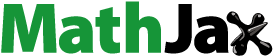
Abstract
Zinc oxide nanoparticles (ZnO-NPs) have been produced by physical and chemical methods. Here, the comparative evaluation of both chemically-synthesised ZnO-NPs (C-ZNPs) and in-vitro cultured S. marianum mediated green-synthesised ZnO-NPs (G-ZNPs) were investigated on seed germination frequency, root and shoot growth, callus induction and biochemical profile of medicinally important plant Silybum marianum. Of all the treatments, callus-mediated ZnO-NPs gave optimum results for seed germination (65%), plantlet’s root length (4.3 cm), shoot length (5.3 cm) and fresh and dry weights (220.4 g L−1 and 21.23 g L−1, respectively). Similarly, the accumulation of phenolic (12.3 µg/mg DW) and flavonoid (2.8 µg/mg DW) contents were also enhanced in callus cultures treated with G-ZNPs. We also observed maximum antioxidant activity (99%) in callus cultures treated with G-ZNPs, however, in case of plantlets, these activities were found highest for in-vitro whole plant-mediated ZnO-NPs. Moreover, G-ZNPs also enhanced total protein content (265.32 BSAE/20g FW) in callus cultures. G-ZNPs were further assessed for their effects on several multidrug resistant bacterial strains and human liver carcinoma (HepG2) cells and our findings revealed that callus extracts treated with G-ZNPs show ameliorated antibacterial (highest zone of inhibition (19 mm) against Klebsiella pneumonia) and anticancer (highest cytotoxicity of 64%) activities.
Introduction
Nanotechnology has applications in almost every field, ranging from material sciences to biotechnology. The most appealing one is to improve the ability of plants to absorb nutrients by revolutionising the agriculture with new tools [Citation1]. Nanomaterials having dimension from 1 to 100 nm are progressively greater in practices for many commercial goals i.e. semiconductors, catalysts, cosmetics or drug carriers, etc. [Citation2].
Elicitation is the most influential and practically achievable strategy for enhancing the production of desired secondary metabolites [Citation3]. NPs have the capacity to be used as effective elicitors in plant biotechnology and a number of studies have supported its possible role in enhancing the expression level of genes associated with the production of secondary metabolites [Citation4]. Among the metal oxides, ZnO-NPs have attracted considerable attention due to its multifarious properties. Various studies have reported its antibacterial and antifungal activities [Citation5–7]. However, there are many challenges and unresolved issues pertaining to the biological properties of NPs on terrestrial organisms, particularly plants [Citation8].
Silybum marianum (Milk thistle) is an annual herb belonging to Asteraceae which is considered as the world’s largest flowering plant family [Citation9]. Milk thistle is native to the Mediterranean region but now widespread throughout the globe [Citation10,Citation11]. This crop has lately become more significant in North America, Japan, Australia, Sub-Saharan Africa, parts of Europe, and also found in Khyber Pakhtunkhwa and Punjab provinces in Pakistan [Citation12,Citation13]. Silymarin is the principal component of S. marianum which comprise several flavonolignans like silybin (A and B), silychristine, isosilybin (A and B) and silydianin [Citation14]. The medicinal use of S. marianum has a comprehensive antiquity [Citation15]. Disorders of the spleen, liver and gallbladder have been treated by using the extracts from its leaves and flowers [Citation16]. Similarly, the seeds are used to treat different ailments including haemorrhage, peritonitis, gallstones, jaundice, bronchitis and varicose veins [Citation17]. The increasing global demand for silymarin is threatening the inadequate population of this species, however, a commercially invaluable plant like S. marianum have been overlooked for its metabolite enhancement and cultivation [Citation18].
Various studies have reported the mechanism and role of ZnO-NPs in plant growth and metabolism [Citation19]. The considerable antimicrobial effects and low cost of ZnO-NPs have enabled its application in food industry to reduce bacterial growth. In many plant enzymatic systems, zinc plays active catalytic or constructive role and its deficiency can lead to disorders in factors regulating plant growth [Citation20]. Owing to the fact, many researchers have investigated the critical role and distinctive properties of ZnO-NPs. ZnO-NPs enhanced the activities of antioxidant enzymes and the transcriptions of respective genes in tomato plant [Citation21]. Similarly, ZnO-NPs at low concentrations were found effective in alleviating various abiotic stresses and enhanced plant growth and development [Citation22]. Being non-toxic to normal cells, ZnO-NPs are progressively acknowledged due to their distinct activities against tumour cells [Citation23]. Keeping in view the importance of bioactive compounds derived from Milk thistle and the role of ZnO-NPs in influencing the growth and secondary metabolism in plants, the focus of the current study was to compare the influence of chemically-synthesised (NP1) and green-synthesised (NP2 and NP3) ZnO-NPs on seed germination frequency, root and shoot length, callus induction and biochemical profile of in-vitro-derived whole plant and callus cultures of S. marianum. Furthermore, the in-vitro antibacterial and anticancer activities of the extracts of whole plant and callus culture subjected to different ZnO-NPs were also evaluated. To the best of our knowledge, such study has not been reported yet.
Material and methods
Plant source and surface sterilization
Seeds of S. marianum were collected from the seed bank of Plant Cell Culture Lab, Department of Biotechnology, Quaid-i-Azam University, Pakistan. Protocol of Abbasi et al. [Citation9] for seed sterilisation was followed. Briefly, seeds were washed with sterile dH2O to remove dust and debris and then sterilised by soaking in 0.1% mercuric chloride for 1 min, followed by 3–4 times washing with sterile dH2O.
Preparation of C-ZNPs and G-ZNPs
C-ZNPs and G-ZNPs, as previously prepared by Nazir et al. [Citation24], were used in this study. For chemical synthesis, briefly, 2 M solution of NaOH was added drop-wise to 50 mL of 0.02 M solution of Zn(CH3CO2)2.2H2O and stirred constantly. When pH of the solution reached 12, white participates were formed which were then washed and dried.
Similarly, for green synthesis of ZnO-NPs, 1 mL of in-vitro whole plant and callus extracts, previously prepared by Nazir et al. [Citation24], were mixed separately in 50 mL of 0.02 M solution of Zn(CH3CO2)2.2H2O and kept under constant stirring. 2 M solution of NaOH was added drop-wise until the pH reached 12, followed by formation of white precipitates that were washed and dried. The callus was generated by Nazir et al., [Citation24] by using explant of stem, root and leaves of in-vitro whole plant at various concentrations of PGRs like BAP and NAA.
Seed germination protocol
C-ZNPs and G-ZNPs were added to MS-medium for seed germination. NPs were suspended in deionised water using sonication. 30 µg/mL suspension was considered as optimum and was made for each NPs by sonication in deionised water for 30 min [Citation1]. 3 mL of C-ZNPs and G-ZNPs suspension (30 µg/mL) were added separately to flasks containing 30 mL of MS media. After autoclaving and solidification of media, four set of seeds of S. marianum were inoculated in flasks containing medium with or without ZnO-NPs. Data on seed germination was recorded after 28 days of inoculation.
Seedling growth parameters
The in-vitro-derived plantlets treated with C-ZNPs and G-ZNPs were harvested after 4-weeks of inoculation. Different growth parameters like root and shoot length and FW and DW of plantlets were calculated. Root and shoot lengths were calculated with scale while FW was determined with a digital balance. These plantlets were then dried in incubator for DW calculation.
Establishment of callus culture
For callus induction, MS medium was supplemented with 30 g sucrose and 8 g Agar. pH of the medium was adjusted to 5.6–5.8. To obtain sufficient amount of callus, two different concentrations of PGRs: BAP and NAA (2BAP + 1NAA and 5BAP + 1NAA) were used, as this combination gave remarkable response for callus induction, as previously reported [Citation24], and 1TDZ was also added to the medium. C-ZNPs and G-ZNPs were separately added to the medium in concentration of 3 mL/30 mL per flask in order to determine their effects on callus establishment. Medium was boiled for 5 min to ensure proper mixing and then 30 mL medium was poured into flasks. The medium containing flasks were then sterilised. Three small pieces of stem explants (0.1 cm) from 28-days old seedlings were inoculated in each flask and kept in growth room with 25 °C and 16 h light/8h dark.
Preparation of extracts
Previously reported procedure was followed for preparation of extracts from in-vitro-derived whole plant and callus treated with C-ZNPs and G-ZNPS. Dried whole plant and callus was grounded to a fine powder. Then, 0.1 g of powder was mixed with 500 µL of methanol, vortexed and sonicated for 5 and 30 min, respectively. The extracts were then centrifuged for 15 min at 10,000 rpm at RT and supernatants were stored for further analysis [Citation25].
Total phenolic content (TPC)
Previous method [Citation26] was used for the determination of TPC using FC reagent. Stock solutions were prepared by 10-time diluted solution of FC reagent, 6% sodium carbonate solution and 4 mg/mL Gallic acid solution in methanol. 20 μL sample, 90 μL FC reagent and 90“μL sodium carbonate solution was added into each well of microplate. 25, 20, 15, 10 and 5 μg/mL of Gallic acid and 20 μL DMSO were used as positive and negative controls, respectively. OD was then determined at 630 nm. Total phenolic production (TPP) was calculated by formula:
Total flavonoid content (TFC)
TFC was also determined as described previously [Citation27]. Briefly, 10% aluminium chloride and 1 M“potassium acetate in dH2O and 4 mg/mL quercetin in methanol were used.”96-wells plate was employed for the preparation of reaction mixture containing 20 μL sample, 10 μL potassium acetate, 10 μL aluminium chloride and 160 μL dH2O. Reaction mixture was then incubated for 30 min.”40, 20, 10, 5, 2.5 μg/mL quercetin and 20 μL methanol were used as positive and negative controls, respectively. Absorbance was taken at 415 nm. Total flavonoid production (TFP) was calculated by formula:
Antioxidant activity
Antioxidant activity was also evaluated using previous protocol [Citation28]. Measurement of DPPH activity of samples was done in 96-wells plate by mixing 20 μL sample and 180“μL DPPH reagent. Final concentration (40, 20, 10 and 5 μg mL−1) of ascorbic acid was used as negative control. The reaction mixture was incubated for 1 h and absorbance was measured at 517 nm. Calculations were done using formula:
where AC is the solution absorbance when extract was added at a specific concentration and AS is the standard solution absorbance without test samples.
Total protein content
Method of Lowry et al. [Citation29] was followed here. Three reagents were used: (A) (1 g Na-K tartrate.4H2O, 50 g Na2CO3, 250 mL 1 N NaOH); (B) (2 g Na-K tartrate.4H2O, 1 g CuSO4.5H2O, 0.1 N NaOH); (C) (10-times diluted FC-reagent). Briefly, 40 µL of protein extract was taken and 36 µL of reagent A was added and then incubated for 10 min at 50 °C. After cooling the reaction mixture at 25 °C, 4 µL of reagent B was added and again incubated. Finally, 120 µL of reagent C was added after cooling the mixture at 25 °C. Absorbance was recorded at 650 nm. BSA was used as standard and calibration curve was made at series of 25, 50, 75, 100, 125, and 150 µg/mL.
Antibacterial activities
Well diffusion method was used to evaluate the antibacterial efficacy of in-vitro-derived plantlets and callus cultures grown on different PGRs, elicited with C-ZNPs and G-ZNPs. Briefly, plant material (20 μg mL−1) was dissolved in DMSO and vortexed daily for about 1 week. MDR gram-positive Listeria monocytogenes (ATCC-19115), Bacillus subtilis (ATCC-6633) and gram-negative Escherichia coli (ATCC-10536), Klebsiella pneumonia (clinical isolate) bacterial strains were considered. The ATCC cultures of test strains were refreshed on LB agar. A loop full of test strains was taken from the plate and transferred to 3 mL of sterile normal saline. The solutions were vortexed and compared to 0.5 McFarland standard solutions and the turbidity was adjusted according to that of McFarland. Sterile cotton swabs were dipped in bacterial solution and lawn was prepared on MHA plates by streaking. The plates were left for some time so that bacteria on lawn could establish their growth. Wells were made and 100 µL of each sample was transferred to each well. DMSO was used as negative while roxithromycine as positive controls. The plates were allowed for 2 h in biosafety cabinet and then transferred to incubator at 37 °C for 24 h and zone of inhibition was measured in mm.
Anticancer activities
Human liver carcinoma (HepG2, ATCC HB-8065) cells were cultured in DMEM supplemented with 10% FBS, 2 mM-glutamine, 1 mM Na-pyruvate, 100 µg/mL streptomycin and 100 U/mL penicillin at 37 °C in a humidified 5% CO2 atmosphere. Cells were harvested by trypsinization with 1 mL 0.5 mM trypsin/EDTA for 1 min at RT.
For evaluating cytotoxicity, pre-seeded cells (>90% viability; 12,000 cells/well) were incubated with 200 µg/mL (20 mg stock prepared in H2O; 1% of the stock = 200 µg/mL) with single dilution of callus extract treated with C-ZNPs and G-ZNPs for 24 h and cytotoxicity was measured by Sulforhodamine B (SRB) assay [Citation30]. Briefly, cultures were fixed by gently adding 50% pre-chilled trichloroacetic acid (25 µL/well) and incubated at 4 °C for 1 h. The plates were rinsed with deionised water at least 5-times and then air-dried. Samples were stained with”0.01% SRB solution (50 µL/well) for about 30 min at RT, washed with 1% acetic acid to completely remove the unincorporated dye and then air-dried. Untreated cells were taken as negative while doxorubicin (34 µM) as positive controls. Percent viability was calculated relative to NTC sample using formula:
where Abs (565) sample and Abs (565) NTC represent the OD at 565 nm for treated and untreated (control) samples, respectively. Abs (565) NP control and Abs (565) blank represent background OD and was measured in NPs and media samples only. HCEC cells were included as a normal control cell line.
shows the schematic illustration of the methodology and whole theme of the study.
Statistical analysis
All of the experiments were conducted in replicates and the data shown in here represent means ± SD. GraphPad Prism was used for statistical analysis and drawing of figures. One-way ANOVA followed by Tukey multiple comparison test was employed for finding significant differences (p < .05).
Results and discussion
Effects of C-ZNPs and G-ZNPs on seedlings growth parameters
Seeds grown on MS media containing C-ZNPs and G-ZNPs were used to evaluate their effects on different growth parameters of S. marianum seedlings. Medium without NPs was used as control (). Percent germination frequency for NP1 was low (39%), as compared to control (40%). Maximum germination frequency (65%) was recorded for NP3, while seeds treated with NP2 showed 45% germination frequency (). Narendhran et al. [Citation31] studied the comparative examination of Lantana aculeate-mediated and chemically-synthesised ZnO-NPs and reported its impact on seed germination of Sesamum indicum, revealing that L. aculeate-mediated ZnO-NPs enhanced seed germination and other growth parameters in S. indicum.
Figure 2. (A) Control; (B) Plantlet treated with NP3; (C) Plantlet treated with NP2; (D) Plantlets treated with NP1.
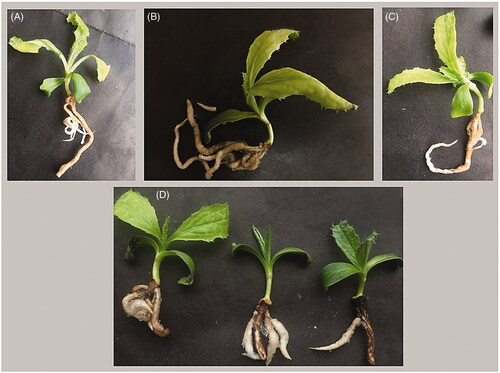
Table 1. Effect of C-ZNPs and G-ZNPs on seed germination frequency and plantlets mean root and shoot lengths of S. marianum.
Mean root length of control was recorded as 3.5 cm. NP1 showed inhibition of rooting (2.3 cm), which is in accordance with the results of Zafar et al. [Citation32], showing inhibition of seed germination and root length treated with metallic ZnO-NPs and the reason recorded for shorter root length was the direct interaction of NPs with root and its accumulation in root tissues. G-ZNPs showed enhanced rooting and maximum root length was recorded for NP3 (4.3 cm), whereas, NP2 slightly improved the root length (3.7 cm) (). Similar trend was observed in case of shoot length where maximum shoot length (5.3 cm) was recorded for NP3 (). Due to enhanced rooting and shooting of seedlings subjected to NP3, it is obvious that maximum FW (220.4 g L−1) and DW (21.23 g L−1) were obtained for NP3 as well (). These results are similar to the previous report showing the maximum shoot length, root length, FW and DW of sesame seedlings observed in biologically synthesised ZnO-NPs-treated plants [Citation33]. C-ZNPs showed adverse effects on root growth of several plant species like wheat, chickpea, radish, rape and rye grass [Citation34–36]. Zinc is an important micronutrient, crucial for growth and development of plant. ZnO-NPs have been shown to stimulate the biosynthesis of PGRs, especially auxins and gibberellins, which in turn promote the seedling growth as well as length and yield of the plant [Citation37]. Other studies suggest that ZnO-NPs play a key role in activation of enzymes like α- and β-amylase which stimulate metabolic reaction at germination stage [Citation38].
Table 2. Fresh and Dry weights of plantlets of S. marianum treated with different NPs.
Establishment of callus culture
C-ZNPs and G-ZNPs were applied to the media containing different combination of PGRs for callus induction, which affected the secondary metabolites of S. marianum. Nazir et al. [Citation24] and Abbasi et al. [Citation9] reported that the combination of BAP and NAA exhibit outstanding response for callus induction in S. marianum. Varied response of callus induction was observed with combinations of different concentrations of BAP and NAA [Citation39]. Similarly, Anwaar et al. [Citation40] also reported that green-synthesised CuO-NPs reveal positive results for callus induction in Oryza sativa.
Antioxidant activity
The antioxidant potential of S. marianum has been revealed previously, which has been attributed mainly to the presence of silymarin [Citation14,Citation41]. Several studies have reported the effects of NPs on the elicitation of plant secondary metabolites [Citation42–44]. Ag and Au-NPs were reported to enhance the secondary metabolites in P. vulgaris [Citation42]. DPPH is usually used to prove the antioxidants existence in a specific sample [Citation9]. The presence of NPs along with different PGRs influenced %DPPH activity in callus and in-vitro-derived plantlets of S. marianum (). There were negligible differences in %DPPH activity of treated plants in presence of PGRs. Yet, maximum DPPH activity (99%) was recorded in stem callus treated with NP3 in the presence of 2BAP + 1NAA. While in case of in-vitro-derived plantlets, highest DPPH activity (90%) was found for plantlets treated with NP2. FRSA showed positive correlation with TPC production i.e. highest DPPH and TPC was observed in plantlets treated with NP2. Previously, a linear correlation has been observed between TPC and antioxidant activities, which support the results of our study [Citation14].
Total phenolic and flavonoid contents
ZnO-NPs were found to be effective elicitors for phenolic content in S. marianum. In case of callus culture, maximum TPC (12.3 µg/mg DW) was recorded for stem-derived callus treated with NP3 in the presence of 2BAP + 1NAA. In case of in-vitro-derived plantlets, maximum TPC (2.5 mg L−1 DW) was found for plantlets treated with NP2 (). Similarly, TFC was also evaluated in the presence of different types of ZnO-NPs in combination with PGRs (). Maximum TFC (2.8 µg/mg DW) was recorded for root-derived callus established by the combination of 5BAP + 1NAA treated with NP3. In case of in-vitro-derived plantlets, highest TFC (5.5 µg/mg DW) was recorded for NP3. TFC production suggested that G-ZNPs effectively induce elicitation of secondary metabolites.
Figure 4. Combined effect of different concentrations of PGRs and NPs-treated plantlets’ samples on TPC in callus cultures in response to (a) 2BAP + 1NAA; (b) 5BAP + 1NAA; (c) 1TDZ; and (d) TPC in response to in-vitro whole plantlets.
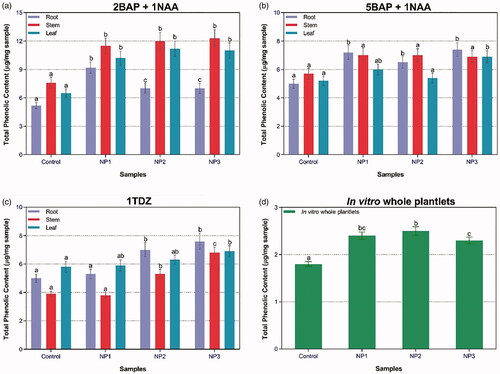
Figure 5. Combined effect of different concentrations of PGRs and NPs-treated plantlets’ samples on TFC in callus cultures in response to (a) 2BAP + 1NAA; (b) 5BAP + 1NAA; (c) 1TDZ; and (d) TFC in response to in-vitro whole plantlets.
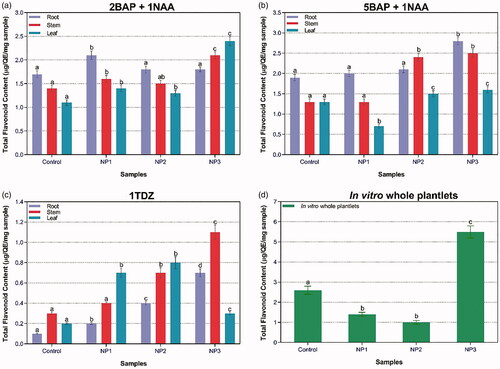
These results are in harmony with those reported by García-López et al. [Citation45] who suggested that ZnO-NPs in concentration from 100 to 500 ppm enhanced the accumulation of phenolic content in radicle of Capsicum annuum seedlings. Similarly, the application of 500 ppm ZnO-NPs elevated the TFC in Capsicum annuum radicle [Citation45]. Likewise, ZnO-NPs increased total flavonoid and phenolic contents in seedlings of Glycyrrhiza glabra [Citation46]. Total phenolic compounds were also found to be stimulated in potato plants, where the increase was much significant at 500 ppm ZnO-NPs [Citation47].
Total protein content
Maximum total protein content (265.32 BSAE/20g FW) was accumulated in root callus treated with NP3 on 5BAP + 1NAA (). The logic behind this elevated protein content in callus treated with NP3 might be cells releasing some of secondary proteins under stressful conditions to balance the oxidative damage employed as a result of stress. The effect of L. aculeate-mediated ZnO-NPs on Seasmum indicum grown in soil deficient in zinc was studied and it was found that the protein content in leaf greatly enhanced with the application of ZnO-NPs [Citation31]. Conversely, it was revealed that C-ZNPs decreased total protein content in lettuce, possibly due to toxic effect of these NPs on protein synthesis [Citation48]. Likewise, higher concentration of Zn also reduced protein concentration in Brassica juncea seedlings [Citation49].
Antibacterial efficacy
Assessment of antibacterial activities of extracts of C-ZNPs- and G-ZNPs-treated callus and in-vitro-derived plantlets was performed by agar well diffusion method against four MDR bacterial strains (). Root-derived callus treated with NP3 showed highest zone of inhibition (19 mm) against Klebsiella pneumonia that is somewhat close to roxithromycine (21 mm), followed by stem-derived callus treated with NP3 (18 mm) against Listeria monocytogenes (). Overall, the results revealed that G-ZNPs exhibit greater antibacterial potential. Gunalan et al. [Citation50] reported that green-synthesised ZnO-NPs show enhanced biocidal activity against various pathogens when compared to C-ZNPs. Due to chemical stability and high surface area of ZnO-NPs, they react with the cytoplasm of microorganisms, causing its destruction and ultimately leading to cell death [Citation51,Citation52]. Similarly, Nazir et al. [Citation24] investigated that G-ZNPs by callus extract from S. marianum possess greater activity against K. pneumonia and B. subtilis, as compared to C-ZNPs. Tiny size of NPs makes their entry easy into the mitochondria via several channels, provoking oxidative stress and cell demise by means of apoptosis.
Figure 7. Antibacterial activities of callus cultures and in-vitro plantlets grown on different PGRs and treated with C-ZNPs and G-ZNPs. From left to right i.e. (A1–A5) Antibacterial activity against K. pneumonia; (B1–B5) Antibacterial activity against B. subtilis; (C1–C5) Antibacterial activity against E. coli; (D1-D5) Antibacterial activity against L. monocytogenes. From top to bottom i.e. (A1, B1, C1, D1) Control of different bacterial strains; (A2, B2, C2, D2) 5BAP + 1NAA (1: NP1 stem; 2: NP2 root; 3: NP2 stem; 4: NP3 stem; 5: NP3 root); (A3, B3, C3, D3) 2BAP + 1NAA (6: NP1 root; 7: NP2 root; 8: NP2 stem; 9: NP3 root; 10: NP1 stem); (A4, B4, C4, D4) 1TDZ (11: NP1 root; 12: NP1 stem; 13: NP2 root; 14: NP2 stem; 15: NP3 root); (A5, B5, C5, D5) in-vitro plantlets (26: NP2; 27: C-ZNPs; 28: Control; 29: callus-synthesised NPs).
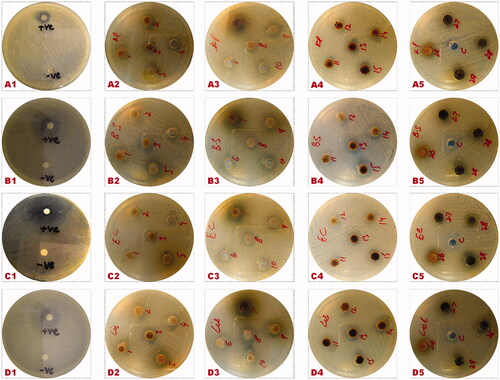
Table 3. Antibacterial zones of inhibition (mm) of callus and in-vitro plantlets-derived C-ZNPs and G-ZNPs.
Anticancer activities
Callus extract treated with C-ZNPs and G-ZNPs were further screened for cytotoxicity against human liver carcinoma (HepG2) cells (). Highest differential activity was observed for root-induced callus extract treated with G-ZNPs with a percent viability of 36% (IC50 = 279 µg/mL) in NP3 and 38% (IC50 = 279 µg/mL) in case of NP2. Percent cytotoxicity of 64% (IC50 = 176 µg/mL) was observed in root-induced callus under the influence of NP3 while NP2-induced cytotoxicity was recorded to be 62% (IC50 = 179 µg/mL). Lowest inhibition of cancer cells growth with highest viability of 46% (IC50 = 229 µg/mL) and 48% (IC50 = 223 µg/mL) was recorded in stem- and root-induced callus treated with C-ZNPs. Similarly, 42% (IC50 = 271 µg/mL) and 45% (IC50 = 259 µg/mL) cell viability were observed for stem-induced callus under the influence of NP3 and NP2, respectively.
Figure 8. Anticancer activity of S. marianum-derived callus treated with C-ZNPs and G-ZNPs against HepG2 cells. (A) Non-treated cells (Negative control); (B) Doxorubicin (Positive control); (C) NP1 Root; (D) NP1 Stem; (E) NP2 Root; (F) NP2 Stem; (G) NP3 Root; (H) NP3 Stem.
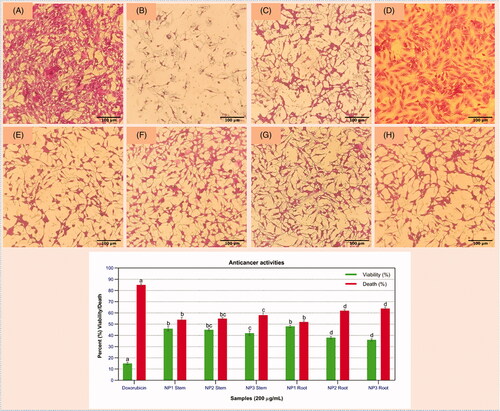
These results indicate that callus treated with G-ZNPs shows highest cytotoxic effect, as compared to C-ZNPs. According to the previous report, callus extracts showed promising anticancer potential as compared to the extract of wild leaf of Moringa oleifera against HeLa cells [Citation51]. Similarly, Lycopersicon esculentum and Alchornea cordifolia leaves extract-mediated ZnO-NPs also showed toxicity against HeLa cell lines [Citation52,Citation53], which support the results of our study. The underlying mechanism of ZnO-NPs in killing cancerous cells is not completely understood yet but various studies have tried to provide insight into the possible mechanisms [Citation54]. Based on the hypothetical concept, the photoactivation of ZnO-NPs is predicted to induce the greater levels of ROS generation via apoptosis which, if effectively targeted to cancer cells, will lead to their selective destruction [Citation55]. Another possible mechanism of ZnO killing human liver cancer cells is the generation of ROS and oxidative stress which has been attributed to small size and large surface area of ZnO-NPs that react with DNA molecule causing damage to both purines and pyrimidine, hence leading to cell death [Citation56].
Conclusion
Conclusively, the effect of G-ZNPs on seed germination, growth, callus induction and biochemical profile of S. marianum as well as antibacterial and anticancer assays is noteworthy. We found that callus-mediated ZnO-NPs were acting as growth promoter and showed enhanced biochemical profile as compared to C-ZNPs. Due to strong antimicrobial and anticancer behaviour of ZnO-NPs, it has a tremendous role in plant tissue culture and proved an excellent candidate to assure commercial biomedical applications. Our results revealed that G-ZNPs-elicited callus expressed much stronger inhibitory effect on bacterial and cancerous cells as compared to C-ZNPs. In-vitro cultures elicited with G-ZNPs could be an alternative strategy in the field of antibacterial and anticancer therapeutics. Yet, our expertise of the basic mechanisms that proclaim such processes is limited and should be investigated further.
Disclosure statement
No potential conflict of interest was reported by the author(s).
References
- Savithramma N, Ankanna S, Bhumi G. Effect of nanoparticles on seed germination and seedling growth of Boswellia ovalifoliolata an endemic and endangered medicinal tree taxon. Nano Vision. 2012;2(1):2.
- Zaka M, Abbasi BH. Effects of bimetallic nanoparticles on seed germination frequency and biochemical characterisation of Eruca sativa. IET Nanobiotechnol. 2017;11(3):255–260.
- Naik PM, Al-Khayri JM. Abiotic and biotic elicitors–role in secondary metabolites production through in vitro culture of medicinal plants. In: Naik PM, Al–Khayri JM, editors. Abiotic and biotic stress in plants-recent advances and future perspectives. London: Intech; 2016. p. 247–277.
- Ghasemi B, Hosseini R, Nayeri FD. Effects of cobalt nanoparticles on artemisinin production and gene expression in Artemisia annua. Turk J Bot. 2015;39(5):769–777.
- Sirelkhatim A, Mahmud S, Seeni A, et al. Review on zinc oxide nanoparticles: antibacterial activity and toxicity mechanism. Nanomicro Lett. 2015;7(3):219–242.
- Bhuyan T, Mishra K, Khanuja M, et al. Biosynthesis of zinc oxide nanoparticles from Azadirachta indica for antibacterial and photocatalytic applications. Mater Sci Semicond Process. 2015;32:55–61.
- Miri A, Mahdinejad N, Ebrahimy O, et al. Zinc oxide nanoparticles: biosynthesis, characterization, antifungal and cytotoxic activity. Mater Sci Eng C. 2019;104:109981.
- El‐Temsah YS, Joner EJ. Impact of Fe and Ag nanoparticles on seed germination and differences in bioavailability during exposure in aqueous suspension and soil. Environ Toxicol. 2012;27(1):42–49.
- Abbasi BH, Khan MA, Mahmood T, et al. Shoot regeneration and free-radical scavenging activity in Silybum marianum L. Plant Cell Tiss Organ Cult. 2010;101(3):371–376.
- Karkanis A, Bilalis D, Efthimiadou A. Cultivation of milk thistle (Silybum marianum L. Gaertn.), a medicinal weed. Ind Crops Prod. 2011;34(1):825–830.
- Kren V, Walterova D. Silybin and silymarin-new effects and applications. Biomed Pap Med Fac Univ Palacky Olomouc Czech Repub. 2005;149(1):29–41.
- Zheljazkov VD, Zhalnov I, Nedkov NK. Herbicides for weed control in blessed thistle (Silybum marianum) 1. Weed Technol. 2006;20(4):1030–1034.
- Shah SMM, Khan FA, Shah SMH. Evaluation of phytochemicals and antimicrobial activity of white and blue capitulum and whole plant of Silybum marianum. World Appl Sci J. 2011;12(8):1139–1144.
- Younas M, Drouet S, Nadeem M, et al. Differential accumulation of silymarin induced by exposure of Silybum marianum L. callus cultures to several spectres of monochromatic lights. J Photochem Photobiol B. 2018;184:61–70.
- Ball KR, Kowdley KV. A review of Silybum marianum (milk thistle) as a treatment for alcoholic liver disease. J Clin Gastroenterol. 2005;39(6):520–528.
- Rainone F. Milk thistle. Am Fam Physician. 2005;72(7):1285–1288.
- Sonnenbichler J, Scalera F, Sonnenbichler I, et al. Stimulatory effects of silibinin and silicristin from the milk thistle Silybum marianum on kidney cells. J Pharmacol Exp Ther. 1999;290(3):1375–1383.
- Khan MA, Abbasi BH, Shinwari ZK. Thidiazuron enhanced regeneration and silymarin content in Silybum marianum L. Pak J Bot. 2014;46(1):185–190.
- Auld DS. Zinc coordination sphere in biochemical zinc sites. Biometals. 2001;14:271–313.
- Taheri M, Qarache HA, Qarache AA, et al. The effects of zinc-oxide nanoparticles on growth parameters of corn (SC704). STEM Fellowship J. 2015;1(2):17–20.
- Wang X, Li Q, Pei Z, et al. Effects of zinc oxide nanoparticles on the growth, photosynthetic traits, and antioxidative enzymes in tomato plants. Biol Plant. 2018;62(4):801–808.
- Mahajan P, Dhoke S, Khanna A, et al. Effect of nano-ZnO on growth of mung bean (Vigna radiata) and chickpea (Cicer arietinum) seedlings using plant agar method. Appl Biol Res. 2011;13(2):54–61.
- Soliman AS, El-Feky SA, Darwish E. Alleviation of salt stress on Moringa peregrina using foliar application of nanofertilizers. J Hortic For. 2015;7(2):36–47.
- Nazir S, Zaka M, Adil M, et al. Synthesis, characterisation and bactericidal effect of ZnO nanoparticles via chemical and bio-assisted (Silybum marianum in vitro plantlets and callus extract) methods: a comparative study. IET Nanobiotechnol. 2018;12(5):604–608.
- Ahmed D, Arshad MA, Asghar MN, et al. Antioxidant and free radical scavenging potential of Otostegia limbata. Asian J Chem. 2010;22(6):4524.
- Singleton VL, Rossi JA. Colorimetry of total phenolics with phosphomolybdic-phosphotungstic acid reagents. Am J Enol Viticult. 1965;16(3):144–158.
- Zia-Ul-Haq M, Shahid SA, Ahmad S, et al. Antioxidant potential of various parts of Ferula assafoetida L. J Med Plant Res. 2012;6(16):3254–3258.
- Amarowicz R, Pegg R, Rahimi-Moghaddam P, et al. Free-radical scavenging capacity and antioxidant activity of selected plant species from the Canadian prairies. Food Chem. 2004;84(4):551–562.
- Lowry OH, Rosebrough NJ, Farr AL, et al. Protein measurement with the Folin phenol reagent. J Biol Chem. 1951;193(1):265–275.
- Arooj S, Nazir S, Nadhman A, et al. Novel ZnO:Ag nanocomposites induce significant oxidative stress in human fibroblast malignant melanoma (Ht144) cells. Beilstein J Nanotechnol. 2015;6(1):570–582.
- Narendhran S, Rajiv P, Sivaraj R. Influence of zinc oxide nanoparticles on growth of Sesamum indicum L. in zinc deficient soil. Int J Pharm Pharm Sci. 2016;8(3):365–371.
- Zafar H, Ali A, Ali JS, et al. Effect of ZnO nanoparticles on Brassica nigra seedlings and stem explants: growth dynamics and antioxidative response. Front Plant Sci. 2016;7:535.
- Premanathan M, Karthikeyan K, Jeyasubramanian K, et al. Selective toxicity of ZnO nanoparticles toward Gram-positive bacteria and cancer cells by apoptosis through lipid peroxidation. Nanomedicine: NBM. 2011;7(2):184–192.
- Lin D, Xing B. Phytotoxicity of nanoparticles: inhibition of seed germination and root growth. Environ Pollut. 2007;150(2):243–250.
- Burman U, Saini M, Kumar P. Effect of zinc oxide nanoparticles on growth and antioxidant system of chickpea seedlings. Toxicol Environ Chem. 2013;95(4):605–612.
- Prakash MG, Chung IM. Determination of zinc oxide nanoparticles toxicity in root growth in wheat (Triticum aestivum L.) seedlings. Acta Biol Hung. 2016;67(3):286–296.
- El-Kereti MA, El-Feky SA, Khater MS, et al. ZnO nanofertilizer and He Ne laser irradiation for promoting growth and yield of sweet basil plant. Recent Pat Food Nutr Agric. 2013;5(3):169–181.
- Israel García-López J, Lira-Saldivar RH, Zavala-García F, et al. Effects of zinc oxide nanoparticles on growth and antioxidant enzymes of Capsicum chinense. Toxicol Environ Chem. 2018;100(5–7):560–572.
- Lv Y-W, Wamg R-J, Lv Y, et al. In vitro propagation of Silybum marianum (l.) Gaertn. and genetic fidelity assessment of micropropagated plants. Pak J Bot. 2017;49(2):673–680.
- Anwaar S, Maqbool Q, Jabeen N, et al. The effect of green synthesized CuO nanoparticles on callogenesis and regeneration of Oryza sativa L. Front Plant Sci. 2016;7:1330.
- Surai PF. Silymarin as a natural antioxidant: an overview of the current evidence and perspectives. Antioxidants. 2015;4(1):204–247.
- Fazal H, Abbasi BH, Ahmad N, et al. Elicitation of medicinally important antioxidant secondary metabolites with silver and gold nanoparticles in callus cultures of Prunella vulgaris L. Appl Biochem Biotechnol. 2016;180(6):1076–1092.
- Javed R, Yucesan B, Zia M, et al. Elicitation of secondary metabolites in callus cultures of Stevia rebaudiana Bertoni grown under ZnO and CuO nanoparticles stress. Sugar Tech. 2018;20(2):194–201.
- Chung I-M, Rekha K, Rajakumar G, et al. Elicitation of silver nanoparticles enhanced the secondary metabolites and pharmacological activities in cell suspension cultures of bitter gourd. 3 Biotech. 2018;8(10):412.
- García-López JI, Zavala-García F, Olivares-Sáenz E, et al. Zinc oxide nanoparticles boosts phenolic compounds and antioxidant activity of Capsicum annuum L. during germination. Agronomy. 2018;8(10):215.
- Oloumi H, Soltaninejad R, Baghizadeh A. The comparative effects of nano and bulk size particles of CuO and ZnO on glycyrrhizin and phenolic compounds contents in Glycyrrhiza glabra L. seedlings. Ind J Plant Physiol. 2015;20(2):157–161.
- Raigond P, Raigond B, Kaundal B, et al. Effect of zinc nanoparticles on antioxidative system of potato plants. JEB. 2017;38(3):435–439.
- Farshian S, Khara J, Malekzadeh P. Influence of arbuscular mycorrhizal fungus (Glomus etunicatum) with lettuce plants under zinc toxicity in nutrient solution. Pak J Biol Sci. 2007;10(14):2363–2367.
- Sharma RK, Agrawal M, Marshall F. Heavy metal contamination of soil and vegetables in suburban areas of Varanasi, India. Ecotoxicol Environ Saf. 2007;66(2):258–266.
- Gunalan S, Sivaraj R, Rajendran V. Green synthesized ZnO nanoparticles against bacterial and fungal pathogens. Prog Nat Sci. 2012;22(6):693–700.
- Yousef JM, Danial EN. In vitro antibacterial activity and minimum inhibitory concentration of zinc oxide and nano-particle zinc oxide against pathogenic strains. J Health Sci. 2012;2(4):38–42.
- Santos-Filho SD. Erythrocyte membrane and hemolysis: effects of natural products. Int J Life Sci. 2016;9(3):28.
- Aula S, Lakkireddy S, Swamy A, et al. Biological interactions in vitro of zinc oxide nanoparticles of different characteristics. Mater Res Express. 2014;1(3):035041.
- Jafarain A, Asghari G, Ghassami E. Evaluation of cytotoxicity of Moringa oleifera Lam. callus and leaf extracts on Hela cells. Adv Biomed Res. 2014;3:194.
- Vijayakumar S, Vaseeharan B, Sudhakaran R, et al. Bioinspired zinc oxide nanoparticles using Lycopersicon esculentum for antimicrobial and anticancer applications. J Clust Sci. 2019;30(6):1465–1479.
- Sharma V, Anderson D, Dhawan A. Zinc oxide nanoparticles induce oxidative DNA damage and ROS-triggered mitochondria mediated apoptosis in human liver cells (HepG2). Apoptosis. 2012;17(8):852–870.