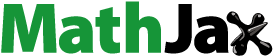
Abstract
Nano-elicitation is one among the prioritised strategies considered globally for sustainable and uniform production of industrially important medicinal compounds. Ocimum basilicum (Thai basil), a renowned medicinal species is a reservoir of commercially vital metabolites and proved for its health assuring effects in cancer, diabetes, microbial and cardiovascular diseases. However, its consumption and industrial demand raised intent to divert towards better alternates for ensuring sustainable production of medicinal compounds. Herein, we investigated the comparative potential of metal oxide [copper oxide (CuO) and manganese oxide (MnO)] nanoparticles to elicit the biosynthesis of bioactive metabolites and antioxidative capacity of O.basilicum callus cultures. Results showed that callus grown on MS media supplemented with 10 mg/L CuO-NPs resulted in the highest biomass accumulation (FW: 172.8 g/L, DW: 16.7 g/L), phenolic contents (TPC: 27.5 mg/g DW), and flavonoid contents (TFC: 9.1 mg/g DW) along with antioxidant activities (DPPH: 94%, ABTS: 881 μM TEAC, FRAP: 386 μM TEAC) compared with MnO-NPs and control. Likewise, the Superoxide dismutase (SOD: 1.28 nM/min/mg FW) and Peroxidase (POD: 0.48 nM/min/mg FW) activities were also recorded maximum in CuO-NPs elicited cultures than MnO-NPs and control. Moreover, the HPLC results showed that rosmarinic acid (11.4 mg/g DW), chicoric acid (16.6 mg/g DW), eugenol (0.21 mg/g DW) was found optimum in cultures at 10 mg/L CuO-NPs. Overall, it can be concluded that CuO nanoparticles can be effectively used as a elicitor for biosynthesis of metabolites in callus cultures of O. basilicum (Thai basil). The study is indeed a contribution to the field that will help decoding the mechanism of action of CuO NPs. However, further molecular investigations are needed to fully develop understanding about the metabolic potential of O. bascillicum and scalling up this protocol for bulkup production of bioactive compounds.
Introduction
Ocimum basilicum (Thai basil), is a novel herb from the Lamiaceae family [Citation1]. Due to its profuse background uses in food, cosmetics and pharmaceutical industry, it is regarded as an utmost commercially utilised plant [Citation1,Citation2]. It has been used in folk medicine for inflammation, cancers, diabetes, intestinal and cardiovascular problems. Besides, it is widely utilised as antimicrobial, anti-convulsant and for sedative purposes [Citation3]. These inclusive features of the plant are attributed to various groups of bioactive metabolites mainly include essential oils, phenols, and flavonoids [Citation4–6]. The most prevalent compounds in O. basilicum are rosmarinic acid, chicoric acid, caffeic acid, euginol and anthocyanins that have momentous commercial value and health attributes [Citation7]. Different metabolic aspects involved in plant growth and protection from harmful agents are also influenced by these bioactive compounds thoroughly distributed in plant [Citation8]. Usually, the bioactive metabolites are generated by plants in response to any exterior or interior stimulus but their production is confined or sunken [Citation9]. Likely, the low availability and immense variability of metabolites, and improper downstream processing are the major complications in developing commercial products of O. basilicum [Citation10]. Due to an overgrowing demand of the valuable phyto-derived medicinal compounds an equivalent substitute to bulky harvesting from cultivated or wild plants, is required to ensure their sustainable production. Accordingly, several techniques and procedures have been developed for commercial production [Citation11].
Plant in vitro culture technology is a better feasible alternative approach that provides smooth, eco-friendly and cost efficient extraction methodologies over chemical synthesis or direct extraction from wild plants [Citation8,Citation12]. For instance, several alterations or modifications to such systems made to further enhance the biosynthesis of compounds because an output capacity of such systems is intrinsically limited [Citation13]. Until now, elicitation proved to be more promising to effectively enhance the phytochemicals’ levels in in vitro cultures of plants [Citation10]. Elements of different nature are used as stimulating agents that elicit key metabolite biosynthesis in in vitro cultures. The phytochemicals production is elicited or initiated by inducing defense system activated as stress response in plants [Citation8]. Mechanistically, the generation of reactive oxygen species is increased in stress conditions and the plant's antioxidant system gets switched via enzymatic and non-enzymatic pathways [Citation14]. There is a broad range of elicitors classified into two groups as biotic and abiotic; comprise of biotic and abiotic components [Citation9]. Multiple abiotic and biotic elicitors have been used in in vitro cultures of various medicinal plants to improve secondary metabolites contents [Citation15,Citation16]. Furthermore, the type, specificity and concentration of elicitor along age of culture and exposure period are certain parameters that can affect elicitation positively or negatively [Citation9].
Recent improvements and extensive usage of nanomaterial have radicalised its biotechnological applications. Nanoparticles ranging in size from 1 to 100 nm employed usually on commercial levels have diverse applications in several fields. Nanoparticles (NPs) have been emanated as peculiar and effectual abiotic elicitors used to achieve improved yields of compounds in plants [Citation17,Citation13]. It is known that, based on the concentration, NPs can have both beneficial and damaging effects on plants. It also supports agriculture by alleviating diseases, support plant growth as nano-nutrients and mainly as insecticides [Citation18,Citation19]. Earlier, the metal oxide nanoparticles got attention for their improved chemical and physiological features, green nature, cost efficient and range of oxidation states along technological attributes [Citation20]. Manganese is heavily occurring as 12th most ordinary element on earth an vital micronutrient in plant and animals [Citation21]. In plants, it involves in regulation of growth, as structural part of proteins complexes and certain metabolic pathways [Citation22]. Likewise, Copper oxide nanoparticles possess several advantages including cost effective and accessibility in contrast to gold or silver NPs along other physical and chemicals features [Citation23]. It has been widely reported for application as fungicides and pesticides [Citation24,Citation25], coatings, antioxidant and fertilizers [Citation23]. Although, copper and manganese as micronutrients influence plant growth and physio-biochemical profiles. Previously, only a few studies have reported the use of CuO and MnO NPs as an elicitor [Citation26–28]. Besides, if not the nanoparticles, the source metallic compounds were mostly used, which may pave the basis for the use of NPs as elicitors [Citation29,Citation30].
Because of overexploitation from innate environment and increasing industrial demand, O. basilicum needs attention for a sustainable and smooth production. Till now, there is no previous report existing on MnO and CuO nanoparticles based elicitation in callus cultures of O. basilicum. Therefore, the current study aimed to investigate the effects of several treatments of both CuO and MnO nanoparticles on biomass and secondary metabolite production in callus cultures of O. basilicum. The study also aimed to determine the anti-oxidants capacity of elicited cultures and to quantify bioactive compounds via HPLC.
Materials and methods
Plant material and seeds germination
Seeds of O. basilicum were collected from National Agriculture Research Centre (NARC) Islamabad, Pakistan. Seeds were sterilised and inoculated by following the earlier described method [Citation31]. Briefly, the viable seeds were first identified by Incubation Dry Separation Floating method [Citation32] and washed three times with autoclaved distilled water. The seeds were then surface sterilised by treating with 0.1% HgCl2 and 70% ethanol for a respective duration of 2 min each. Finally, the seeds were washed three times with autoclaved distilled water to remove any impurities and surface dried with filter papers (autoclaved). The growth media (MS0; Murashige and Skoog basal medium; Phytotechnology Laboratories, USA) fortified with 30 g/L sucrose (carbon source) and 8 g/L agar (solidifying agents) were used for seed germiantion. Before inoculation, the media was sterilised by autoclaving at 121 °C for 20 min after pH adjustment (5.5–5.7) with NaOH (0.1 N) and HCL (1.0 N). The flasks having seeds inoculated on media were placed in continuous growth conditions (temperature 25 ± 2 °C and photoperiod (16/8 h light/dark with a light intensity of 40–50 μmol m−2 s−1 (Philips TLD 35 Florescent lamps) in the growth room.
Establishment of callus culture and nanoparticles treatment
The leaf explant (0.5 cm2) was used from in vitro germinated plantlets (28 days old) and innocluated on hormonal media. The BAP (5 mg/L and NAA (1 mg/L) was used in media to induce optimum callogenesis as optimised previously [Citation33]. Prior autoclaving, pH of the growth media was adjusted to 5.2–5.6 with 0.1 N NaOH and 0.1 N HCL. The flasks having leaf explants inoculated on hormonal media were maintained properly at 25 ± 1 °C under 16/8 h (Light/Dark) photoperiod with light intensity of 40–50 μmol m−2 s−1 (Philips TLD 35 Florescent lamps).
For experimental part, chemically synthesised CuO-NPs (20–50 nm) and MnO-NPs (20–30 nm) were provided by department of chemistry [unpublished data], Quaid-i-Azam University synthesised by co-precipitation method [Citation22] and thermal decomposition method [Citation23] respectively. To investigate metallic oxide nanoparticles effects, callus (0.1 g) was sub-cultured on same hormonal media supplemented with various concentrations of CuO NPs (1, 5, 10, 25, 50, 100 mg/L) and MnO NPs (1, 5, 10, 25, 50, 100 mg/L). All the treatments were applied in three experimental replicates and repeated twice.
Subsequently, the cultures were moved to the growth room (25 ± 2 °C) and maintained under 16 L/8D h light period. Callus without treatment of NPs was used as control. The experiment was conducted in triplicate and finally harvested after 4 weeks for biomass determination and further analysis.
Biomass determination and sample extraction
The callus cultures were harvested, and leftover media were removed with filter paper. The callus cultures were weighed for fresh weight analysis while for dry weight analysis the cultures were placed in an incubator to dry. For phytochemical analysis, calli powder was used to prepare the methanolic extracts by following the protocol of [Citation33]. Briefly, 10 mL methanol (99.9%) was poured in 100 mg of ground samples and kept for 24 h at room temperature on rotatory shaker. The reaction mixture was sonicated for 20 min and vertexed for 30 min at 5 min intervals. The procedure was repeated twice, and the mixture was finally centrifuged at 13,000 rpm for 15 min to collect the supernatant. The supernatant was syring filtered and stored at 4 °C for further analysis.
Determination of phtochemical contents
For the determination of total phenolic contents, the protocols of Slinkard and Singleton (1977) were used with slight changes [Citation34]. Concisely, 20 μL of the sample, 90 μL of Folin-Ciocalteu reagent and 90 μL of sodium carbonate were mixed. The reaction mixture was incubated for 1.5 h at room temperature and 200 ul was then added into microplate wells (96 well plates). The absorbance of reaction mixture was measured at 630 nm with UV/VIS-DAD spectrophotometer (Halo DR-20, UV-VIS spectrophotometer, Dynamica Ltd, Victoria, Australia). Positive and negative controls were used by employing gallic acid (1 mg/mL) and methanol (20 μL), respectively. The calibration curve (0–50 μg/mL, R2 = 0.968) was plotted by using gallic acid as standard and the TPC was expressed as gallic acid equivalents (GAE)/g of DW. Total phenolic production (TPP) was measured by using the following formula and estimated in mg gallic acid/L.
Total flavonoids contents were determined by employing AlCl3 colorimetric method reported by Chang et al. [Citation35], with minor modifications [Citation35]. Breifly, 20 μL sample extract was taken and mixed with 10 μL potassium acetate, 10 μL aluminium trichloride solution and 160 μL distilled water were added. The reaction mixture was kept for 39 min at room temperature for proper incubation. The absorbance was recorded ar 415 nm by using UV/VIS-DAD spectrophotometer The calibration curve (0–40 μg/mL, R2 = 0.998) was drawn for standard (quercetin). The TFC was expressed as quercetin equivalents (QE)/g of dry weight while Total flavonoid production (TFP) was expressed in mg quercetin/l and calculated as;
Quantification of metabolites by HPLC
The detection and estimation of valuable phenolic and flavonoid compounds of O.basilicum were carried out by using Varian HPLC system formed with autosampler (Prostar 410), photodiode array detector (Prostar 335), and degassed (Metachem Degasit) by following the previously described method [Citation36]. Separation was done at 35 °C using an Hypersil PEP 300 C18 column (250 × 4.6 mm, 5 mm, Thermo Scientific, Illkirch, France). The acetonitrile (solvent A) and 0.1% (v/v) formic acid acidified ultrapure water (solvent B) were utilised in mobile phase. The composition of the mobile phase varied during a 1-h run according to a linear gradient ranging from a 5:95 (v/v) to 100:0 (v/v) mixture of solvents A and B, respectively at a flow rate of 0. Detection was completed at 320 and 520 nm. Compounds were identified by comparing with their standards purchased from sigma aldrech and calibration curves were used for quantification of bioactive metabolites by following the previously described method. The data acquisition was carried out with MassLynx 4.0 software. The phytochemical compounds were quanitified against 5-point calibration curve (R2 > 0.999) and retention time of reference standards. The analysis for each sample was made in triplicates and repeated twice, results expressed as mg/g DW .
Determination of superoxide dismutase and peroxidase activity
The method described by Giannoplolitis and Ries [Citation37] was employed for the determination of superoxide dismutase activity. The enzymatic activities were performed by using extracts derived quickly from fresh callus samples. Shortly, 100 mg fresh calli were homogenised in 1 mL of 50 mM Potassium phosphate buffer (pH: 7) having 1% PVP. The mixture was incubated and then centrifuged at 14,000 rpm for 30 mintues. The supernatants were taken in sterile tubes for analysis.
The Super oxide dismutase activity was performed by mixing 20 μL of nitroblue tetrazolium (0.75 mM), 20 μL of methionine (130 mM), 78 μL of phosphate buffer (50 mM, pH7), 2 μL of riboflavin (0.02 mM), 20 μL of ethylenediaminetetraacetic acid (EDTA) (1 mM) and 60 μL fresh sample extract. The reaction mixture was then incubated for 7 min and absorbance was taken at 660 nm by using Micro-plate reader (Thermo Scientific Multiskan GO).
Peroxidase (POD) activity was performed as reported by [Citation38]. The reaction mixture was prepared by mixing 40 μL of potassium phosphate buffer (50 mM, pH 7), 20 μL guaiacol (1 M), 20 μL of H2O2 (27.5 M), 20 μL of fresh sample extract, and 100 μL of dH2O then incubated for the 20 s and absorbance was taken at 470 nm with micro-plate reader (Thermo Scientific Multiskan GO). The enzymatic activities were calculated by using following formula;
where A denotes Absorbance, E = Extinction coefficient (6.39 mM−1 cm−1), L = Length of wall (0.25 cm), C = enzyme concentration (value calculated in nM/min/mg FW) and FW represent fresh weight of sample.
Determination of antioxidant activity
DPPH scavenging activity
Determination of antioxidant activity was done by following the protocol of Shah et al. [Citation39]. Briefly, 20 μL extract was added with 180 μL DPPH reagent and kept for 1 h incubation period at room temperature in the dark. Absorbance was measured at 517 nm. The scavenging activity of the free radical was calculated using the following formula:
where AE represents sample absorbance at 517 nm and AD represents DPPH solution absorbance.
Ferric reducing antioxidant power (FRAP) assay
To determine the FRAP potential of cultures the protocol of Jan et al. [Citation40] was used with slight improvements. The 10 µL of sample extract was used to mix it with FRAP solution (190 µL) followed by an incubation period of 15 min at room temperature. Absorbance was recorded at the wavelength (630 nm) using a Microplate Reader (BioTek ELX800, Bio-Tek Instruments). The antioxidant capacity of samples was expressed in terms of TEAC (Trolox C equivalent antioxidant capacity).
ABTS assay
For the ABTS (2,2-azinobis-3ethylbenzthiazoline-6-sulphonic acid) assay, the Faisal et al. protocol was used [Citation41]. (7 mM) ABTS salt was added in the same proportion with (2.45 mM) potassium persulfate and placed for 16 h in the dark. 10 µL sample mixture was mixed with 190 µL ABTS solution and kept in the dark at room temperature for 15 min. Absorbance was measured at 734 nm. Antioxidant capacity was presented as TEAC.
Cellular antioxidant assay
For this assay, Nazir et al. protocol was followed with slight modifications [Citation1]. The fluorescent dye DHR123 (Dihydrorhodamine123) in cells was used for estimating the production of reactive nitrogen and oxygen species, by oxidising the products of the respective fluorescent. Yeast cells overnight incubated with DMSO (control cell) and with a sample which further was washed twice with PBS. In the presence of 0.4 μM DHR123, the sediments were immersed in PBS, and incubation was done at 30 °C for 10 min in the dark then signal detection was done by fluorometer after washing it with PBS.
Statistical analysis
All experiments were performed in triplicates and conductd twice. Graph Pad Prism Windows, v 5.0 was used for statistical analysis, and analytical data were evaluated as mean ± standard deviation using Excel 2018 (Microsoft, Redmond, WA, USA).
Results and discussion
Effects of CuO-NPs and MnO-NPs on biomass accumulation
Nanoparticles induce various physiological and biochemical changes in cells that can affect plants either positively or negatively based on the type, size and concentration of nanoparticles interacting with plant cells [Citation42,Citation43]. Usually, lower concentrations of NPs stimulate plant growth and secondary metabolite production [Citation44]. In current study, CuO and MnO nanoaprticles were applied in callus cultures of O. basicilicum to investigate effects on cells proliferation and determine biomass. The results revealed an overall growth promoting effect of metallic oxide nanoparticles in a dose dependent way. Maximum biomass (FW: 172.8 g/L and DW: 16.7 g/L) was recorded at 10 mg/L CuO-NPs, while the minimum biomass (FW: 86.8 g/L and DW: 7.0 g/L) was noted at 100 mg/L CuO-NPs. Wherein, MnO-NPs induced maximum possible biomass accumulation (FW: 139.6 g/L and DW: 13.5 g/L) and minimum biomass (FW: 72.6 g/L and DW: 6.9 g/L) in cultures grown at 25 mg/L and 100 mg/L MnO Nps, respectively ().
Figure 1. Determination of Biomass (A) Dry weight and B) Fresh weight analysis, in callus cultures of Thai basil grown on MS media supplemented with different concentrations of CuO-NPs and MgO-NPs.
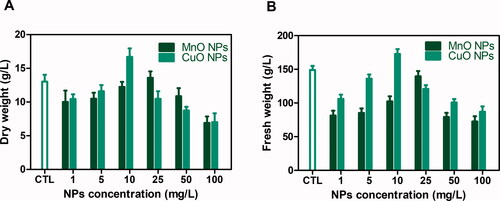
Most of studies regarding NPs accumulation, toxicity, absorption and biotransformation are still contradictory and therefore, the mechanism of action of nanoparticles behind plant growth is not yet specified [Citation45]. However, it can be hypothesised that more growth induction and callus proliferation by CuO nanoparticles can be due to Copper role in physio-biochemical processes including hormone signalling, metabolism, and electron transport chain and part of regulatory protiens [Citation46]. Moreover, increased NPs concentrations showed a negative effect on biomass production. This might be due to toxicity and decreased adaptability of plant cells to NPs high concentrations. Mechanistically, the oxidative stress induction generates toxic free radicals, that increases with increase in nanoparticles stress. The increase beyond threshold levels causes reduction in various physiological parameters in callus and radicals scavenging products that retard overall growth of cells [Citation47]. Our findings are also in accordance with previous reports where they observed that higher concentrations have inhibitory effects while lower concentrations stimulate plant growth [Citation48,Citation49].
Effects of CuO and MnO nanoparticles on phytochemical contents
Production of non-enzymatic antioxidants (phenolics and flavonoids) in the protection mechanism against ROS is considered as a prime benchmark [Citation50]. Their accumulation varies in different environments and can be improved by adding elicitors in growth medium. In current study, CuO and MnO nanoparticles applied as elicitors in callus cultures were investigated in terms of phytochemicals accumulation. O.basilicumResults showed a similar trend for TPC, with a progressive increase as the CuO-NPs concentration reached 10 mg/L before reducing above this critical value. Comparatively, maximum phenolic contents (TPC: 27.5 mg/g DW) were found at 10 mg/L of CuO-NP, while maximum level of TPC (10.5 mg/g DW) was noted for 25 mg/L in case of MnO-NPs than control ().
Figure 2. (A) Total phenolic contents and (B) Total flavonoid contents in callus cultures of Thai basil grown on MS media supplemented with different concentrations of CuO-NPs and MgO-NPs.
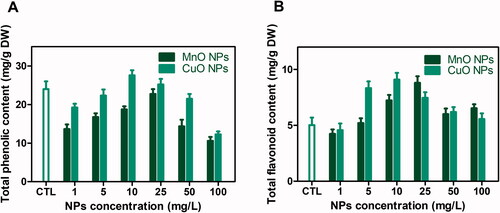
Similarly, maximum value for TFC (9.1 mg/g DW) was found in response to 10 mg/L CuO-NPs followed by 25 mg/L MnO-NPs (TFC: 8.8 mg/g DW). The possible reason for increased phenolics and flavonoids production is ROS generation by NPs that start complex reactions and affect cell metabolic processes. Once entered inside cells, NPs interact with cellular organelles, induce genetic alterations and oxidative stress [Citation51]. Under such conditions, the plant defense mechanism generates phenolics and flavonoids as natural antioxidants [Citation52]. Our results are in accordance with previous reports in which CuO-NP enhanced TPC and TFC levels [Citation47,Citation53]. Similar effects of different metal nanoparticles on phenolics and flavonoids production in various medicinal plant species have also been reported previously by Chung et al. [Citation54].
Effects of CuO-NPs and MnO-NPs on metabolites production
Various bioactive compounds exist in plants' defense systems, which are released when the plant is facing stress or unfavourable conditions. These compounds include low molecular weight phytochemicals including phenolics and alkaloids to defend the plant survival [Citation55]. Exposure of O.basilicum callus culture to CuO-NPs and MnO-NPs induced significant changes in its polyphenols profile. The HPLC analysis showed that CuO-NPs resulted in higher production of rosmarinic acid (11.4 mg/g DW), chicoric acid (16.6 mg/g DW), cyanidin (2.02 mg/g DW), peonidin (1.18 mg/g DW), (eugenol (0.21 mg/g DW), methyl eugenol (0.17 µg/g DW) chavicol (0.30 µg/g DW) and methyl chavicol (0.17 µg/g DW) in the callus at 10 mg/L concentration compared to control ( and ). The mechanism behind the enhanced production of metabolites is not yet clear, however different studies have reported CuO-NPs as promising abiotic elicitors in in vitro cultures for the production of important bioactive compounds [Citation56,Citation57]. In callus cultures, the biomass at higher concentrations of CuO-NPs and MnO-NPs has reduced, because of the toxicity at higher levels that denature macromolecules and disturb metabolic processes in such a way that the growth and development of plant cells are retarded [Citation58]. In the case of MnO-NPs, a significant increase in caffeic acid production (0.20 mg/g DW) was observed at a moderate concentration compared to control. However, increased NPs concentration resulted in decreased accumulation of secondary metabolites. These results can be interpreted as a protective effect induced by the accessibility of NPs against ROS. High levels of MnO-NPs in the callus culture lead to biochemical changes, cell death, and inhibitory effects on growth [Citation59]. The increased level of NPs reduced the ability of cells to withstand ROS and dramatically decreased the callus biomass [Citation60,Citation61]. Our results are also in accordance with the previous report where CuO-NPs at 10 mg/L concentration enhanced the production of secondary metabolites in Stevia rebaudiana [Citation47].
Table 1. Comparative effect of CuO-NPs and MgO-NPs on production of secondary metabolites in callus culture of Thai basil.
Table 2. Comparative effect of CuO-NPs and MgO-NPs on essential oil components in callus culture of Thai basil.
Effects of CuO-NPs and MnO-NPs on antioxidant enzymes activity
Plants usualy produce stress enzymes such as SOD, POD, Ascorbate peroxidase and Catalses to cope with oxidative stress and scavenge free radicals generated by biotic and abiotic stress [Citation62]. Superoxide dismutase (SOD) and peroxidase (POD) are isozymes, distinguished by different physical and chemical properties and sequences of amino acids but they have the same catalytic reactions. . The knowledge about CuO and MnO nanoparticels role or effects on these enzymes is scares. Herein, the cultures were also investigated for SOD and POD enzyme activities, exposed to CuO-NPs and MnO-NPs. The optimum enzyme activities (SOD: 1.28 nM/min/mg FW and POD: 0.48 nM/min/mg FW) were recorded in cultures grown at 10 mg/L CuO-NPs. Cultures in response to 25 mg/L MnO-NPs were also found with significant enzymatic activities (). However, with increasing concentrations of NPs, a sudden drop in enzymatic potential was observed. This sudden decrease in enzymatic activities could be the result of a decline in the capacity of cells to withstand the effect of ROS [Citation63]. Plants also evolved a series of antioxidative defense processes that depend on the non-enzymatic and enzymatic mechanisms [Citation64]. In plants, ROS act as a messenger for signalling in several physiological processes but cannot be fully scavenged. To cope with oxidative stress, plants can activate a set of antioxidant enzymes to detoxify excess ROS production [Citation65]. SOD catalyses superoxide anion's dismutation to H2O2 and its stimulation may protect plants from oxidative harm [Citation66]. Likewise, the key enzyme involved in H2O2 reduction is POD. The oxidative stress caused by NPs can be counterbalanced by the activation of enzymatic antioxidants. In the future, these improved enzymatic and non-enzymatic reactions may also serve as a means for enhancing productivity in O.basilicum. Moreover, our findings are in accordance with previous reports where NPs application activated antioxidant enzymes in several plant species [Citation67].
Effects of CuO and MnO NPs on antioxidant activities
Environmental stress on plants drives abrupt changes in metabolic pathways resulting in the generation of reactive oxygen species (ROS) that comprises nitric oxide (NO), hydrogen peroxide, hydroxyl radical, and superoxide anions capable of damaging plant cells, lipids, proteins, and DNA [Citation68]. Plants respond to protect themselves from their negative effects via several phytochemicals including glutathione, phenolics, and flavonoids as antioxidants [Citation69]. So here, the study involved investigation of CuO-NPs and MnO-NPs effects on O.basilicumO.basilicum callus cultures by employing three distinct antioxidant assays i.e. FRAP, ABTS, and DPPH. The FRAP represents the mechanism of electron transfer (ET), ABTS follows the mechanism of hydrogen atom transfer (HAT) both demonstrated as TEAC (Trolox C equivalent antioxidant capacity µM) while DPPH is coupled HAT and ET based assay indicated as a percentage (%).
The results showed that the highest antioxidant activities (ABTS: 881.4 µM TEAC, DPPH: 94.7% and FRAP: 386 µM TEAC) were found in cultures exposed to 10 mg/L CuO-NPs while callus elicited with MnO-NPs (25 mg/L) were found with optimum activities (ABTS: 749 µM TEAC, DPPH: 91.1% and FRAP: 428 µM TEAC) as compard with control. The results were found to be descending by increasing the concentrations of both types of nanoparticles (). Our findings are strongly supported by earlier reports where CuO-NPs increased the antioxidant activities in Stevia rebaudiana shoots and callus culture [Citation47,Citation56]. Antioxidant activity findings disclosed a significant relationship with secondary metabolites. CuO-NPs considerably improved phytochemical levels in O.basilicum callus culture that eventually increased its antioxidative capacity. A positive correlation between the phenolic profile and antioxidant ability was also noted, which is consistent with the results of previous reports [Citation70, Citation71].
Table 3. Comparative effect of CuO-NPs and MgO-NPs on in vitro and in vivo antioxidant activities in callus culture of Thai basil.
Effects of CuO and MnO NPs on in vivo antioxidant activity
The imbalance between reactive nitrogen/oxygen species (RNS/ROS) and the defense system cause oxidative stress that leads to the oxidation of macromolecules. Natural antioxidants like flavonoids can effectively mitigate the adverse effects of excessive production of ROS and RNS [Citation70,Citation71]. In vivo, antioxidant activity was also assessed through the capacity to inhibit the production of ROS and RNS using a cellular antioxidant assay. Among different treatments of NPs applied, the callus cultures under CuO-NPs (10 mg/L) and MnO-NPs (25 mg/L) showed the highest cellular antioxidative effect (52.4% and 50.7% respectively) compared with control ().
Conclusion
Oscimum bacsillicum is a vital medicinal plant species with multitude of biological attributes. It is the need of an hour to develop biotechnological approaches for sustainable production of its biomass and bioactive compounds. Herein, for the first time we examined nano-elicitation with metallic oxide nanoparticles and results were found significantly improved. Overall, CuO NPs were observed to enhance biomass proliferation and increased phytochemicals contents. The in vitro antioxidant activities and cellular antioxidant activities were also found to be maximum in cultures elicited with metallic oxide nanoparticles as compared with control. The findings proposed positive role of CuO-NPs as an effective elicitor for key metabolite accumulation in callus cultures. This research will make a significant contribution in understanding the critical impact of NPs on biochemical parameters in addition to the large-scale production of valuable metabolites in O. bascillicum (O.basilicum) in vitro cultures. However, further studies are needed to fully understand the molecular mechanism of nanoparticles in cellular development and metabolism in plants.
Author contributions
SN performed experiments, compiled data, and prepared manuscripts. HJ helped in manuscript writing and formal analysis. TK, HA, and BM assisted in experimental work and formatting. BHA conceived the idea, supervised research, and reviewed the manuscript. CH performed HPLC and interpreted HPLC data. All authors reviewed the manuscript.
Disclosure statement
No potential conflict of interest was reported by the author(s).
Data availability statement
The data is available with the 1st author and corresponding author of this article
References
- Nazir S, Jan H, Tungmunnithum D, et al. Callus culture of Thai basil is an effective biological system for the production of antioxidants. Molecules. 2020;25(20):4859.
- Vani SR, Cheng S, Chuah C. Comparative study of volatile compounds from genus Ocimum. Am J Appl Sci. 2009;6(3):523.
- Nguyen PM, Kwee EM, Niemeyer ED. Potassium rate alters the antioxidant capacity and phenolic concentration of basil (Ocimum basilicum L.) leaves. Food Chem. 2010;123(4):1235–1241.
- Lee J, Scagel CF. Chicoric acid found in basil (Ocimum basilicum L.) leaves. Food Chem. 2009;115(2):650–656.
- Peng Y, Sun Q, Park Y. The bioactive effects of chicoric acid as a functional food ingredient. J Med Food. 2019;22(7):645–652.
- Selvi MT, Thirugnanasampandan R, Sundarammal S. Antioxidant and cytotoxic activities of essential oil of Ocimum canum sims. from India. Journal of Saudi Chemical Society. 2015;19(1):97–100.
- Nadeem M, Abbasi BH, Younas M, et al. LED-enhanced biosynthesis of biologically active ingredients in callus cultures of Ocimum basilicum. J Photochem Photobiol B. 2019;190:172–178.
- Abbasi BH, Khan T, Khurshid R, et al. UV-C mediated accumulation of pharmacologically significant phytochemicals under light regimes in in vitro culture of Fagonia indica (L.). Sci Rep. 2021;11(1):679–616.
- Khan T, Khan T, Hano C, et al. Effects of chitosan and salicylic acid on the production of pharmacologically attractive secondary metabolites in callus cultures of Fagonia indica. Ind Crops Prod. 2019;129:525–535.
- Khan T, Ullah MA, Garros L, et al. Synergistic effects of melatonin and distinct spectral lights for enhanced production of anti-cancerous compounds in callus cultures of Fagonia indica. J Photochem Photobiol. B. 2019;190:163–171.
- Azmir J, Zaidul ISM, Rahman M, et al. Techniques for extraction of bioactive compounds from plant materials: a review. J Food Eng. 2013;117(4):426–436.
- Davies KM, Deroles SC. Prospects for the use of plant cell cultures in food biotechnology. Curr Opin Biotechnol. 2014;26:133–140.
- Nazir M, Tungmunnithum D, Bose S, et al. Differential production of phenylpropanoid metabolites in callus cultures of Ocimum basilicum L. with distinct in vitro antioxidant activities and in vivo protective effects against UV stress. J Agric Food Chem. 2019;67(7):1847–1859.
- Molazem D, Azimi J. Proline reaction, peroxide activity and antioxiant enzymes in varieties of maize (Zea mays L.) under different levels of salinity. Aust J Basic Appl Sci. 2011;5(10):1248–1253.
- Hughes KW. In vitro ecology: exogenous factors affecting growth and morphogenesis in plant culture systems. Environ Exp Bot. 1981;21(3–4):281–288.
- Narayani M, Srivastava S. Elicitation: a stimulation of stress in in vitro plant cell/tissue cultures for enhancement of secondary metabolite production. Phytochem Rev. 2017;16(6):1227–1252.
- Namdeo A. Plant cell elicitation for production of secondary metabolites: a review. Pharmacogn Rev. 2007;1(1):69–79.
- de la Rosa G, García-Castañeda C, Vázquez-Núñez E, et al. Physiological and biochemical response of plants to engineered NMs: implications on future design. Plant Physiol Biochem. 2017;110:226–235.
- Fakruddin M, Hossain Z, Afroz H. Prospects and applications of nanobiotechnology: a medical perspective. J Nanobiotechnol. 2012;10(1):1–8.
- Jassal V, Shanker U, Gahlot S, et al. Sapindus mukorossi mediated green synthesis of some manganese oxide nanoparticles interaction with aromatic amines. Appl Phys A. 2016;122(4):271.
- Veeramani H, Aruguete D, Monsegue N, et al. Low-temperature green synthesis of multivalent manganese oxide nanowires. ACS Sustainable Chem Eng. 2013;1(9):1070–1074.
- Hoseinpour V, Ghaemi N. Green synthesis of manganese nanoparticles: applications and future perspective–a review. J Photochem Photobiol, B. 2018;189:234–243.
- Siddiqi KS, Husen A. Current status of plant metabolite-based fabrication of copper/copper oxide nanoparticles and their applications: a review. Biomater Res. 2020;24:11–15.
- Borkow G, Gabbay J. Copper, an ancient remedy returning to fight microbial, fungal and viral infections. Curr Chem Biol. 2009;3(3):272–278.
- Zheng X, Xu C, Tomokiyo Y, et al. Observation of charge stripes in cupric oxide. Phys Rev Lett. 2000;85(24):5170–5173.
- Cook C, Kostidou A, Vardaka E, et al. Effects of copper on the growth, photosynthesis and nutrient concentrations of phaseolus plants. Photosynt. 1997;34(2):179–193.
- Safavi K. 2012. Evaluation of using nanomaterial in tissue culture media and biological activity. Paper presented at the 2nd International Conference on Ecological, Environmental and Biological Sciences (EEBS'2012).
- Tian H, Ghorbanpour M, Kariman K. Manganese oxide nanoparticle-induced changes in growth, redox reactions and elicitation of antioxidant metabolites in deadly nightshade (Atropa belladonna L.). Ind Crops Prod. 2018;126:403–414.
- Chung I-M, Rekha K, Venkidasamy B, et al. Effect of copper oxide nanoparticles on the physiology, bioactive molecules, and transcriptional changes in Brassica rapa ssp. rapa seedlings. Water Air Soil Pollut. 2019;230(2):48.
- Osman NI, Sidik NJ, Awal A. Efficient enhancement of gallic acid accumulation in cell suspension cultures of Barringtonia racemosa L. by elicitation. Plant Cell Tiss Organ Cult. 2018;135(2):203–212.
- Mahendran D, Pb KK, Sreeramanan S, et al. Enhanced biosynthesis of colchicine and thiocolchicoside contents in cell suspension cultures of Gloriosa superba L. exposed to ethylene inhibitor and elicitors. Ind Crops Prod. 2018;120:123–130.
- Usman H, Ullah MA, Jan H, et al. Interactive effects of wide-spectrum monochromatic lights on phytochemical production, antioxidant and biological activities of Solanum xanthocarpum callus cultures. Molecules. 2020;25(9):2201.
- Shah M, Jan H, Drouet S, et al. Chitosan elicitation impacts flavonolignan biosynthesis in Silybum marianum (L.) gaertn cell suspension and enhances antioxidant and anti-Inflammatory activities of cell extracts. Molecules. 2021;26(4):791.
- Singleton VL, Rossi JA. Colorimetry of total phenolics with phosphomolybdic-phosphotungstic acid reagents. Am J Enol Vitic. 1965;16(3):144–158.
- Chang C-C, Yang M-H, Wen H-M, et al. Estimation of total flavonoid content in propolis by two complementary colorimetric methods. J Food Drug Anal. 2020;10(3):178–182.
- Jan H, Shah M, Usman H, et al. Biogenic synthesis and characterization of antimicrobial and anti-parasitic zinc oxide (ZnO) nanoparticles using aqueous extracts of the Himalayan columbine (Aquilegia pubiflora). Front Mater. 2020;7:249.
- Giannopolitis CN, Ries SK. Superoxide dismutases: I. Occurrence in higher plants. Plant Physiol. 1977;59(2):309–314.
- Sadiq MB, Hanpithakpong W, Tarning J, et al. Screening of phytochemicals and in vitro evaluation of antibacterial and antioxidant activities of leaves, pods and bark extracts of Acacia nilotica (L.) del. Ind Crops Prod. 2015;77:873–882.
- Shah M, Nawaz S, Jan H, et al. Synthesis of bio-mediated silver nanoparticles from Silybum marianum and their biological and clinical activities. Mater Sci Eng C. 2020;112:110889.
- Jan H, Khan MA, Usman H, et al. The Aquilegia pubiflora (Himalayan columbine) mediated synthesis of nanoceria for diverse biomedical applications. RSC Adv. 2020;10(33):19219–19231.
- Faisal S, Khan MA, Jan H, et al. Edible mushroom (Flammulina velutipes) as biosource for silver nanoparticles: from synthesis to diverse biomedical and environmental applications. Nanotechnology. 2020;32(6):065101.
- Navarro E, Baun A, Behra R, et al. Environmental behavior and ecotoxicity of engineered nanoparticles to algae, plants, and fungi. Ecotoxicology. 2008;17(5):372–386.
- Thuesombat P, Hannongbua S, Akasit S, et al. Effect of silver nanoparticles on rice (Oryza sativa L. cv. KDML 105) seed germination and seedling growth. Ecotoxicol Environ Saf. 2014;104:302–309.
- Farag MA, Al-Mahdy DA, Meyer A, et al. Metabolomics reveals biotic and abiotic elicitor effects on the soft coral Sarcophyton ehrenbergi terpenoid content. Sci Rep. 2017;7(1):648–611.
- Ma X, Geiser-Lee J, Deng Y, et al. Interactions between engineered nanoparticles (ENPs) and plants: phytotoxicity, uptake and accumulation. Sci Total Environ. 2010;408(16):3053–3061.
- Paramo LA, Feregrino-Pérez AA, Guevara R, et al. Nanoparticles in agroindustry: applications, toxicity, challenges, and trends. Nanomaterials. 2020;10(9):1654.
- Javed R, Yucesan B, Zia M, et al. Elicitation of secondary metabolites in callus cultures of Stevia rebaudiana Bertoni grown under ZnO and CuO nanoparticles stress. Sugar Tech. 2018;20(2):194–201.
- Jimenez-Garcia SN, Vazquez-Cruz MA, Guevara-Gonzalez RG, et al. Current approaches for enhanced expression of secondary metabolites as bioactive compounds in plants for agronomic and human health purposes–a review. Pol J Food Nutr Sci. 2013;63(2):67–78.
- Ovais M, Khalil AT, Islam NU, et al. Role of plant phytochemicals and microbial enzymes in biosynthesis of metallic nanoparticles. Appl Microbiol Biotechnol. 2018;102(16):6799–6814.
- Patcharawan S. 2013. Antioxidant and anti-inflammatory cytokine activity by extracts from hoan-ngoc (Pseuderanthemum palatiferum (Nees) Radlk.). Suranari: School of Environmental Biology Institute of Science Suranaree University of Technology.
- Hatami M, Naghdi Badi H, Ghorbanpour M. Nano-elicitation of secondary pharmaceutical metabolites in plant cells: a review. J Med Plants. 2019;18(71):6–36.
- Berni R, Luyckx M, Xu X, et al. Reactive oxygen species and heavy metal stress in plants: impact on the cell wall and secondary metabolism. Environ Exp Bot. 2019;161:98–106.
- Zafar H, Ali A, Zia M. CuO nanoparticles inhibited root growth from Brassica nigra seedlings but induced root from stem and leaf explants. Appl Biochem Biotechnol. 2017;181(1):365–378.
- Chung I-M, Rekha K, Rajakumar G, et al. Production of bioactive compounds and gene expression alterations in hairy root cultures of Chinese cabbage elicited by copper oxide nanoparticles. Plant Cell Tiss Organ Cult. 2018;134(1):95–106.
- Septembre-Malaterre A, Remize F, Poucheret P. Fruits and vegetables, as a source of nutritional compounds and phytochemicals: changes in bioactive compounds during lactic fermentation. Food Res Int. 2018;104:86–99.
- Aminizadeh M, Riahi MA, Mohammadi M. 2016. Nano-Metal oxides induced sulforaphane production and peroxidase activity in seedlings of Lepidium draba (Brassicaceae). Prog Biol Sci. 2016;6(1):75–83.
- Mulabagal V, Tsay H-S. Plant cell cultures-an alternative and efficient source for the production of biologically important secondary metabolites. Int J Appl Sci Eng. 2004;2(1):29–48.
- Javed R, Mohamed A, Yücesan B, et al. CuO nanoparticles significantly influence in vitro culture, steviol glycosides, and antioxidant activities of Stevia rebaudiana Bertoni. Plant Cell Tiss Organ Cult. 2017;131(3):611–620.
- Pérez-Labrada F, Hernández-Hernández H, López-Pérez MC, et al. Nanoparticles in plants: morphophysiological, biochemical, and molecular responses. In: Tripathi DK, editor. Plant life under changing environment. London: Elsevier; 2020. p. 289–322.
- Ghormade V, Deshpande MV, Paknikar KM. Perspectives for nano-biotechnology enabled protection and nutrition of plants. Biotechnol Adv. 2011;29(6):792–803.
- Stadtman ER, Oliver CN. Metal-catalyzed oxidation of proteins: physiological consequences. J Biol Chem. 1991;266(4):2005–2008.
- Jiang W, Yang L, He Y, et al. Genome-wide identification and transcriptional expression analysis of superoxide dismutase (SOD) family in wheat (Triticum aestivum). PeerJ. 2019;7:e8062.
- Zaeem A, Drouet S, Anjum S, et al. Effects of biogenic zinc oxide nanoparticles on growth and oxidative stress response in flax seedlings vs. in vitro cultures: a comparative analysis. Biomolecules. 2020;10(6):918.
- Spengler A, Wanninger L, Pflugmacher S. Oxidative stress mediated toxicity of TiO2 nanoparticles after a concentration and time dependent exposure of the aquatic Macrophyte hydrilla verticillata. Aquat Toxicol. 2017;190:32–39.
- Sharma P, Jha AB, Dubey RS, et al. Reactive oxygen species, oxidative damage, and antioxidative defense mechanism in plants under stressful conditions. J Bot. 2012;2012:1–26.
- Quan LJ, Zhang B, Shi WW, et al. Hydrogen peroxide in plants: a versatile molecule of the reactive oxygen species network. J Integr Plant Biol. 2008;50(1):2–18.
- Ma C, Liu H, Guo H, et al. Defense mechanisms and nutrient displacement in Arabidopsis thaliana upon exposure to CeO2 and in 2O3 nanoparticles. Environ Sci Nano. 2016;3(6):1369–1379.
- Durzan DJ, Pedroso MC. Nitric oxide and reactive nitrogen oxide species in plants. Biotechnol Genetic Eng Rev. 2002;19(1):293–338.
- Kasote DM, Katyare SS, Hegde MV, et al. Significance of antioxidant potential of plants and its relevance to therapeutic applications. Int J Biol Sci. 2015;11(8):982–991.
- Djeridane A, Yousfi M, Nadjemi B, et al. Antioxidant activity of some Algerian medicinal plants extracts containing phenolic compounds. Food Chem. 2006;97(4):654–660.
- Kim D-O, Chun OK, Kim YJ, et al. Quantification of polyphenolics and their antioxidant capacity in fresh plums. J Agric Food Chem. 2003;51(22):6509–6515.