Luo D. Ribozyme mediated destruction of influenza A virus in vitro and in vivo. J Med Virol. 1994;42(4):385–395.
- Martick M, Horan LH, Noller HF, et al. A discontinuous hammerhead ribozyme embedded in a mammalian messenger RNA. Nature. 2008;454(7206):899–902.
- De La Peña M, García-Robles I, Cervera A. The hammerhead ribozyme: a long history for a short RNA. Molecules. 2017;22(1):78.
- Hammann C, Luptak A, Perreault J, et al. The ubiquitous hammerhead ribozyme. RNA. 2012;18(5):871–885.
- Amarzguioui M, Prydz H. Hammerhead ribozyme design and application. Cell Mol Life Sci. 1998;54(11):1175–1202.
- Kumar V, Jung YS, Liang PH. Anti-SARS coronavirus agents: a patent review (2008–present). Expert Opin Ther Pat. 2013;23(10):1337–1348.
- Li Y-J, Macnaughton T, Gao L, et al. RNA-templated replication of hepatitis delta virus: genomic and antigenomic RNAs associate with different nuclear bodies. J Virol. 2006;80(13):6478–6486.
- Been MD. HDV ribozymes. Curr Top Microbiol Immunol. 2006;307:47–65.
- Riccitelli N, Luptak A. HDV family of self-cleaving ribozymes. Progress in molecular biology and translational science. 2013;120.:123–171.
- Jimenez RM, Polanco JA, Lupták A. Chemistry and biology of self-cleaving ribozymes. Trends Biochem Sci. 2015;40(11):648–661.
- Fedor MJ. Structure and function of the hairpin ribozyme. J Mol Biol. 2000;297(2):269–291.
- Kuzmin YI, Da Costa CP, Fedor MJ. Role of an active site guanine in hairpin ribozyme catalysis probed by exogenous nucleobase rescue. J Mol Biol. 2004;340(2):233–251.
- Hampel A. The hairpin ribozyme: discovery, two-dimensional model, and development for gene therapy. Progress in nucleic acid research and molecular biology. 1997;58:1–39.
- Shippy R, Lockner R, Farnsworth M, et al. The hairpin ribozyme. Discovery, mechanism, and development for gene therapy. Mol Biotechnol. 1999;12(1):117–129.
- Yu M, Poeschla E, Yamada O, et al. In vitro and in vivo characterization of a second functional hairpin ribozyme against HIV-1. Virology. 1995;206(1):381–386.
- Liu Y, Wilson TJ, McPhee SA, et al. Crystal structure and mechanistic investigation of the twister ribozyme. Nat Chem Biol. 2014;10(9):739–744.
- Eiler D, Wang J, Steitz TA. Structural basis for the fast self-cleavage reaction catalyzed by the twister ribozyme. Proc Natl Acad Sci U S A. 2014;111(36):13028–13033.
- Gaines CS, York DM. Ribozyme catalysis with a twist: active state of the twister ribozyme in solution predicted from molecular simulation. J Am Chem Soc. 2016;138(9):3058–3065.
- Wilson TJ, Li NS, Lu J, et al. Nucleobase-mediated general acid–base catalysis in the Varkud satellite ribozyme. Proc Natl Acad Sci U S A. 2010;107(26):11751–11756.
- Lilley DMJ. The Varkud satellite ribozyme. RNA. 2004;10(2):151–158.
- Ferré-D’Amaré AR, Scott WG. Small self-cleaving ribozymes. Cold Spring Harb Perspect Biol. 2010;2(10):a003574.
- Ferré-D'Amaré F-D. The glmS ribozyme: use of a small molecule coenzyme by a gene-regulatory RNA. Q Rev Biophys. 2010;43(4):423–447.
- Brooks KM, Hampel KJ. Rapid steps in the glmS ribozyme catalytic pathway: cation and ligand requirements. Biochemistry. 2011;50(13):2424–2433.
- Viladoms J, Fedor MJ. The glmS ribozyme cofactor is a general acid–base catalyst. J Am Chem Soc. 2012;134(46):19043–19049.
- O'Rourke SM, Estell W, Scott WG. Minimal hammerhead ribozymes with uncompromised catalytic activity. J Mol Biol. 2015;427(14):2340–2347.
- Serganov A, Patel DJ. Ribozymes, riboswitches and beyond: regulation of gene expression without proteins. Nat Rev Genet. 2007;8(10):776–790.
- Amini ZN, Olson KE, Müller UF. Spliceozymes: ribozymes that remove introns from pre-mRNAs in trans. PLOS One. 2014;9(7):e101932.
- Phylactou LA, Kilpatrick MW, Wood MJA. Ribozymes as therapeutic tools for genetic disease. Hum Mol Genet. 1998;7(10):1649–1653.
- Del Corpo O, Goguen RP, Malard CMG, et al. A U1i RNA that enhances HIV-1 RNA splicing with an elongated recognition domain is an optimal candidate for combination HIV-1 gene therapy. Mol Ther Nucleic Acids. 2019;18:815–830.
- Hull CM, Anmangandla A, Bevilacqua PC. Bacterial riboswitches and ribozymes potently activate the human innate immune sensor PKR. ACS Chem Biol. 2016;11(4):1118–1127.
- Grimm D, Wang L, Lee JS, et al. Argonaute proteins are key determinants of RNAi efficacy, toxicity, and persistence in the adult mouse liver. J Clin Invest. 2010;120(9):3106–3119.
- Akinc A, Zumbuehl A, Goldberg M, et al. A combinatorial library of lipid-like materials for delivery of RNAi therapeutics. Nat Biotechnol. 2008;26(5):561–569.
- Shang R, Zhang F, Xu B, et al. Ribozyme-enhanced single-stranded Ago2-processed interfering RNA triggers efficient gene silencing with fewer off-target effects. Nat Commun. 2015;6(1):1–13.
- Fedorov Y. Off-target effects by siRNA can induce toxic phenotype. RNA. 2006;12(7):1188–1196.
- Weinberg CE, Weinberg Z, Hammann C. Novel ribozymes: discovery, catalytic mechanisms, and the quest to understand biological function. Nucleic Acids Res. 2019;47(18):9480–9494.
- Roth A, Weinberg Z, Chen AGY, et al. A widespread self-cleaving ribozyme class is revealed by bioinformatics. Nat Chem Biol. 2014;10(1):56–60.
- Usman N, Blatt LM. Nuclease-resistant synthetic ribozymes: developing a new class of therapeutics. J Clin Invest. 2000;106(10):1197–1202.
- Schubert S, Kurreck J. Ribozyme- and deoxyribozyme-strategies for medical applications. Curr Drug Targets. 2004;5(8):667–681.
- Goyal S, Gupta N, Chandra R. Nanoparticles for ribozymes delivery. Advances in nanomedicine for the delivery of therapeutic nucleic acids. 2017;135–150.
- Kashani-Sabet M. Non-viral delivery of ribozymes for cancer gene therapy. Expert Opin Biol Ther. 2004;4(11):1749–1755.
- Höbel S, Prinz R, Malek A, et al. Polyethylenimine PEI F25-LMW allows the long-term storage of frozen complexes as fully active reagents in siRNA-mediated gene targeting and DNA delivery. Eur J Pharm Biopharm. 2008;70(1):29–41.
- Aigner A, Fischer D, Merdan T, et al. Delivery of unmodified bioactive ribozymes by an RNA stabilizing polyethylenimine LMW PEI efficiently down regulates gene expression. Gene Ther. 2002;9(24):1700–1707.
- Jasinski DL, Binzel DW, Guo P. One-pot production of RNA nanoparticles via automated processing and self assembly. ACS Nano. 2019;13(4):4603–4612.
- Shu D, Khisamutdinov EF, Zhang L, et al. Programmable folding of fusion RNA in vivo and in vitro driven by pRNA 3WJ motif of phi29 DNA packaging motor. Nucleic Acids Res. 2014;42(2):e10.
- Rouge JL, Sita TL, Hao L, et al. Ribozyme-spherical nucleic acids. J Am Chem Soc. 2015;137(33):10528–10531.
- Shu D, Shu Y, Haque F, et al. Thermodynamically stable RNA three-way junction for constructing multifunctional nanoparticles for delivery of therapeutics. Nat Nanotechnol. 2011;6(10):658–667.
- Shu Y, Pi F, Sharma A, et al. Stable RNA nanoparticles as potential new generation drugs for cancer therapy. Adv Drug Deliv Rev. 2014;66:74–89.
- Rybak-Smith MJ. Effect of surface modification on toxicity of nanoparticles. Encyclopedia of nanotechnology. 2012;645–652.
- Alsaleh NB, Brown JM. Engineered nanomaterials and type I allergic hypersensitivity reactions. Front Immunol. 2020;11: 222.
- Bourdon JA, Saber AT, Jacobsen NR, et al. Carbon black nanoparticle instillation induces sustained inflammation and genotoxicity in mouse lung and liver. Part Fibre Toxicol. 2012;9(1):5.
- Ajdary M, Moosavi M, Rahmati M, et al. Health concerns of various nanoparticles: a review of their in vitro and in vivo toxicity. Nanomaterials. 2018;8(9):634.
- Xue HY, Liu S, Wong HL. Nanotoxicity: a key obstacle to clinical translation of siRNA-based nanomedicine. Nanomedicine. 2014;9(2):295–312.
- Bellaousov S, Reuter JS, Seetin MG, et al. RNAstructure: web servers for RNA secondary structure prediction and analysis. Nucleic Acids Res. 2013;41(Web Server issue):W471–W474.
- Cui D, Zhang C, Liu B, et al. Regression of gastric cancer by systemic injection of RNA nanoparticles carrying both ligand and siRNA. Sci Rep. 2015;5(1):10726.
- Khisamutdinov EF, Li H, Jasinski DL, et al. Enhancing immunomodulation on innate immunity by shape transition among RNA triangle, square and pentagon nanovehicles. Nucleic Acids Res. 2014;42(15):9996–10004.
- Kwon YJ. Before and after endosomal escape: roles of stimuli-converting siRNA/polymer interactions in determining gene silencing efficiency. Acc Chem Res. 2012;45(7):1077–1088.
- Saha AK, Zhen M-YS, Erogbogbo F, et al. Design considerations and assays for hemocompatibility of FDA-approved nanoparticles. Semin Thromb Hemost. 2020;46(5):637–652.
- Sullenger BA, Gilboa E. Emerging clinical applications of RNA. Nature. 2002;418(6894):252–258.
- Zhang H, Penninger JM, Li Y, et al. Angiotensin-converting enzyme 2 (ACE2) as a SARS-CoV-2 receptor: molecular mechanisms and potential therapeutic target. Intensive Care Med. 2020;46(4):586–590.
- Shu Y, Shu D, Diao Z, et al. Fabrication of polyvalent therapeutic RNA nanoparticles for specific delivery of siRNA, ribozyme and drugs to targeted cells for cancer therapy. IEEE NIH Life Sci Syst Appl Workshop. 2009;2009:9–12.
- Zeller SJ, Kumar P. RNA-based gene therapy for the treatment and prevention of HIV: from bench to bedside. Yale J Biol Med. 2011;84(3):301–309.
- Kharma N, Varin L, Abu-Baker A, et al. Automated design of hammerhead ribozymes and validation by targeting the PABPN1 gene transcript. Nucleic Acids Res. 2016;44(4):e39.
- Hasöksüz M, Kiliç S, Saraç F. Coronaviruses and SARS-CoV-2. Turk J Med Sci. 2020;50(SI-1):549–556.
- Patra JK, Das G, Fraceto LF, et al. Nano based drug delivery systems: recent developments and future prospects. J Nanobiotechnol. 2018;16(1):71.
- Mistry SJ, Atweh GF. Therapeutic interactions between stathmin inhibition and chemotherapeutic agents in prostate cancer. Mol Cancer Ther. 2006;5(12):3248–3257.
- Yu X, Trase I, Ren M, et al. Design of nanoparticle-based carriers for targeted drug delivery. J Nanomater. 2016;2016:1–15.
- Bazak R, Houri M, El Achy S, et al. Passive targeting of nanoparticles to cancer: a comprehensive review of the literature. Mol Clin Oncol. 2014;2(6):904–908.
- Attia MF, Anton N, Wallyn J, et al. An overview of active and passive targeting strategies to improve the nanocarriers efficiency to tumour sites. J Pharm Pharmacol. 2019;71(8):1185–1198.
- Ling D, Hackett MJ, Hyeon T. Surface ligands in synthesis, modification, assembly and biomedical applications of nanoparticles. Nano Today. 2014;9(4):457–477.
- Vines JB, Yoon JH, Ryu NE, et al. Gold nanoparticles for photothermal cancer therapy. Front Chem. 2019;7:167.
- Itani R, Tobaiqy M, Faraj AA. Optimizing use of theranostic nanoparticles as a life-saving strategy for treating COVID-19 patients. Theranostics. 2020;10(13):5932–5942.
- Shin MD, Shukla S, Chung YH, et al. COVID-19 vaccine development and a potential nanomaterial path forward. Nat Nanotechnol. 2020;15(8):646–655.
- Graham BS, Gilman MSA, McLellan JS. Structure-based vaccine antigen design. Annu Rev Med. 2019;70(1):91–104.
- Nelemans T, Kikkert M. Viral innate immune evasion and the pathogenesis of emerging RNA virus infections. Viruses. 2019;11(10):961.
- Jones KL, Drane D, Gowans EJ. Long-term storage of DNA-free RNA for use in vaccine studies. Biotechniques. 2007;43(5):675–681.