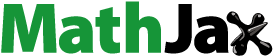
Abstract
Many of the bioactive substances used in pharmaceutical industry are easily affected by temperature, light and oxygen, and are easily degraded during storage and processing, and exhibit poor adsorption properties during digestion, which limits their direct use. Microalgae are rich in oils which have antimicrobial properties and antioxidants that attract attention in both food and pharmaceutical sectors in recent years. Studies to encapsulate bioactive compound-rich microalgae oils with nanotechnological approaches to improve the physical and chemical stability are relatively new, and it is promising to apply these approaches for pharmaceutical purposes. In this study, cytotoxic effects of oil extracts of Botryococcus braunii and Microcystis aeruginosa and their oil-loaded nanoparticles on L929 cell line, PC-3 prostate cell line, SHSY-5Y neuroblastoma cell line and AGS gastric adenocarcinoma cell line were investigated. The obtained extracts were found to have no cytotoxic effect on L929 cells. However, they showed cytotoxic effect on cancer cells. As for the nanoparticles; a gradual release was determined and the stability of the nanoparticle structure was shown. In the light of obtained findings, it was considered that nanoparticles produced with oil extracts of microalgae which have bioactive substances, have potential to be evaluated especially in pharmaceutical and cosmetic fields.
Introduction
The focus of nanotechnology applications in the food and pharmaceutical fields is to improve the release system of bioactive components in the nano structure, and to develop an encapsulation system that not only improves the stability of the product, but also protects the bioactives affected by environmental conditions from unwanted interactions and simultaneously releases the product into the target area without reducing the quality of the product. For this purpose, novel methods are needed to improve product quality, shelf life and nutritional value of functional substances rich in bioactives. However, the fact that the consumption habits are on the materials obtained from natural raw materials reveal the necessity of obtaining the substance that will provide the transport of bioactive substances from a natural source [Citation1].
Nanoparticles have small particle size and increased surface area, which can be manipulated to achieve active and passive targeting. The decreased particle size contributes to their unique property being able to cross the blood–brain barrier and cell and tissue barriers. Nanoparticles bind to receptors by endocytosis, which leads to decreased reorganization by P-glycoprotein [Citation2]. For years, many nanoparticle-based delivery systems such as liposome, polymeric micelles and nano lipid carriers have been developed [Citation3,Citation4]. There are several advantages of nanoparticle-based delivery systems such as controlled drug release, encapsulation of various drugs and providing extend blood circulation time and utilization of the enhanced permeability and retention effect for enhancing treatment [Citation5]. Especially, polymer-based nanoparticles have come into prominence for clinical and biological applications because of their low toxicity, low immunogenicity and biocompatibility [Citation6].
In the literature, different drugs and bioactive compounds like doxorubicin, crocin and curcumin are widely investigated to be utilized against cancer cells. Studies show that, type of drug/bioactive compound, concentration of drug/bioactive compound, type of cancer cell line and exposure time of cytotoxicity analysis cause different results on evaluating an anticancer effect. Doxorubicin is one of the most investigated drugs for cancer cells in both free and nanoencapsulated form. Although it has high efficiency, doxorubicin has significant side effects including hair loss, bone marrow suppression, vomiting, rash, and inflammation of the mouth. Therefore, there is a need for substitution of drugs like doxorubicin to fight against cancer cells without damaging normal cells. Instead of using chemical compounds, bioactive compounds which occur in nature and consist of essential and/or nonessential compounds show promising results by reducing the viability of the cancer cells without having side effect [Citation7]. As a bioactive compound source, algae have been widely investigated since 1994 for anticancer studies. In the literature, there are many studies on exploring the effect of algal extracts on different cancer cell lines such as human colon, breast, prostate and oral. Efficiency of compounds produced by algae on different cancer lines has been proven with scientific studies. Algae serve as reservoirs of many bioactive metabolites, including valuable fatty acids and carotenoids. These bioactives have a wide range of bioactivity, including antioxidant, anti-inflammatory, anti-viral and anti-cancer effects [Citation8]. In the literature, the most investigated algal species for anticancer studies are Chlorella sp., Spirulina sp., D. salina and H. pluvialis. However, it is known that, algae have been estimated to contain from 30,000 to more than 1 million species all around the world which suggests that there may be tens of thousands of species that need to be studied for anticancer studies and have great potential [Citation9]. Besides, algae have many advantages that surpass other natural sources. For instance, although ω-3 fatty acids are obtained from fish oils, problems have increased and become less preferable in recent years due to unpleasant taste and poor oxidative stability. Compared to fish, microalgae produce the ω-3 as well as make the process simple and economical [Citation10]. Today, encapsulation techniques are used to protect bioactives from oxidation and prevent unwanted taste formation in food and pharmaceutical applications.
Although there are studies on effect of algal extracts on cancer cells, there is not any research on the effect of B. braunii and M. aeruginosa extracts against cancer cells. The cytotoxic effects of microalgal extracts on PC-3 prostate cell line, SHSY-5Y neuroblastoma cell line and AGS gastric adenocarcinoma cell line were compared. Due to the proven effects of algal metabolites, nanoparticles loaded with extracts obtained from microalgae were produced, characterized and tested for evaluating their anticancer activity. Although, there is a few study on encapsulation of algal extracts in the literature, there is not any study on nanoparticle production loaded with B. braunii and M. aeruginosa extracts and anticancer effect of these nanoparticles.
Materials and methods
Materials
All the chemicals used in the experiments were supplied from Merck, Germany. C. protothecoides oils were commercially supplied and B. braunii and M. aeruginosa species were obtained from their cultures in the Algal Biotechnology and Bioprocess Laboratory, Department of Bioengineering, Yildiz Technical University. In the cell experiments, L929 mouse fibroblast, PC-3 prostate cancer cell line, SHSY-5Y neuroblastoma (brain) cell line and AGS gastric adenocarcinoma (stomach) cell line were obtained from Cell Culture Laboratory, Department of Bioengineering, Yildiz Technical University.
Production of microalgal oil extracts
B. braunii and M. aeruginosa were cultivated in modified BG-11 medium in a 20 L photobioreactor, and cultivation was carried out at the temperature of 27 °C and pH 8.3. A pump was used for aeration of the culture medium. Photobioreactor was exposed to continuous illumination. Cultivated microalgae were centrifuged at 8000 rpm for 20 min and dried in an oven at the temperature of 60 °C for 24 h. Dried microalgae samples were crushed into powder and extracted using ethanol as solvent. The extract was distilled by evaporation at 78 °C under vacuum in the evaporator to remove ethanol. The oil extract was stored in a closed and dark environment in case of any oxidation.
Characterization of microalgal oil extracts
Gas chromatography was used to determine the content of microalgae oil. Analyzes were carried out on YL Instruments 6100 GC gas chromatography. The gas chromatography contains a flame ionization detector and a 30 m × 0.32 mm × 0.25 µm ZB-FFAP column. The column temperature program starts at 50 °C and reaches to 175 °C at 15 °C/min, then temperature increases to 230 °C at 5 °C/min. The injector temperature was 230 °C and the flow rate was set at 1.8 ml/min. The detector temperature was kept at 280 °C. Hydrogen gas was used as the carrier gas. Methyl heptadeconate (C17: 0) was used as internal standard and the samples were made ready for gas chromatography by mixing with methyl heptadeconate and n-heptane.
The total antioxidant activity of the algal extracts was determined using the 1,1-diphenyl-2-picryl hydrazil (DPPH) free radical scavenging method [Citation11]. The decrease in absorbance in the reaction mixture is indicative of high free radical removal activity.
The DPPH radical scavenging activity was calculated using the following formula:
(1)
(1)
A0 is the absorbance value of the control; A1 is the absorbance value of sample or standard.
Assessment of cytotoxicity
The 2,3-Bis-(2-methoxy-4-nitro-5-sulfophenyl)-2H-tetrazolium-5-carboxanilide (XTT) method which is based on the principle that metabolically active cells reduce XTT, a tetrazolium salt, to orange formazan components, is selected for the cytotoxicity assessment of the samples. The increased absorbance value as a result of colour formation is proportional to the number of metabolically active cells. One of the most important advantages of the XTT method is that there is no need for additional agents or cell washing procedures and that the method has the sensitivity even at low cell concentrations [Citation12].
In this study, 24-h cytotoxic effect was evaluated by applying different concentrations of the extracts of three species (in the range of 3.5–2500 µg/ml) to different cell lines. L929 (mouse fibroblast cells), PC-3 (human prostate cancer cell), SH-SY5Y (neuroblastoma cell) and AGS (human gastric cancer cell) lines were used in cytotoxicity experiments. Cells stored in liquid nitrogen were thawed at 37 °C, transferred to a tube containing 5 ml of DMEM-F12 medium (supplemented with 10% FBS, 5 µg/ml penicillin–streptomycin) and centrifuged at 1000 rpm for 5 min. The cells in the pellet were counted by trypan blue staining, the medium was then suspended in medium and transferred to sterile 25 T cell culture flasks. Cells transferred to flasks were incubated at 37 °C in a 5% carbon dioxide incubator. Passaging was performed when the cells were 80–90% confluent. Briefly, trypsin-EDTA-added cells were allowed to stand in the incubator for 5 min and when detached cells were microscopically determined cells were suspended in the medium. After centrifugation, trypsin-EDTA was removed, counting was performed in the cell pellet and cells were prepared for use in the cytotoxicity test.
Flat bottom sterile 96-well plate was seeded with 104 cells/well. The cells were incubated for 24 h to adhere to the surface and proliferate, and after microscopic examination, the oils at different concentrations were added to cells for cytotoxic activity (n = 3).
Algae extracts were sterilized by filtration through a 0.45 µm filter before adding to the cells and dilutions were prepared in DMEM-F12 medium. The cells exposed the different concentrations of oils were incubated for 24 h at 37 °C in a 5% carbon dioxide incubator and then the XTT test was performed. Oil-containing media was aspirated from wells and 100 µL of 0.5 mg/mL XTT solution (with 7.5 µg/mL phenazine) was added to the wells. The plates were incubated for a further 4 h at 37 °C and the optical density was evaluated by measuring the absorbance at 450 nm with the multi-plate reader.
Preparation of microalgal oil extract loaded nanoparticles
To produce nanoparticle, 8% (w/v %) PVA solution and 2% (w/v) sodium alginate solution stocks were prepared. After the materials dissolved completely, they were mixed by taking 80:20 (v/v %) of the two solutions by volume. Subsequently, 1% (w/v) algae oil and 1% (w/v) Tween 20 were added and mixed for a further 15 min to obtain a completely homogeneous solution, which was then fed into the syringe in electrospraying equipment (Laboratory scale Holmarc Opto Mechatronics Pvt. Ltd. Nano Fibre Electrospinning Unit). In the experiment, first, the collector plate was covered with aluminium foil and the negative electrode was attached to the plate. The polymer solution-essential oil mixture filled into the injector was placed on the pump portion and the other end of the electrode was attached to the metal syringe tip. In this way, an electrical gravitational field is formed with opposite charged electrodes. Pump rate was adjusted to 0.2 ml/h and distance between syringe and collector plate was 13 cm.
Characterization of nanoparticles
The particle size of the microalgal extract-loaded nanoparticles were measured by using Malvern Zetasizer Nano ZS instrument.
To determine whether the encapsulation process was performed, Thermoscientific Brand Nicolet 6700 model FTIR was used and the absorbance values of the functional groups of extracted oils and nanoparticles in the range of 600–4000 cm−1 were determined.
Scanning electron microscope (SEM) measurements of the nanoparticles obtained by the electrospraying method were taken with Zeiss EVO LS 10 SEM in YTU Central Laboratory at 20–50.00k × magnification.
Thermogravimetric analysis of raw materials was performed using a thermogravimetric analyzer (TA Instruments, New Castle, DE, SDT Q600). Analysis was carried out up to temperature of 600 °C with a heating rate of 10 °C/min and nitrogen flow rate of 40 ml/min.
The release profile was analyzed with Sudan Red G dye. Release medium was prepared with Tween 20 (wt/vol) in double-distilled water. About 5 ml release medium and 25 mg of particles were stirred at 100 rpm in a flask at 25 °C. At selected time intervals, the discharge medium was collected and centrifuged at a speed of 5000 rpm for 15 min. The concentration of dye within the supernatant was determined by using the UV spectrophotometer at 501 nm.
Statistical analysis
Experiments carried out in triplicate. The results obtained from each experiment were given as mean ± standard error in figures.
Results and discussion
Characterization of microalgal oil extracts
When the chromatograms were examined, the presence of palmitic, oleic, linoleic, arachidic and eicosapentaenoic acids were determined in the content of B. braunii oil. Other fatty acids were present in trace amounts. According to the obtained chromatogram, the highest fatty acid content was belong to linoleic acid. B. braunii was found to be rich in polyunsaturated fatty acids with bioactive value. In the case of M. aeruginosa, palmitic acid, oleic acid and linoleic acid were determined. The highest fatty acid content was belong to palmitic acid. According to these analyses, B. braunii and M. aeruginosa were found to have bioactive value and were rich in useful polyunsaturated fatty acids. B. braunii can produce chemicals that have significant potential as feedstocks for biofuels, pharmaceuticals and nutraceuticals [Citation13,Citation14]. As for M. aeruginosa, it is investigated for the production of antioxidant, food additive and industrial chemical. Similar fatty acid profile of B. braunii and M. aeruginosa were reported in the literature [Citation15,Citation16].
The total antioxidant activity of the algal extracts was determined using the 1,1-diphenyl-2-picryl hydrazil (DPPH) free radical scavenging method. When the obtained results were examined, it was seen that microalgal extracts had antioxidant activities. Measurements for standard and microalgae extracts were taken after 30 min, 1 h and 1.5 h. Free radical scavenging activity of microalgae extracts was determined and activity comparisons were made according to BHT used as standard. The BHT standard was prepared as 500 and 1000 μg/ml. As a result of the experiments, it was observed that the extracts of B. braunii and M. aeruginosa species have antioxidant activities as shown in . Among these species, M. aeruginosa had higher antioxidant activities. This was expected due to their lipids and phenolics. Li et al. also reported that, M. aeruginosa had DPPH activity between 50% and 70% in different culture media [Citation17]. In the study of Banskota et al., DPPH radical scavenging activity of nine different microalgae species were investigated. It was found that T. chuii and B. braunii showed high activity surpassing Chlorella sp. and Nannochloropsis sp. [Citation18].
Assessment of cytotoxicity of microalgal oil extracts
The cytotoxic effect of different algal extracts was examined on L929, PC-3, SHSY-5Y and AGS cell lines. The cytotoxic effect of the extracts and commercially available C. protothecoides oil on the L929 cell line is presented in . As shown in . braunii (BB) and M. aeruginosa (MA) showed no toxicity on L929 cell lines. There is not any study in the literature which investigates the cytotoxic effect of extracts of B. braunii and M. aeruginosa species on cell culture. Also, when the studies on different algal species in the literature are examined, it is seen that algal extracts do not have toxic effects on L929 cells. For instance, Chu et al. showed that Spirulina extracts do not cause cytotoxic effect on mouse fibroblast cells (3T3). It was reported that, Spirulina extract has a protective effect against apoptotic cell death due to free radicals [Citation19]. In another study, cytotoxic effect of extracts from Eisenia bicyclis algae was tested on the RBL-2H3 rat mast cell line, HaCaT human keratinocyte cell line and NIH3T3 mouse fibroblast cell line. As a result of the study, it was observed that the extracts had no toxic effect on HaCaT and NIH3T3 cells and it did not create toxic effect on RBL-2H3 cells to a certain concentration [Citation20]. Yan et al. investigated the production of cellulose/microalgae films, and it was reported that, L929 cells were able to adhere on the films which were created by using Nannochloropsis and cellulose, and the cells cultured on the composite films proliferated better than those on the pure cellulose film [Citation21]. Senousy et al. assessed the cytotoxic effect of 12 cyanobacteria species and 2 microalgae species against human peripheral blood mononuclear (PBMC) cells. It was reported that Oscillatoria sancta HSSASE19 was determined as the safest recording IC50 value of 991.1 ± 0.5 μg/ml. On the other hand, Chlorella sorokiniana HSSASE17 and Dolichospermum flos-aquae HSSASE2 showed cytotoxicity with IC50 values of 498.9 ± 0.3 and 337 ± 0.9 μg/ml. These results represented that algal extracts may show cytotoxic effect on healthy cells under very high concentrations [Citation22].
Cytotoxic effect of microalgal extracts on PC-3, SHSY-5Y and AGS cancer cell lines are given in . Also, the qualitative data of the effect of microalgal extracts are given in . The cytotoxic effect of B. braunii and M. aeruginosa extracts was found to be significantly greater than the effect of commercially available C. protothecoides oil on cancer cells. From these results, it was seen that commercial microalgal oil which previously supplied, lost its bioactive characteristic. On the other hand, newly extracted microalgal extracts were highly effective on cancer cells. Cytotoxic effects of microalgal extracts on cancer cells were partially high compared to C. protothecoides oil. According to the XTT results, M. aeruginosa extracts had higher effect than the extracts of B. braunii. When cytotoxic effects on cancer cell lines were compared, it was determined that microalgal extracts affected SHSY-5Y, then PC-3, and AGS cell lines, respectively. In concentrations where it did not show toxic effects in L929 cells, the decrease in the viability of cancer cells showed the anticancer potential of BB and MA extracts. The anticancer effect may be due to activation of the apoptotic pathway. These findings were consistent with research showing that activation of the pro-apoptotic proteins is the primary cause of the cytotoxicity reported by researchers who showed that algal extracts have cytotoxic effects against various cancer types [Citation23–26]. Czerwonka et al. reported that Spirulina extract induced apoptosis in the human lung cancer A549 cells. Besides, it was stated that higher concentrations of Spirulina extract disrupted cancer cell membrane integrity at concentrations above 250 μg/ml, leading to necrotic cell death [Citation27]. Reyna-Martinez et al. also reported that 60–70% tumour cytotoxicity was observed by C. sorokiniana and Scenedesmus sp. methanol extracts, respectively, at 500 µg/ml, by the mechanism of apoptosis. It was indicated that, at low doses, a variety of injurious stimuli such as heat, radiation, hypoxia, and cytotoxic anticancer drugs can induce apoptosis, or lead to necrosis at higher doses [Citation28].
Characterization of microalgal oil extract loaded nanoparticles
The average particle size of microalgal oil extract loaded nanoparticles was determined by using a Zeta Sizer, and size distribution graph is given in . The particle sizes of extracts of B. braunii (BBN) and M. aeruginosa (MAN) were found as 197.7 ± 20 nm and 90.34 ± 10 nm, respectively. PDI for the samples of BBN and MAN were determined in the range of 0.32–0.44. shows the SEM images of the BBN and MAN samples. As a result of SEM analysis, it was seen that the produced particles were spherical, and 100–600 nm particles were detected with the analysis. The reason of the difference in the size of the nanoparticles might be the sudden changes in the voltage and current. In addition to that, since the particles are tend to agglomerate, bigger sized particles may have been formed. It was reported that solvent used in the process may not evaporate completely when it reaches the collector. This causes agglomeration of wet nanoparticles and formation of larger particles [Citation29,Citation30]. Nourmohammadi et al. investigated encapsulation of Spirulina to be used as an additive in yogurt. In this study, Spirulina was encapsulated using alginate and whey protein concentrate (WPC) by the emulsification method. It was found that, the particle size of microcapsules was found as 51.73 ± 37.69 μm [Citation31]. In the literature, there is not any study on B. braunii and M. aeruginosa oil extracts loaded nanoparticle production by the electrospraying method. Only, there is one study on electrospraying of commercial C. protohecoides oil-loaded nanoparticles. For this reason, data obtained from this study were compared with the study carried out by Karakaş et al. In that study, alginate and chitosan nanoparticles loaded with microalgal extracts were produced via electrospraying and microemulsion methods. It was reported that particle sizes were changed between 123.9 and 762.4 nm because of performing different methods and materials. It was observed that the smallest nanoparticles were obtained with electrospraying method [Citation32,Citation33]. Our findings in our study were found similar with this study.
As can be seen with FTIR spectrum in , bands of hydroxyl, ether and carboxylic functional groups were observed. At 3283 cm−1, the –OH tensile band was formed and the bands seen at 2922–2853 cm−1 had CH antisymmetric and symmetrical stresses. Peaks at 1743 and 1159 cm−1 indicated the presence of oil in the samples. At 1021 and 831 cm−1 there was C–O stress and CH plane deformation. The peak at 1462 cm−1 appeared as asymmetric and symmetrical vibrational stresses of carboxylate salt ions. As can be seen in the spectrum, the peaks of the microalgal oil-loaded nanoparticles and microalgal oil coincided at 2922, 2853, 1743, and 1462 cm−1. This overlap showed the presence of oil in the produced nanoparticles. In the literature, overlaps in the peaks were observed in the various studies that used different oils and polymers for encapsulation researches. Moghaddam et al. studied the production of calcium alginate nanocapsules containing n-nonadecane. When the FTIR spectrum of produced nano capsules and oil was examined, overlaps were observed at 2920 and 2850 cm−1, which was similar to the peaks in this study, and showed that n-nonadecane was successfully encapsulated into the capsules [Citation34]. In another study, the presence of silicone oil in polycaprolactone capsules was observed by FTIR analysis [Citation35].
As for the thermal degradation of the nanoparticles, degradation began with a moisture output of about 100 °C. The moisture contents of the samples were between 5.54 and 4.25%. At the temperature above 225 °C, the microalgal oil extract-loaded nanoparticles started to decompose slowly. Residues between 8 and 9% were found for both oil-free particles and oil-loaded nanoparticle. The nanoparticles degraded in two steps after the moisture output. First, a weight loss of 61–62% in the temperature range of 225–275 °C, and a weight loss of 18–23% in the temperature range of 275–600 °C occurred in the second step.
In the release test with Sudan Red G dye, it was seen that algal extract in the nanoparticle was started to release in the first 24 h. In the first 24 h, the release of algae oil in the nanoparticles occured in a linear decrease. In this duration, there was a controlled release and the absorbance of the samples started to decrease from 1.2 to 0.8 during this release process. It can be reported that controlled release of the material could be achieved with this stabilized release level and duration.
Assessment of cytotoxicity of the nanoparticles
The cytotoxic effect of the microalgal oil extract loaded nanoparticles on the L929 cell line is presented in . Since the commercial C. protothecoides oil did not have any remarkable effect on cancer cell lines, comparisons were carried out only for nanoparticles produced with the oil extracts of B. braunii and M. aeruginosa. When the effect of nanoparticles loaded with B. braunii and M. aeruginosa oil extracts on L929 cell line was examined, it was seen that the nanoparticles did not cause cytotoxic effect on L929 cells after 24 h. In the literature, studies on encapsulation of microalgal extracts are generally carried out to be utilized in food applications and only a few of them is for pharmaceutical application. For this reason, cytotoxicity analyses against cancer cells were not performed except for three studies. In one of these studies, anticancer activities of C. protothecoides oil-loaded nanoparticles were investigated against HUVEC cell line. It was reported that particles showed no cytotoxic effect in this cell line. On the other hand, particles affected A-172 and HCT-116 cancer cell lines [Citation32]. In another study, polypeptide from Chlorella pyrenoidosa was encapsulated using alginate and chitosan via complex coacervation and ionotropic gelation method. In this study, particles were tested only against human liver cancer HepG2 cells. It was presented that the inhibition rates of unencapsulated, microencapsulated, and nanoencapsulated product on HepG2 cells were found as 47.9, 38, and 31%, respectively [Citation36]. Cha et al. produced silk fibroin (SF) nanofiber scaffold containing microalgae Spirulina extract by electrospinning, and the scaffold were characterized, and biocompatibility was investigated. The cell viability results showed better cell proliferation than the pure SF nanofiber scaffold, and the Spirulina extract can promote cell growth [Citation37]. Our results of this analysis were expected, since B. braunii and M. aeruginosa oil extract did not have any cytotoxic effect on L929 cells, and polymers used in this study were biocompatible and non-toxic polymers. .
Different from the results of the analysis with L929 cell line, a slight decrease was seen in the viability of the PC-3, SHSY-5Y and AGS cells (). From this results, it was demonstrated that the polymers used in the nanoparticle structure were biocompatible as mentioned in with the L929 cells and there was no specific leakage from the nanoparticles for 24 h. Thus, the stability of the nanoparticle structure was shown, and extracts were not released immediately, instead they performed a gradual release. This is a formulation that can provide a long-term anticancer effect by providing controlled release in vivo condition without causing toxicity to normal tissues.
Conclusion
The results of this study indicated that the cytotoxic effects of the oil extracts of B. braunii and M. aeruginosa were promising enough for growth inhibition of various cancer cells without affecting the noncancerous cells. Among the cancer cells, the highest anticancer effect was observed for M. aeruginosa extracts on SHSY-5Y, then PC-3, and AGS cell lines, respectively. However, as it was mentioned in the Introduction section, bioactive substances like microalgal oil extracts can lose their activities since it was proven with the comparison of commercially obtained algal oil and freshly extracted samples. By using the electrospraying method, microalgal oil extract-loaded nanoparticles were produced, and the extracts were covered from environmental effects. In the light of these results, extracts of B. braunii and M. aeruginosa species seem promising to be used in pharmaceutical applications. Also, it is considered that algal nanoparticles will gain tremendous prominence with future studies.
Acknowledgements
Benan Inan was supported by TUBITAK-BIDEB 2228-B Doctoral scholarship program for graduate students. The authors also thank Betül Mutlu and Zeynep Karavelioğlu for their help in the XTT assay.
Disclosure statement
No potential conflict of interest was reported by the author(s).
Data availability statement
The authors confirm that the data supporting the findings of this study are available within the article.
Additional information
Funding
References
- İnan B. Microalgal bioprocess optimization, production of microalgal extract loaded nanoparticles and investigation of their anticancer effects [PhD Thesis]. Turkey: Yildiz Technical University; 2019.
- Ijaz H, Qureshi J, Tulain UR, et al. Lipid particulate drug delivery systems: a review. Bioinspired Biomim Nanobiomater. 2018;7(2):109–121.
- Wu Y, Sadatmousavi P, Wang R, et al. Self-assembling peptide-based nanoparticles enhance anticancer effect of ellipticine in vitro and in vivo. Int J Nanomedicine. 2012;7:3221.
- Li S, Wang A, Jiang W, et al. Pharmacokinetic characteristics and anticancer effects of 5-fluorouracil loaded nanoparticles. BMC Cancer. 2008;8(1):1–9.
- Puri A, Loomis K, Smith B, et al. Lipid-based nanoparticles as pharmaceutical drug carriers: from concepts to clinic. Crit Rev Ther Drug Carrier Syst. 2009;26(6):523–580.
- Cakir-Koc R, Budama-Kilinc Y, Kokcu Y, et al. Molecular docking of immunogenic peptide of Toxoplasma gondii and encapsulation with polymer as vaccine candidate. Artif Cells Nanomed Biotechnol. 2018;46(Suppl. 2):744–754.
- Biesalski HK, Dragsted LO, Elmadfa I, et al. Bioactive compounds: definition and assessment of activity. Nutrition. 2009;25(11–12):1202–1205.
- Sakai S, Sugawara T, Matsubara K, et al. Inhibitory effect of carotenoids on the degranulation of mast cells via suppression of antigen-induced aggregation of high affinity IgE receptors. J Biol Chem. 2009;284(41):28172–28179.
- Guiry MD. How many species of algae are there? J Phycol. 2012;48(5):1057–1063.
- Harun R, Singh M, Forde GM, et al. Bioprocess engineering of microalgae to produce a variety of consumer products. Renew Sustain Energy Rev. 2010;14(3):1037–1047.
- Brand-Williams W, Cuvelier ME, Berset C. Use of a free radical method to evaluate antioxidant activity. LWT. 1995;28(1):25–30.
- Roehm NW, Rodgers GH, Hatfield SM, et al. An improved colorimetric assay for cell proliferation and viability utilizing the tetrazolium salt XTT. J Immunol Methods. 1991;142(2):257–265.
- Ambati RR, Gogisetty D, Aswathnarayana Gokare R, et al. Botryococcus as an alternative source of carotenoids and its possible applications – an overview. Crit Rev Biotechnol. 2018;38(4):541–558.
- Cheng P, Okada S, Zhou C, et al. High-value chemicals from Botryococcus braunii and their current applications – a review. Bioresour Technol. 2019;291:121911.
- Concha E, Heipieper HJ, Wick LY, et al. Effects of limonene, n-decane and n-decanol on growth and membrane fatty acid composition of the microalga Botryococcus braunii. AMB Expr. 2018;8(1):1–8.
- Aboim JB, Oliveira D, Ferreira JE, et al. Determination of biodiesel properties based on a fatty acid profile of eight Amazon cyanobacterial strains grown in two different culture media. RSC Adv. 2016;6(111):109751–109758.
- Li Y, Li P, Xu P, et al. Effect of nutrients and antioxidant enzyme activities from Microcystis aeruginosa. Int J Curr Microbiol Appl Sci. 2016;5(8):571–576.
- Banskota AH, Sperker S, Stefanova R, et al. Antioxidant properties and lipid composition of selected microalgae. J Appl Phycol. 2019;31(1):309–318.
- Chu WL, Lim YW, Radhakrishnan AK, et al. Protective effect of aqueous extract from Spirulina platensis against cell death induced by free radicals apoptotic assay. 2010;10:1–8.
- NamKoong S, Joo H-M, Jang S-A, et al. Suppressive effects of fucoxanthin on degranulation in IgE-antigen complex-stimulated RBL-2H3 cells. Korean J Plant Resour. 2012;25(3):339–345.
- Yan C, Wang R, Wan J, et al. Cellulose/microalgae composite films prepared in ionic liquids. Algal Res. 2016;20:135–141.
- Senousy HH, Abd Ellatif S, Ali S. Assessment of the antioxidant and anticancer potential of different isolated strains of cyanobacteria and microalgae from soil and agriculture drain water. Environ Sci Pollut Res Int. 2020;27(15):18463–18474.
- Murugan K, Iyer VV. Differential growth inhibition of cancer cell lines and antioxidant activity of extracts of red, brown, and green marine algae. In Vitro Cell Dev Biol Anim. 2013;49(5):324–334.
- Atasever-Arslan B, Yilancioglu K, Kalkan Z, et al. Screening of new antileukemic agents from essential oils of algae extracts and computational modeling of their interactions with intracellular signaling nodes. Eur J Pharm Sci. 2016;83:120–131.
- Martins RM, Nedel F, Guimarães VBS, et al. Macroalgae extracts from Antarctica have antimicrobial and anticancer potential. Front Microbiol. 2018;9:1–10.
- Talero E, García-Mauriño S, Ávila-Román J, et al. Bioactive compounds isolated from microalgae in chronic inflammation and cancer. Mar Drugs. 2015;13(10):6152–6209.
- Czerwonka A, Kaławaj K, Sławińska-Brych A, et al. Anticancer effect of the water extract of a commercial Spirulina (Arthrospira platensis) product on the human lung cancer A549 cell line. Biomed Pharmacother. 2018;106:292–302
- Reyna-Martinez R, Gomez-Flores R, López-Chuken U, et al. Antitumor activity of Chlorella sorokiniana and Scenedesmus sp. microalgae native of Nuevo León State, México. PeerJ. 2018;6:e4358–15.
- Thien DVH, Hsiao SW, Ho MH. Synthesis of electrosprayed chitosan nanoparticles for drug sustained release. Nano Life. 2012;02(01):1250003.
- Bock N, Dargaville TR, Woodruff MA. Electrospraying of polymers with therapeutic molecules: state of the art. Prog Polym Sci. 2012;37(11):1510–1551.
- Nourmohammadi N, Soleimanian-Zad S, Shekarchizadeh H. Effect of Spirulina (Arthrospira platensis) microencapsulated in alginate and whey protein concentrate addition on physicochemical and organoleptic properties of functional stirred yogurt. J Sci Food Agric. 2020;100(14):5260–5268.
- Karakaş CY, Tekarslan Şahin H, İnan B, et al. In vitro cytotoxic activity of microalgal extracts loaded nano–micro particles produced via electrospraying and microemulsion methods. Biotechnol Prog. 2019;35:e2876.
- Karakaş CY, Özçimen D. A novel approach to production of Chlorella protothecoides oil-loaded nanoparticles via electrospraying method: modeling of critical parameters for particle sizing. Biotechnol Appl Biochem. 2020;1–10.
- Moghaddam MK, Mortazavi SM, Khayamian T. Preparation of calcium alginate microcapsules containing n-nonadecane by a melt coaxial electrospray method. J Electrostat. 2015;73:56–64.
- Gao Y, Zhao D, Chang MW, et al. Optimising the shell thickness-to-radius ratio for the fabrication of oil-encapsulated polymeric microspheres. Chem Eng J. 2016;284:963–971.
- Wang X, Zhang X. Separation, antitumor activities, and encapsulation of polypeptide from Chlorella pyrenoidosa. Biotechnol Prog. 2013;29(3):681–687.
- Cha BG, Kwak HW, Park AR, et al. Structural characteristics and biological performance of silk fibroin nanofiber containing microalgae spirulina extract. Biopolymers. 2014;101(4):307–318.