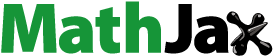
Abstract
The goal of the current study was to target 7-ethyl-10-hydroxycamptothecin (SN38) orally to colon tumours by synthesizing a targeting polymer. To achieve the optimum delivery for SN38, initially methoxy-polyethylene glycol (mPEG)-chitosan was synthesized and then nanoparticles were developed through ionic gelation between mPEG-chitosan and hyaluronic acid as a ligand for cell-surface glycoprotein CD44 receptor. The SN38 was loaded in nanoparticles (SN38-NPs) using the non-covalent physical adsorption method. The size of the optimized SN38-NPs was 226.7 nm, encapsulation efficiency was 89.23% and drug content was 7.98 ± 0.54% in the optimum formulation. The attachment of mPEG to chitosan was confirmed by proton nuclear magnetic resonance. The results of differential scanning calorimetry and Fourier transforms infra-red analysis indicated that SN38 existed in amorphous form and functional groups of SN38 protected in the formulations which could be a sign of suitable encapsulation of SN38 in SN38-NPs. In vitro study indicated that SN38-NPs were more potent against the cancer cells than free SN38. The cellular uptake of SN38-NPs improved up to 1.6-fold against human colorectal adenocarcinoma (Caco-2) cells. Moreover, SN38-NPs remarkably demonstrated superior anti-tumor efficacy in contrary to pure SN38. This suggests the advantage of SN38-NPs as a potent oral drug carrier which could be further explored for clinical investigations.
Introduction
Colon cancer is the fourth diagnosed cancer and mortal disease in both sexes worldwide. The abnormal changes of epithelial of the colon or rectum of the gastrointestinal tract cause colon cancer [Citation1]. In recent years, SN38 is an active metabolite of irinotecan hydrochloride (CPT-11) which is 100–1000 folds more potent than irinotecan especially employed in the treatment of many malignancies such as colon cancer [Citation2–4]. The in vivo conversion of CPT-11 to SN38 is less than 8% of the CPT-dosage applied, and dependent on patients, a large amount of CPT and its metabolites are eliminated through biliary and renal excretion [Citation5]. SN38 is unstable at physiological pH, the lactone ring converted to carboxylate form with no therapeutic effect [Citation6,Citation7]. However, the clinical application of SN38 is challenging due to its low solubility in aqueous and which leads to limited permeation across biological membrane followed by poor oral bioavailability [Citation8]. The oral route is the most popular route of administration as it is more convenient for the patients. However, there are significant barriers to the effective bioavailability of the oral drug, especially hydrophobic drugs such as SN38. In addition, when a drug administered orally, numerous digestive enzymes degrade the drug molecules, and the mucous lining of the gastrointestinal (GI) tract is a major obstacle to the absorption of the drug [Citation9]. Several scientific studies have been developed to demonstrate the benefits of oral drug delivery for colon cancer [Citation5,Citation9–14].
Various drug delivery systems have been comprehensively scrutinized to control the challenges associated with the administration of SN38. Among them, polymeric nanoparticles (NPs)- based oral drug delivery system have shown the intestinal permeation and a promising way to enrich its efficiency and safety [Citation15,Citation16].
Hyaluronic acid (HA) is a biocompatible carbohydrate polymer with an integrated backbone. HA is found in the human body and plays an important role in cell proliferation and linking with cellular receptors such as cell-surface glycoprotein CD44 [Citation17]. CD44 is overexpressed in several types of cancer cells, where nanoparticles consisting of HA can be taken up by the cells through CD44 receptor-mediated endocytosis. In addition, it is reported that HA is a factor causing high loading and selective targeting moiety [Citation18–21]. HA-based nanoparticles showed the increasing therapeutic effect of the oral formulation [Citation22]. HA has been used as a platform for combination chemotherapy such as in colorectal tumours to disturb oncogenic signalling [Citation23]. Combination of HA with other polymers generated an oral delivery vehicle for the BMI-1 inhibitor in CT26 colon tumour model and prevented metastasis gastrointestinal [Citation11].
Chitosan is a natural mucoadhesive polymer, which can directly attach to the GI surface and increase cellular uptake at the target site [Citation18,Citation24,Citation25]. Chitosan increases quinone reductase (QR) and glutathione S-transferases (GST) activities, and glutathione (GSH) concentration by deterrent ornithine decarboxylase (ODC) activity and cyclooxygenase-2 (COX-2) expression which causing chemo-preventive activity against colon cancer [Citation26]. Low molecular weight chitosan presents significant characteristics in clinical applications, improving solubility physiological pH and inhibitors of cancer growth [Citation27]. Methoxy-polyethylene glycol (mPEG) is a water-soluble polymer that exhibits low toxicity and improves the biocompatibility of nanoparticles. It could reduce the phagocytic effect and increase the durability of the half-life of nanoparticles in the systemic circulation by generating a barrier layer around nanoparticles to prevent adhesion of opsonins in blood [Citation15,Citation28]. Due to these advantages, grafting PEG to chitosan can develop nanoparticles for colon cancer drug delivery [Citation29]. Grafting of hydrophilic polymers such as PEG onto chitosan is a common strategy to enhance the solubility and biocompatibility of chitosan as well as to achieve lower recognition by the host immune system and improve blood circulation time [Citation30].
In many types of research, SN38 has been bonded to NPs through a chemical reaction [Citation6,Citation31,Citation32]. In our design, the non-covalent physical adsorption was applied to load drugs in nanoparticles without applying chemical reaction and toxic junction reagents. Polar- п interaction between the hydroxyl (OH) groups of HA and aromatic rings of SN38, the hydrogen bond between oxygen in the carbonyl group of SN38 and OH group of HA and chitosan selected to load SN38 on mPEG-chitosan/HA NPs.
Accordingly, the purpose of this study was to design a novel nanocarrier using chitosan, mPEG, and HA as the main ingredients via ionic gelation between cationic mPEG-chitosan conjugate and anionic HA. Then, physiochemical aspects of SN38-NPs and the cytotoxic potential were investigated in vitro, ex vivo and in vivo.
Materials
Low molecular weight chitosan (50 kDa) with the deacetylation degree of 85% was purchased from Sigma Aldrich (St. Louis, MO). The polymer HA sodium salt was obtained from Solarbio® (Beijing, China). mPEG molecular weight 5000 Da, sodium hydroxide (NaOH), glacial acetic acid, hydrochloric acid (HCl) and dimethylsulphoxide (DMSO) were obtained from Sigma Aldrich (Männedorf, Switzerland). SN38 was obtained from ABRTA CO. (Shaanxi, China).
Methods
Experimental design
The effects of three parameters including (pH, HA/mPEG- chitosan ratio and the amount of acid acetic) were investigated on size and zeta potential, the efficiency of drug loading and polydispersity index (PDI) of NPs. The dependent and independent variables are listed in . These three independent variables were defined as high level (+1), base (0) and minimum level (−1). The second order of polynomial function was used to evaluate the effect of each factor alone as well as the interaction of the factors as mentioned in EquationEquation (1)(1)
(1) :
(1)
(1)
Where, β0, βi, βii and βij represent the intercepts, linear, squared, and the interaction coefficients, respectively.
Table 1. Individual variables in Box-Behnken design.
The values of x1, x2, and x3 are three independent variables for investigating the optimum condition. To obtain the optimum conditions, i.e. achieving minimum size and maximum stable zeta potential, minimum PDI and maximum loading efficiency, 17 runs with five replications of the central point were investigated. The optimum condition was evaluated utilizing the design expert software (V. 10.0.0, Stat-Ease Inc., Minneapolis, MN), and the final response was compared with the predicted value [Citation33].
Synthesis of mPEG-chitosan conjugate
mPEG was attached to chitosan via amide bond formation. Briefly, 1 g of chitosan was dissolved in 10 ml of formic acid as a solvent, whereby a viscose solution was formed. Then, 1 g of mPEG was added to the above-mentioned solution and stirred for 15 min followed by dropwise addition of 1 ml of formaldehyde under constant stirring for 60 min at ambient temperature. The mixture was neutralized using 0.5 ml of 2 M NaOH. The obtained solution was purified through dialysis (molecular weight cut-off (MWCO): 12,000–14,000, Sigma-Aldrich, Schnelldorf, Germany) in deionized water for 24 h through replacing water three times. Next, the product was lyophilized (alpha 1-2 ld plus, Martin Christ GmbH, Germany) and stored in the fridge for further use [Citation34].
NPs were prepared via the ionic gelation method as described previously by Yang et al. [Citation35] with minor modifications. In brief, mPEG-chitosan at a concentration of 0.5 mg/ml was dissolved in 5 ml of deionized water containing acetic acid for 30 min with the pH adjusted according to the predetermined value () utilizing 1 M NaOH. HA at a concentration of 0.5 mg/ml was dissolved in deionized water. Subsequently, two solutions were mixed and stirred for 3 h. Thereafter, SN38 at a concentration of 10% (W/V) of the polymeric solution was dissolved in DMSO for 1 h. The SN38 solution was added to the reaction mixture dropwise under constant magnetic stirring at 800 rpm for 6 h at 25 °C. Afterward, the solution was sonicated using a probe sonicator (bandelin electronic type GM 3100, Berlin, Germany). The prepared nanoparticles were dialyzed (MWCO: 12,000–14,000, Sigma-AldrichGermany) in deionized water for 24 h after which the obtained nanoparticles were centrifuged (21,000 rpm, 30 min) and lyophilized.
Characterization of NPs
Particle size distribution and surface charge
The mean size, PDI, and zeta potential of obtaining nanoparticles were measured using the differential laser scattering method (DLS Zetasizer Nano ZPS instrument, Malvern, Worcestershire, UK). For sample preparation, 2 mg of samples was diluted to 4 ml with deionized water. Each measurement was carried out in triplicate.
Morphology of nanoparticles
The surface morphology of NPs was observed by scanning electron microscopy (SEM; Oxford Instruments, Abingdon, UK). The lyophilized NPs were fixed on the aluminium disc at room temperature, coated with gold by sputter coater and evaluated at 15 kV using 6300 fields.
Differential scanning calorimetry (DSC)
The thermal properties of NPs can be determined via DSC. The thermal behaviour of SN38-NPs was investigated via thermal analysis (DSC-823, Mettler Toledo, Geneva, Switzerland). The samples were sealed in aluminium pans and heated within 30–300 °C at a heating ramp 10 °C/min under nitrogen atmosphere at a flow rate of 50 cm3/min.
X-ray diffraction (XRD)
XRD of chitosan, mPEG, HA, SN38 and SN38-NPs were characterized using XRD (Rigaku, Ultima IV) with monochromatic copper K-α radiation. The scanning rate was 0.02° sec−1 from 5 to 80°.
Fourier transforms infra-red (FT-IR) analysis
The FT-IR spectra of mPEG, chitosan, mPEG- chitosan, HA, SN38, SN38-NPs were analyzed (Agilent Cary 630 FTIR spectrometer, Santa Clara, CA) within 400–4000 cm−1 at a resolution of 4 cm−1.
Drug content measurement
In order to measure the amount of drug loaded in NPs, a specified amount of SN38-NPs was dispersed in 1 ml of the solvent containing 500 µl of DMSO and 500 µl of deionized water. The mixture was sonicated for 15 min and centrifuged at 13,500 rpm for 30 min, after which the supernatant was separated and SN38 measured by high performance liquid chromatography (HPLC; Smart line; Knauer, Berlin, Germany). The HPLC method applied was the same as that explained earlier [Citation36]. The drug loading (DL) was analyzed using EquationEquation (2)(2)
(2) , while the encapsulation efficiency (EE) was calculated by EquationEquation (3)
(3)
(3) :
(2)
(2)
(3)
(3)
In vitro drug release study
To investigate the in vitro release, a special portion of prepared nanoparticles containing the equivalent to 2 mg of SN-38 was dispersed in 5 ml phosphate buffer saline (PBS; pH1.5, 6.8 and 7.4) to simulate gastric, simulated intestinal fluid and physiological intestine pH, respectively. The dialysis bag (MWCO: 12 KDa; Sigma Aldrich) immersed in a tube containing 45 ml of release medium. The tube rotates 60 rpm at 37 °C (IKA KS 4000 ic control, Staufen, Germany). At predetermined points, 1 ml of the PBS was collected, and 1 ml of fresh PBS was added to the medium to keep sink conditions. The collected PBS mixed with 1 ml of DMSO to extract SN-38 and the amount of SN38 was measured by HPLC. Drug release results were normalized by changing drug concentration in solution to a percentage of the cumulative drug release.
In vitro cytotoxic potential evaluation
The cytotoxic potential of NPs on human colorectal adenocarcinoma (Caco-2) cells, C26 and human non-cancer colon fibroblast CCD-18Co was evaluated using a resazurin assay [Citation37]. Caco-2 cells at a density of 25,000 cells/well in 24-well plates were cultured at 37 °C and 5% carbon-di-oxide (CO2) atmosphere. One day prior to conducting the test, the minimum essential medium (MEM) was changed to a medium of the same composition without Fetal bovine serum (FBS). C26 cells were cultured in Roswell Park Memorial Institute Medium-1640 supplemented with 10% (v/v) FBS and penicillin/streptomycin (100 U/ml each). CCD-18Co at a density of 25,000 cells/well in 24-well plates were cultured using Dulbecco's Modified Eagle's Media supplemented with 10% (v/v) Fetal bovine serum, and 1% (v/v) penicillin/streptomycin mix. The cells were maintained in an incubator at 37 °C and 5% CO2 atmosphere. Different concentrations of SN38 from 0.25–50 µg/ml were dissolved in a medium containing 0.5% DMSO, and NPs containing the same amount of SN38 were prepared in the same medium. MEM served as positive control while 2% (v/v) Triton X-100 solution served as a negative control. The cells were incubated at 37 °C and 5% CO2 atmosphere for 24 h. Then, the samples were removed, and the cells were washed twice with PBS buffer. Subsequently, 250 µl of 2.2 µM resazurin solution was added to the wells and incubated at 37 °C and 5% CO2 atmosphere for 3 h. Afterward, 100 µl of supernatant in each well was transferred to 96-well plate where the fluorescent intensity was measured at excitation and emission wavelengths of 540 and 590 nm, respectively, using a microplate reader (ELX800, Biotek, Winooski, VT). Cell viability was calculated according to EquationEquation (4)(4)
(4) :
(4)
(4)
In vitro cellular uptake studies
Cellular uptake of fluorescent SN38-NPs
Caco-2 cells and C26 were seeded at a density of 25,000 cells/well. Before cell uptake performance, cells were washed twice with PBS at pH 7.4. Then, 500 µl of pre-warmed fluorescein diacetate (FDA) loaded NPs (containing 5 µM of SN38 and at the concentration of 0.5% m/v, in MEM without FBS, pH 7.4) was added to cells. The cells were incubated for 2 and 6 h. NPs were removed from inside the cells, and the cells were washed twice. Then, 200 µl of 2% (V/V) Triton X-100 in 5 M NaOH was added to the cell. The lysis treatment without removing NPs was regarded as 100% while the cells incubated with MEM were considered as 0%.
The fluorescence intensity of lysate corresponding to the uptake efficiency was quantified using a microplate reader (ELX800, Biotek) at λex = 485 nm and λem= 535 nm. Cellular uptake was evaluated using EquationEquation (5)(5)
(5) :
(5)
(5)
Where Ft: fluorescence intensity of lysate, F100: fluorescence intensity of NPs without removal and F0: fluorescence intensity of corresponding 0%.
Cellular uptake by confocal microscopy
To evaluate the internalization of SN38-NPs on Caco-2 cells, confocal laser scanning microscopy (CLSM; Nikon Corporation, Seongbuk, Japan) was used. The Caco-2 cells were seeded as described before and then the cells were treated with 500 µl of pre-warmed loaded NPs for 2 and 6 h. The SN38-NPs were removed, and the cells were washed with PBS, fixed by 1.5 ml of ethanol (75% v/v) and measured under CLSM.
Ex-vivo release of SN38 from SN38-NPs
To investigate the release of SN38 adsorbed on SN38-NPs, 25 mg of NPs was dispersed in 5 ml PBS. Also, 10 cm of the large intestine of a male rat was removed and washed three times with Krebs-Ringer bicarbonate solution, pH 7.4. One side of the intestine was fastened, and the NPs suspension was poured into the intestine using a needle with the intestine placed in a tube with fifty ml of PBS. At predetermined intervals, 1 ml of the PBS was collected, and 1 ml of fresh PBS was added to the medium to keep sink conditions [Citation38]. The collected PBS was mixed with 1 ml of DMSO to extract SN38 where the amount of SN38 was measured via HPLC.
In vivo anti-tumor study
All animal studies were performed in accordance with the guidelines of the Institutional Ethical Committee and Research Advisory Committee of Semnan University. Briefly, BALB/c female mice (17–20 g) bearing C26 tumours were selected for all chemotherapy studies. 80 µL cell suspension including 3 × 105 C26 cells was injected into the mice. After 14 days, when the mean tumour size reached 15 ± 6 mm3, mice were divided into four groups (five mice in each group) and 0.2 ml of normal saline 0.9% as a negative control, free SN38 (3 mg/kg), SN38-NPs (3 mg/kg), NPs (without drug;160 mg/kg) was fed by oral route according to the previous literatures [Citation32]. Mice weight and tumour size was evaluated for 30 days. The average tumour volumes were calculated using the Equation (6):
(6)
(6)
Furthermore, the evaluation of the mice interrupted when the tumour size was larger than 2 cm in one dimension or they lost more than 80% of the body weight [Citation31,Citation32].
All animal studies were approved by the Ethics Review Committee for Animal Experimentation of Semnan University under ID 992113.
Statistical data analysis
Graph pad prism version 5.01, La Jolla, CA was used for statistical analysis. The one-way analysis of variance (ANOVA) and Tukey multiple comparison post-test were used. All data were expressed as the mean ± standard deviation with at least three experiments.
Results and discussion
Synthesis of mPEG-chitosan conjugate
For preparing SN38-NPs, mPEG-chitosan was synthesized. Free amino groups of chitosan reacted with formaldehyde to form an intermediate of Schiffʼs bases where this intermediate was reacted with a OH group of mPEG: one side of mPEG contained by methoxy and the other side reacting with the amino group (Figure S1). The reaction between the amino group of chitosan and OH group of mPEG caused amide П bond. The attachment of mPEG to chitosan was confirmed by proton nuclear magnetic resonance (1H NMR;400.133 MHZ, DMSO-d6). As shown in Figure S2(A), the peak at 3.325 ppm was related to C2-H of chitosan. In Figure S2(B), the signal assignable to the proton of mPEG units (-CH2-CH2-O-) appearing at 3.51–3.699 ppm and the sharp peak at 3.31 ppm was related to methoxy of mPEG. As displayed in Figure S2(C), the peak at 3.512 ppm is associated with (-CH2-CH2-O-) of mPEG and the broad peak appearing at 4.432 ppm is attributed to hydrogen at the amine group of NH-CH2-O confirming the conjunction between the amine group of chitosan and mPEG. The degree of PEGylation was determined from the 1H NMR spectrum by integrating the ratio of the area between the peaks of substitution (-CH2-CH2-O-) of mPEG and amine group of NH-CH2-O, which was 1.2%.
Figure S3(A) shows the FT-IR spectra of chitosan, we can observe the bands within 3292–3363 cm−1 assigned to N-H and O-H stretching. Peaks at 2869 and 2923 cm−1 can be attributed to C-H symmetric and asymmetric stretching, respectively. The peak at 1590 cm−1 is related to the N-H bending of the primary amine. The absorption peak at 1260 cm−1 was attributed to the bending vibrations of hydroxyls in chitosan. Figure S3(B) represents characteristic peaks of mPEG at 2881 and 1466 cm−1 which were related to C-H stretching and C-H scissoring, respectively. The mPEG-chitosan shows all characteristic peaks of chitosan. Also, the peak at 1582 cm−1 in Figure S3(C) indicates a crosslinking bond due to the interaction between chitosan and mPEG. Also, the elimination of the broad peak of mPEG in mPEG-chitosan demonstrates that the free OH group of mPEG reacted with the amine group of chitosan.
Preparation of SN38-NPs
The size of NPs directly affects bioavailability and blood circulation time. Particles with 100 − 200 nm have demonstrated more prolonged blood circulation times [Citation27]. The Zeta potential determines NPs stability and the interaction between NPs and the cell membrane [Citation39]. High zeta potential increases repellent forces between particles, preventing aggregation of particles [Citation37]. NPs with different ranges of PDI have different capabilities to carry the drug to cells. Box-Behnken method was applied to evaluate the optimum level of individual parameters, as shown in , on the responses. The results are displayed in Table S1. The significance of the model and each coefficient was tested via ANOVA combined with the application of F-test as well as t-test, where p < .05 was considered significant [Citation33]. The ANOVA analysis for the fitting model have been investigated in Table S2. The results were fitted by Equations (7-10):
(7)
(7)
(8)
(8)
(9)
(9)
(10)
(10)
Where, Y1, Y2, Y3, and Y4 represent the size, zeta potential, PDI, and encapsulation efficiency. A, B and C represent pH, the HA and mPEG-chitosan ratio and acetic acid concentration, respectively. The R2 coefficients of the model for size, zeta potential, PDI, and encapsulation efficiency were 0.9786, 0.9829, 0.8309 and 0.8294, respectively. Furthermore, the p = .0001 means these models very well fit the response. The ANOVA analysis (Table S 2) illustrates the lack of fit F-values of 4.3, 2.2, 0.072 and 0.2 that were obtained for size, zeta potential, PDI and encapsulation efficiency, respectively. The model F-values were 45.7, 44.75, 3.82 and 3.78 for size, zeta potential, PDI and encapsulation efficiency, respectively. The results show the model used for each response was significant and the lack of fit of each model was not significant (p <.05), as desired [Citation40]. According to the ANOVA test, the quadratic model was the best-fitted model for all responses.
shows that with increasing HA/mPEG-chitosan, the size of NPs has grown, but at the low concentration of polyanion, there is not enough negative charge to cover the positive charge of the amine group in chitosan and the particles are not stable [Citation27]. shows the elevation of the pH from 3 to 4.2 has caused size reduction. However, further elevation of pH has enlarged the particles. The pH affects the ionization of carboxylic acid groups of HA and amino groups of chitosan, which interfere with the electrostatic interaction between two polymers. The pKa of chitosan is 6.5 below which the isoelectric point, the amino groups are protonated, and the charge of chitosan is positive. Also, the pKa of HA is close to 2.6, where pH elevation causes a more negative charge of carboxylic acid [Citation41]. Under acidic conditions, more protonated chitosan can interact better with the negative charge HA, but under very acidic conditions at pH ˂ 4.2, probably due to reduction of the negative charge of HA, less interaction occurs between two polymers, and the particle size is larger than at pH 4.2 to 4.8. With the elevation of pH from 4.2 to 6, the particles grew larger, suggesting a direct relationship between pH rise and HA dissociation due to lower protonation of amino of chitosan and the formation of aggregated NPs [Citation19]. The effect of HA/mPEG-chitosan in acidic pH is less than at high pHs. This is because at higher pHs, there is no electrostatic interaction between two opposite polymers, and probably larger particles form because of intermolecular hydrogen bonding. Based on , the reduction of zeta potential from positive to negative shows a direct relationship with the HA/mPEG-chitosan concentration rise. The negative zeta potential shows that the presence of carboxylic groups shields the amino groups on the surface of particles. As displayed in , there is a considerable reduction of zeta potential with pH elevation; when the pH increased, the positive amino groups decreased, and more negative particles were formed. On the other hand, as shown in , the effect of acetic acid concentration on zeta potential is less significant. In , with the elevation of the HA/mPEG-chitosan, PDI has increased. The results suggest that increasing mPEG-chitosan/HA causes more unstable particles that coagulate with each other.
Figure 1. Effects of HA/mPEG-chitosan and pH as well as pH and acetic acid concentration on (A,B) size, ((C,D) zeta potential, (E,F) PDI and (G,H) encapsulation efficiency.
shows that by reducing the pH from 6 to 5, PDI has decreased and alteration of pH to 3.5 has caused more reduction in PDI. The effect of pH on PDI is the same as its impact on the size.
With the elevation of the acid acetic concentration, shows the creation of heterodisperse particles. The rise in the acetic acid concentration causes an increase in more counter ions, which shields amino groups of chitosan, causing contraction of chitosan molecules [Citation41].
As displayed in , with the elevation of HA/mPEG-chitosan and pH, the encapsulation efficiency has increased as larger NPs can incorporate more drugs. Further, the increase in pH and acetic acid in shows the same effect on drug loading efficiency. In addition, HPLC analysis confirmed no drug degradation, indicating drug stability.
After analyzing the data to find the optimized formulation, by solving EquationEquations (1(1)
(1) –Citation4), the optimum values predicted by software for independent variables were reported in Table S 3 with overall desirability of 0.73. The optimum conditions obtained were repeated three times. The data indicated that the model has good acceptability to predict optimum conditions. As shown in , the size of the SN38-NPs is 226 nm and the encapsulation efficiency under optimum condition was 89.23% with the drug content being 7.98 ± 0.54%. displays the morphological study of SN38-NPs by SEM. The SN38-NPs are uniform and spherical with narrow size distribution and no aggregation and clumping were observed.
DSC
DSC was done to investigate the thermal properties and the physical state of the drug in SN38-NPs. The amorphous or crystalline form of the drug can affect the in vitro and in vivo release. As exhibited in , chitosan exhibited an exothermic peak at 140 °C, while HA revealed a broad peak at 106 °C and an exothermic peak at 239 °C. SN38 indicated an endothermic melting point at 287 °C and a characteristic peak at 225 °C which disappeared in the thermogram of SN38-NPs. The transformation of SN38 into the amorphous state in SN38-NPs revealed better wettability and significant pharmacokinetic properties [Citation42]. Also, the disappearance of HA peaks indicates the interaction between HA, chitosan, and SN38.
XRD
The XRD patterns of chitosan, mPEG, HA, SN38 and SN38-NPs are shown in Figure S4. The characteristic peaks of SN38 appeared at 11.04, 17.88, 23.96 and 26.24°, respectively revealed the crystalline structure of SN38. The intensity of these sharp peaks of SN38 was reduced or disappeared in the case of SN38 NPs (Figure S4(E)) which reflects that SN38 existed in an amorphous state in SN38-NPs. The specific peaks of chitosan at 19.68°, mPEG at 19.12°, 23.2° and HA at 20.2° were disappeared in SN38-NPs due to conversion of the crystalline form to the amorphous form. The amorphous pattern of SN38 in SN38-NPs enhanced the solubility of the drug [Citation13].
FT-IR analysis
FT-IR analysis was used to characterize the interaction between polymers and chemical groups on the surface of SN38-NPs, with the chemical groups of chitosan, mPEG and mPEG-chitosan discussed in the section “Experimental design”. In the spectrum of HA in , the peaks at 1607 and 1720 cm−1 correspond to amide and carbonyl bonds of glucuronic and N-acetyl-glucosamine of HA respectively, and the peak at 1032 cm−1 represents C-OH linkage stretching. The peaks at 1733 cm−1 in indicate the lactone ring and the peaks at 1620 cm−1 represents the carbonyl amide group of pure SN38. In , the peaks at 1734 and 1622 cm−1 mark the lactone ring and carbonyl group confirming the adsorption of SN38 on SN38-NPs. These peaks are weaker than the peaks in which means by adsorption of SN38, the intramolecular hydrogen bonds in pure SN38 were eliminated and intermolecular hydrogen bond between O-H, C=O of HA, the amine group of chitosan and the lactone ring and carbonyl group of SN38 were formed [Citation43,Citation44]. In addition, the lactone form of SN38 is dominant which is more significant for its cytotoxic activity [Citation6]. The peaks at 2881 and 1466 cm−1 which were contributed to C-H stretching and C-H scissoring of mPEG, respectively showed in the final spectrum. The peak at 1582 cm−1 in indicates a crosslinking bond due to the interaction between chitosan and mPEG which indicates the free OH group of mPEG reacted with amine group of chitosan. The SN38-NPs spectrum shows all contributed polymers and pure SN38 significant peaks.
In vitro drug release study
Release study of SN38 from SN38-NPs over one week at pH 1.2, 6.8 and 7.4 as simulated environmental of the gastric, simulated intestinal fluid and simulated colonic fluid conditions are shown in Figure S5. In all pH, the release of SN38 from SN38-NPs showed sustained and prolonged release. Total SN38 release in pH 1.2, 6.8 and 7.4 was 9.78 ± 2.69%, 22.3 ± 4.12% and 49.23 ± 4.18%, respectively after 168 h. The cumulative release of SN38 from nanoparticles at pH 1.2 was inhibited. Therefore, SN-38 at gastric fluid shows less release in comparison to intestinal and colonic fluid at the same temperature. Chitosan can interact with the anionic drug to form complexes; this complex can protect the drug from premature release at the upper gastrointestinal tract and causing the drug release at specific colon tumours [Citation45]. This pattern of release favoured to target the drug by oral drug delivery. These results are consistent with previous studies [Citation5,Citation12,Citation13].
In vitro cell viability effect
The in vitro cytotoxicity of NPs, free SN38 and SN38-NPs were evaluated on Caco-2 and C26 cells (cancerous positive cells) and the cytotoxicity of SN38-NPs was investigated on CCD-18co (cancerous negative cells). Resazurin assay was performed to examine the cytotoxic test on Caco-2and C26 cells. As depicted in , the SN38-NPs at different concentrations exhibited no cytotoxic on the CCD-18co. SN38-NPs () indicated a significant cytotoxic potential effect (p ˂.05) in all concentrations (1.25–10 µM) compared to free SN38 but NPs exhibited no cytotoxicity effect and also SN38-NPs showed cytotoxicity in C26 cells (). One of the important features of biodegradable NPs is that they should not affect the viability of cells [Citation34]. The overall increase in the cytotoxic effect of SN38-NPs demonstrated that the interaction between CD44 receptor expression on cancer cells and HA moiety on the surface of SN38-NPs resulting more accumulation of SN38 into the cancer cells, resulting in more cytotoxicity against cancer cells [Citation46].
In vitro cellular uptake studies
Cellular uptake of fluorescent SN38-NPs
The cellular uptake of free SN38, SN38-NPs and SN38-NPs without HA on Caco-2 and C26 are represented in . The obtained results showed that cellular uptake of SN38-NPs was two-fold higher than SN38-NPs without HA, as well as 3.83-fold higher than free SN38 on Caco-2 cells. The obtained result clearly indicated the higher uptake of SN38-NPs compared to SN38-NPs without HA can be related to CD44 receptor-mediated endocytosis [Citation46]. The lower cellular uptake of SN38 in comparison with SN38-NPs revealed that passively transportation of free SN38 into the cytoplasm may be caused efflux out by P-glycoprotein (P-gp) pumps [Citation47]. Caco-2 cell line model is used to investigate the intestinal permeability of drugs. Caco-2 cells formed a monolayer of polarized cells after differentiation which provides tight junctions and active transporters such as the P-glycoprotein. 3.83-fold enhancement of SN38-NPs compare to free SN38 could be explained modification of the surface of NPs by mPEGylated chitosan, which causes stealth property and cannot be recognized by P-gp [Citation48,Citation49]. Choie et al. [Citation50] demonstrated that the surface modification of NPs using HA and PEG caused 1.6-fold greater accumulation in the tumour tissue compared to bare NPs.
Figure 6. In vitro cellular uptake of free SN38, SN38-NPs and SN38- NPs without HA after 2 h and after 6 h (A) Caco-2 and (B) C26 (mean ± SD; n = 3). CLSM of Caco-2 cells (C) 2 h and (D) 6 h incubation with FDA loaded SN38-NPs with 20 x magnification level.
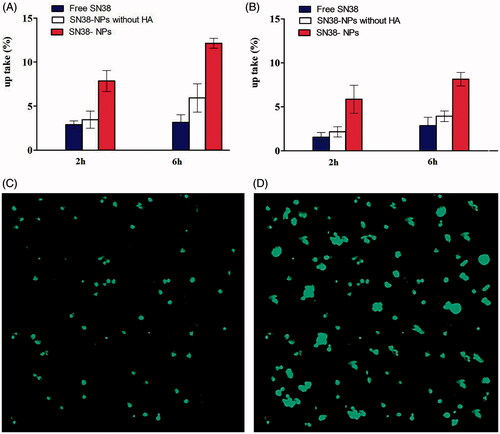
shows the uptake of SN38-NPs is 2.89-fold higher than free SN38 and 2.07-fold higher than SN38-NPs without HA after 6 h. Higher drug concentrations in Caco-2 cells in comparison with C26 cells was due to more P-gp in Caco-2 cells and more efficacy of efflux inhibitors such as PEGylated chitosan [Citation51].
Cellular uptake by CLSM
The cellular uptake of fluorescent SN38-NPs was traced by CLSM to confirm the internalization of SN38-NPs into cells in . When fluorescent SN38-NPs inter to the cells, the cells take up the whole ingredients, not separately [Citation43]. The CLSM images indicate the intensity of fluorescent SN38-NPs progressed after 6 h. The strong green emission shows that more SN38 distributed in the cytoplasm by passing the time, this result is similar to Gu et al. [Citation43].
Ex-vivo release of SN38 from SN38-Nps
In this study, the rat intestine was used for measuring the transport of SN38-NPs and free SN38 across the intestinal barrier. As shown in , the transport of SN38-NPs from the intestinal barrier (17.4%) was significantly (p < .01) higher than that of free SN38 (5.3%). The sustained release of SN38-NPs is a result of NPs stability and modification of the SN38-NPs with PEGylated chitosan. Accordingly, PEGylated chitosan can improve the transport of SN38 from the intestine and opens a tight junction [Citation32]. Furthermore, the mPEG brushes are more hydrophilic and cause water penetration into NPs thereby NPs are more flexible and pass the intestinal barrier more easily than free SN38 [Citation7].
In vivo anti-tumor study
Therapeutic efficacy of free SN38, SN38-NPs, NPs (without SN38), normal saline as control was investigated on the C26 subcutaneous tumour model of colon carcinoma. Mice were treated with SN38-NPs (two times in a week for four weeks, equivalent SN38 concentration of 3 mg/kg), free SN38 (two times in a week for fourweeks, 3 mg/kg), NPs (without SN38) and normal saline. The tumour size (mm3) and body weight loss of mice were evaluated for 30 days. SN38 was fed at a dosage of 3 mg/kg into the mice because the minimum dosage that mice can tolerate is 3 mg/kg [Citation31].
As shown in , SN38-NPs exhibited more therapeutic index efficacy in suppressing tumour growth compared with SN38, NPs or normal saline tumour treated mice. On the other hand, the results in demonstrated free SN38 had no obvious efficacy in inhibiting tumour growth. The results are in good agreement with cytotoxicity results, the higher cellular transportation of SN38 by implementing SN38-NPs increased more cellular toxicity and reduce tumour growth rate. The toxicity of all treated groups was analyzed based on body weight loss (BWL). The results in demonstrated no obvious BWL detected in any group. Lack of any serious BWL loss might have been related to the negligible toxicity effect of the formulation doses.
Figure 8. In vivo anti-tumour efficacy of free SN38, NPs, SN38-NPs and normal saline into C26-tumour-bearing mice during the 30 days with respect to (A) tumour volume, (B) image of solid tumour and (C) body weight (mean ± SD; n = 5).
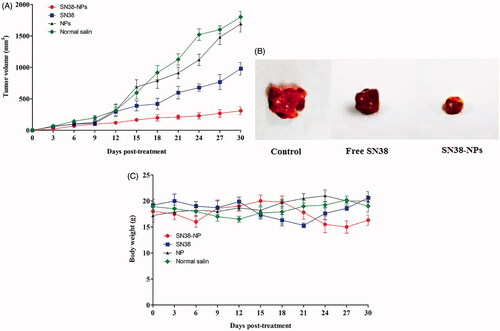
Several positive features make SN38-NPs as a high therapeutic and intelligent delivery system. First, modifying the surface of NPs by PEGylated chitosan allows the NPs cannot be recognized by the mononuclear phagocyte system (MPS) and increased the half-life time of SN38-NPs in the blood system [Citation52]. Secondly, CD44 expression in colon cancer tissue is higher than normal mucosa and it is related to cancer aggressiveness. The N-terminal of CD44 proteins was known as a receptor of HA [Citation18]. Thereby, Implementing HA on the surface of SN38-NPs as active targets resulting in a specific and intelligent drug delivery system. Third, Tumour tissues have lymphatic drainage and leaky vasculature which causing enhanced permeability and retention (EPR) effect for NPs with size 10–220 nm. All these features can improve the tumour accumulation of SN38-NPs, increase more penetration and longer residence time in tumours which providing more cellular uptake of SN38-NPs compare with free SN38.
Conclusions
In this study, SN38-NPs were synthesized using biodegradable polymers such as chitosan, mPEG, and HA under optimal conditions for colon delivery. The SN38-NPs exhibited homogenized dispersity without aggregation. The SN38-NPs revealed more significant cytotoxic potential on Caco-2 cells rather than free SN38 with no cytotoxic effect observed in CCD-18Co normal cells. The synergistic effect between constituents of NPs such as mPEG assists SN38 in evading from macrophages and HA, which is a primary ligand for the CD44 receptor, would significantly increase the cellular uptake of SN38-NPs in comparison with free HA NPs. The in vivo study demonstrated SN38-NPs are a favourable efficacy drug delivery system against C26 tumour-bearing mice. Overall, the prepared NPs as compared to other SN38 delivery systems appear to be more timesaving and are a promising solution for oral delivery.
Supplemental Material
Download MS Word (401 KB)Acknowledgements
The financial support provided by the Research vice Presidency of Semnan University and Mazandaran Medical Science University.
Disclosure statement
Authors have declared that no competing interests exist.
References
- Khalek FJA, Gallicano GI, Mishra L. Colon cancer stem cells. Gastrointest Cancer Res. 2010;(Suppl 1):S16.
- Ebrahimnejad P, Dinarvand R, Jafari MR, et al. Characterization, blood profile and biodistribution properties of surface modified PLGA nanoparticles of SN-38. Int J Pharm. 2011;406(1-2):122–127.
- Mohammady H, Dinarvand R, Esfandyari MM, et al. Encapsulation of irinotecan in polymeric nanoparticles: characterization, release kinetic and cytotoxicity evaluation. Nanomed J. 2016;3(3):159–168.
- Ebrahimnejad P, Dinarvand R, Sajadi S, et al. Preparation and characterization of poly lactide-co-glycolide nanoparticles of SN-38. PDA J Pharm Sci Technol. 2009;63(6):512–520.
- Guo M, Rong W-T, Hou J, et al. Mechanisms of chitosan-coated poly(lactic-co-glycolic acid) nanoparticles for improving oral absorption of 7-ethyl-10-hydroxycamptothecin. Nanotechnology. 2013;24(24):245101–245120.
- Ebrahimnejad P, Dinarvand R, Sajadi A, et al. Preparation and in vitro evaluation of actively targetable nanoparticles for SN-38 delivery against HT-29 cell lines. Nanomedicine. 2010;6(3):478–485.
- Ramezani P, Abnous K, Taghdisi SM, et al. Targeted MMP-2 responsive chimeric polymersomes for therapy against colorectal cancer. Colloids Surf B Biointerfaces. 2020;193:111135.
- Ebrahimnejad P, Jafari M, Heidari D. An efficient method for fabrication of carboxylated short multi-walled carbon nanotubes decorated with magnetic iron oxide nanoparticles. Curr Nanosci. 2013;9(5):615–618.
- Dinarvand M, Kiani M, Mirzazadeh F, et al. Oral delivery of nanoparticles containing anticancer SN38 and hSET1 antisense for dual therapy of colon cancer. Int J Biol Macromol. 2015;78:112–121.
- Alkhader E, Roberts CJ, Rosli R, et al. Pharmacokinetic and anti-colon cancer properties of curcumin-containing chitosan-pectinate composite nanoparticles. J Biomater Sci Polym Ed. 2018;29(18):2281–2298.
- Xu J, Zhang Y, Xu J, et al. Reversing tumor stemness via orally targeted nanoparticles achieves efficient colon cancer treatment. Biomaterials. 2019;216:119247.
- Roger E, Lagarce F, Benoit J-P. Development and characterization of a novel lipid nanocapsule formulation of Sn38 for oral administration. Eur J Pharm Biopharm. 2011;79(1):181–188.
- Prasad S, Dangi J. Development and characterization of pH responsive polymeric nanoparticles of SN-38 for colon cancer. Artif Cells Nanomed Biotechnol. 2016;44(8):1824–1834.
- Bala V, Rao S, Li P, et al. Lipophilic prodrugs of SN38: synthesis and in vitro characterization toward oral chemotherapy. Mol Pharmaceutics. 2016;13(1):287–294.
- Mir M, Ebrahimnejad P. Preparation and characterization of bifunctional nanoparticles of vitamin E TPGS-emulsified PLGA-PEG-FOL containing deferasirox. J Nanosci Nanotechnol. 2015;4(2):80–87.
- Lin PY, Chiu YL, Huang JH, et al. Oral nonviral gene delivery for chronic protein replacement therapy. Adv Sci. 2018;5(8):1701079.
- Almalik A, Donno R, Cadman CJ, et al. Hyaluronic acid-coated chitosan nanoparticles: molecular weight-dependent effects on morphology and hyaluronic acid presentation. J Control Release. 2013;172(3):1142–1150.
- Xia P, Xu X-Y. Prognostic significance of CD44 in human colon cancer and gastric cancer: Evidence from bioinformatic analyses. Oncotarget. 2016;7(29):45538–45546.
- Sadeghi Ghadi Z, Ebrahimnejad P. Curcumin entrapped hyaluronan containing niosomes: preparation, characterization and in vitro/in vivo evaluation . J Microencapsul. 2019;36(2):169–179.
- Sadeghi-Ghadi Z, Vaezi A, Ahangarkani F, et al. Potent in vitro activity of curcumin and quercetin co-encapsulated in nanovesicles without hyaluronan against Aspergillus and Candida isolates. J Mycol Med. 2020;30(4):101014.
- Ghadi ZS, Dinarvand R, Asemi N, et al. Preparation, characterization and in vivo evaluation of novel hyaluronan containing niosomes tailored by Box-Behnken design to co-encapsulate curcumin and quercetin. Eur J Pharm Sci. 2019;130(2):234–246.
- Hsieh C-M, Huang Y-W, Sheu M-T, et al. Biodistribution profiling of the chemical modified hyaluronic acid derivatives used for oral delivery system. Int J Biol Macromol. 2014;64:45–52.
- de Souza AB, Chaud MV, Santana MHA. Hyaluronic acid behavior in oral administration and perspectives for nanotechnology-based formulations: a review. Carbohydr Polym. 2019;222:115001.
- Pereira LA, da Silva Reis L, Batista FA, et al. Biological properties of chitosan derivatives associated with the ceftazidime drug. Carbohydr Polym. 2019;222:115002.
- Khalili S, Ebrahimnezhad P. Survival of Lactobacillus Acidophilus as probiotic bacteria using chitosan nanoparticles. Int J Eng. 2017;30(4):456–463.
- Khan Z, Pillay V, Choonara YE, et al. Drug delivery technologies for chronotherapeutic applications. Pharm Dev Technol. 2009;14(6):602–612.
- Duceppe N, Tabrizian M. Factors influencing the transfection efficiency of ultra low molecular weight chitosan/hyaluronic acid nanoparticles. Biomaterials. 2009;30(13):2625–2631.
- Jafari M, Heidari D, Ebrahimnejad P. Synthesizing and characterizing functionalized short multiwall carbon nanotubes with folate, magnetite and polyethylene glycol as multi-targeted nanocarrier of anti-cancer drugs. Iran J Pharm Res. 2016;15(2):449–456.
- Chung-Wook C, Kyu-Don C, Young-Il J, et al. 5-aminolevulinic acid-incorporated nanoparticles of methoxy poly(ethylene glycol)-chitosan copolymer for photodynamic therapy. Int J Nanomedicine. 2013;8(1):809–819.
- Zhang X, Zhang H, Wu Z, et al. Nasal absorption enhancement of insulin using PEG-grafted chitosan nanoparticles. Eur J Pharm Biopharm. 2008;68(3):526–534.
- Taghavi S, Abnous K, Babaei M, et al. Synthesis of chimeric polymersomes based on PLA-b-PHPMA and PCL-b-PHPMA for nucleoline guided delivery of SN38. Nanomed Nanotechnol Biol Med. 2020; 28:102227.
- Alibolandi M, Amel Farzad S, Mohammadi M, et al. Tetrac-decorated chitosan-coated PLGA nanoparticles as a new platform for targeted delivery of SN38. Artif Cells Nanomed Biotechnol. 2018;46(sup2):1003–1014.
- Taleghani AS, Ebrahimnejad P, Heidarinasab A, et al. Sugar-conjugated dendritic mesoporous silica nanoparticles as pH-responsive nanocarriers for tumor targeting and controlled release of deferasirox. Mater Sci Eng C Mater Biol Appl. 2019;98(85):358–368.
- Kulkarni AR, Hukkeri VI, Sung HW, et al. A novel method for the synthesis of the PEG-crosslinked chitosan with a pH-independent swelling behavior. Macromol Biosci. 2005;5(10):925–928.
- Yang L, Gao S, Asghar S, et al. Hyaluronic acid/chitosan nanoparticles for delivery of curcuminoid and its in vitro evaluation in glioma cells. Int J Biol Macromol. 2015;72(10):1391–1401.
- Ebrahimnejad P, Dinarvand R, Sajadi A, et al. Development and validation of an ion-pair HPLC chromatography for simultaneous determination of lactone and carboxylate forms of SN-38 in nanoparticles. J Food Drug Anal. 2009;17(4):246–256.
- Sharifi F, Nazir I, Asim MH, et al. Zeta potential changing self-emulsifying drug delivery systems utilizing a novel Janus-headed surfactant: a promising strategy for enhanced mucus permeation. J Mol Liq. 2019;291(15):111285–111295.
- Taleghani AS, Ebrahimnejad P, Heydarinasab A, et al. Adsorption and controlled release of iron-chelating drug from the amino-terminated PAMAM/ordered mesoporous silica hybrid materials. J Drug Delivery Sci Technol. 2020;56:101579.
- Sharifi F, Jahangiri M. Investigation of the stability of vitamin D in emulsion-based delivery systems. Chem Ind Chem Eng. 2018;24(2):28.
- Nazeri N, Avadi MR, Faramarzi MA, et al. Effect of preparation parameters on ultra low molecular weight chitosan/hyaluronic acid nanoparticles. Int J Biol Macromol. 2013;62:642–646.
- Prego C, Torres D, Fernandez-Megia E, et al. Chitosan-PEG nanocapsules as new carriers for oral peptide delivery. Effect of chitosan pegylation degree. J Control Release. 2006;111(3):299–308.
- Sun X, Dabu Zhu YC, Shi G, et al. One-step mechanochemical preparation and prominent antitumor activity of SN-38 self-micelle solid dispersion. Int J Nanomed.. 2019;14(14):2115–2126.
- Gu Q, Xing JZ, Huang M, et al. SN-38 loaded polymeric micelles to enhance cancer therapy. Nanotechnology. 2012;23(20):205101–205115.
- Varnamkhasti BS, Hosseinzadeh H, Azhdarzadeh M, et al. Protein corona hampers targeting potential of MUC1 aptamer functionalized SN-38 core-shell nanoparticles. Int J Pharm. 2015;494(1):430–444.
- Wu Q-X, Lin D-Q, Yao S-J. Design of chitosan and its water soluble derivatives-based drug carriers with polyelectrolyte complexes. Mar Drugs. 2014;12(12):6236–6253.
- Vangara KK, Liu JL, Palakurthi S. Hyaluronic acid-decorated PLGA-PEG nanoparticles for targeted delivery of SN-38 to ovarian cancer. Anticancer Res. 2013;33(6):2425–2434.
- Kibria G, Hatakeyama H, Harashima H. Cancer multidrug resistance: mechanisms involved and strategies for circumvention using a drug delivery system. Arch Pharm Res. 2014;37(1):4–15.
- Balimane PV, Chong S. Cell culture-based models for intestinal permeability: a critique. Drug Discov Today. 2005;10(5):335–343.
- Hilgers AR, Conradi RA, Burton PS. Caco-2 cell monolayers as a model for drug transport across the intestinal mucosa. Pharm Res. 1990;7(9):902–910.
- Choi KY, Min KH, Yoon HY, et al. PEGylation of hyaluronic acid nanoparticles improves tumor targetability in vivo. Biomaterials. 2011;32(7):1880–1889.
- Nasirizadeh S, Jaafari MR, Iranshahi M, et al. The effect of efflux pump inhibitors on in vitro and in vivo efficacy of solid lipid nanoparticles containing SN38. J Drug Delivery Sci Technol. 2020;60:101969.
- Blanco E, Shen H, Ferrari M. Principles of nanoparticle design for overcoming biological barriers to drug delivery. Nat Biotechnol. 2015;33(9):941–951.