Abstract
Gusperimus is an anti-inflammatory drug that has shown to be effective in managing autoimmunity and preventing graft rejection. This is unstable and easily broken down into cytotoxic components. We encapsulated gusperimus binding it covalently to squalene obtaining squalene-gusperimus nanoparticles (Sq-GusNPs). These nanoparticles enhanced the immunosuppressive effect of gusperimus in both mouse macrophages and T cells. The half-maximal inhibitory concentration in macrophages was 9-fold lower for Sq-GusNPs compared with the free drug. The anti-inflammatory effect of the Sq-GusNPs was maintained over time without cytotoxicity. By studying nanoparticles uptake by cells with flow cytometry, we demonstrated that Sq-GusNPs are endocytosed by macrophages after binding to low-density lipoprotein receptors (LDLR). In presence of cathepsin B or D release of gusperimus is increased demonstrating the participation of proteases in the release process. Our approach may allow the application of Sq-GusNPs for effective management of inflammatory disorders including autoimmunity and graft rejection.
Introduction
Gusperimus is an immunosuppressive drug that has shown to be effective for the treatment of transplant rejection and autoimmunity [Citation1] through its immunomodulatory effect on both innate and adaptative arms of the immune system [Citation1–4]. However, the immunosuppressive activity of gusperimus is hindered by stability issues due to its high hydrophilicity [Citation5]. This results in loss of activity [Citation2] but also cytotoxicity as undesired by-products are generated during breakdown [Citation6]. To overcome this, we encapsulated gusperimus using the squalenoylation platform in which the drug is conjugated covalently to squalene [Citation7]. The obtained squalene-gusperimus bioconjugate was able to form nanoparticles (NPs) in water without the use of any other excipient and these NPs were shown to be stable and exert a sustained anti-inflammatory effect over time [Citation2]. The Sq-GusNPs had a diameter between ∼150 and 200 nm, a zeta potential of ∼ −34 mV, and a high drug loading capacity of ∼50% [Citation2].
Nanoencapsulation using squalene has been shown to improve the uptake of different therapeutics [Citation7]. This has been suggested to be mediated by the property of squalene to interact with cholesterol-rich proteins, such as low-density lipoproteins (LDL) in the circulation [Citation7]. These circulating lipoproteins can act as indirect natural carriers for Sq-GusNPs to facilitate spreading in the human body and target specific cell types [Citation8]. Especially cells that need to proliferate have a high requirement for cholesterol and express low-density lipoprotein receptors (LDLR) to enhance uptake of LDL. Thus, proliferating immune cells, such as macrophages [Citation9] but also some types of cancer cells [Citation8] can be specific targets for drugs-containing squalene NPs. Therefore squalene nanoencapsulation has many advantages for anti-inflammatory drugs, such as gusperimus which will enhance not only stability but also uptake by target immune cells to exert anti-inflammatory effects [Citation3].
Despite increased stabilization by nanoencapsulation, it is unknown how the Sq-GusNPs exert their therapeutic effect after being internalized. The internalization of squalene-derived NPs has been suggested to occur via low-density lipoprotein receptor (LDLR) mediated endocytosis [Citation7,Citation10,Citation11] by the interaction of LDL with the NPs surface [Citation10]. Once into the cell, the bioconjugate as the entity forming the squalene-derived NPs is suggested to be cleaved by proteases after which the active drug is released exerting the therapeutic effect due to its prodrug nature [Citation8,Citation11,Citation12]. As it is unknown how the Sq-GusNPs are taken up in cells and how their biological activity is maintained, we performed the current study by investigating the immunosuppressive effect, the internalization, and the release mechanism from Sq-GusNPs in vitro. As described in the scheme of , first, the immunosuppressive effect was investigated in cells of the innate (RAW-264.7 macrophages) and the adaptative (CTLL-2 cytotoxic T cells) arms of the immune system. Subsequently, the internalization mechanism was studied following uptake of Nile red labelled Sq-GusNPs by RAW-264.7 macrophages after induction of LDLR expression which was corroborated by quantitative reverse-transcription polymerase chain reaction (qRT-PCR) using different isotypes of the LDLR. Finally, we performed in vitro release studies by quantifying gusperimus using fluorescamine mimicking the acidic conditions found in endolysosomal compartments. Our data demonstrate that Sq-GusNPs are internalized via endocytosis after LDLR binding and that they serve as a prodrug that is activated by catalytic cleavage caused by proteases, such as cathepsins B and D.
Materials and methods
Materials
Gusperimus was obtained from Nordic Pharma SAS (Paris, France). Squalene gusperimus (Sq-Gus) bioconjugate was synthesized in our laboratory through squalene transformation until obtention of its carboxylic acid derivative and consequent reaction with gusperimus as previously reported () [Citation2]. Mouse macrophages LRRK2 parental RAW-264.7 (ATCC® SC-6003™) and cytotoxic T lymphocytes CTLL2 (ATCC® TIB-214™) were obtained from American Type Culture Collection (ATCC) (Manassas, VA, USA). DMEM supplemented with 4.5 g/L glucose and L-Glutamine was purchased from LONZA (Walkersville, MD, USA). RPMI 1640 supplemented with 25 mM Hepes & L-Glutamine was purchased from LONZA (Verviers, Belgium). L-glutamine, gentamycin, sodium pyruvate, alamarBlue™ reagent, TRIzol™ reagent, and SuperScript II Reverse Transcriptase were purchased from Life Technologies Europe B.V. (Bleiswijk, Netherlands). Foetal calf serum (FCS), lipoprotein deficient serum from foetal calf (LPDS), lipopolysaccharides (LPS) from Escherichia coli O111:B4 purified by phenol extraction, Nile red technical grade, cathepsin D from human liver (250 units/mg), cathepsin B from bovine spleen (≥10 units/mg), fluorescamine ≥98% (TLC), FastStart Universal SYBR Green Master (Rox), 3,3′-dihexyloxacarbocyanine iodide (DiOC6), propidium iodide (PI), and primers of mouse low-density lipoprotein receptor (LDLR) were purchased from Sigma–Aldrich Chemie N.V. (Zwijndrecht, Netherlands). T-STIM culture supplement rat with CON A was purchased from Discovery Labware, Inc. (Two Oak Park, Bedford, MA, USA). ELISA DuoSet kits for mouse IL-10 and TNF-α were purchased from R&D Systems (Abingdon, UK). Float-A-Lyzer G2 dialysis device 3.5–5 kD was purchased from Repligen Europe B.V. (Breda, Netherlands).
Nanoparticle preparation
Sq-GusNPs preparation
Sq-GusNPs were prepared through nanoprecipitation as previously reported [Citation2]. Briefly, the Sq-Gus bioconjugate was dissolved in ethanol at a concentration of 2 mg/mL. Later 380 µL of the solution was added drop by drop to 1 ml of deionized water under stirring (500 rpm) for 10 min after which ethanol was evaporated using the concentrator SpeedVac SPD2010 (Thermo Fisher Scientific, Bleiswijk, Netherlands). This resulted in an aqueous suspension of pure NPs with a size of 182.5 ± 62.2 nm as measured by dynamic light scattering (DLS) with the particle size analyzer NICOMP 380 ZLS (Particle Sizing Systems, Inc., Santa Barbara, CA, USA).
Nile red labelled Sq-GusNPs preparation
Labelled Sq-GusNPs were obtained as previously reported [Citation2]. Briefly, an ethanolic solution of Nile Red at a concentration of 0.1 mg/mL was prepared. From this 380 µL was taken and ethanol was evaporated using the concentrator SpeedVac SPD2010 to obtain dried crystals of Nile Red. Subsequently, 380 µL of Sq-Gus bioconjugate dissolved in ethanol at a concentration of 2 mg/mL was added to the dried Nile Red crystals, vortexed, and NPs obtained as described in the previous section (Sq-GusNPs preparation). The aqueous suspension of labelled Sq-GusNPs was filtered through a 0.22 µm filter to separate the Nile red precipitate after ethanol evaporation obtaining a translucid suspension of labelled Sq-GusNPs. The prepared NPs had a size of 154.9 ± 47.0 nm as measured by DLS with the particle size analyzer NICOMP 380 ZLS.
Cell culture
RAW-264.7 cells were cultured in DMEM supplemented with 4.5 g/L glucose and L-Glutamine, 10% FCS heat-inactivated, L-glutamine (2 mM), and gentamicin (60 µg/mL). CTLL-2 cells were cultured in RPMI 1640 supplemented with 25 mM Hepes & L-Glutamine, 10% FCS heat-inactivated, L-glutamine (2 mM), sodium pyruvate (1 mM), and 10% T-STIM with Con A. Cells were cultured in an incubator at 37 °C and 5% CO2.
Cell viability with alamarBlue™
To determine cell viability with alamarBlue™ the reagent was diluted in a culture medium (10% v/v). After treatment, cells were washed with PBS buffer and incubated for 4 h with 0.5 ml of the diluted reagent. After this fluorescence intensity or absorbance was determined. Fluorescence was measured with the plate reader CLARIOstarPlus (BMG LABTECH, Offenburg, Germany) Ex/Em 560/590 nm. Absorbance was measured in a microplate spectrophotometer Benchmark Plus BIO-RAD (Bio-Rad Laboratories B.V., Veenendaal, Netherlands) at 570 nm with correction at 600 nm. Fluorescence or absorbance obtained from cells without any treatment was used as a control.
Determination of half-maximal inhibitory concentration (IC50) of cell proliferation in macrophages
Mouse RAW-264.7 macrophages at a density of 5 × 104 cells/well were seeded in 12-well plates. After 24 h cells were washed with PBS buffer and exposed to different concentrations of either free gusperimus or Sq-GusNPs in a total volume of 1 ml of culture medium. Following incubation for 48 h, cells were washed with PBS, and viability was determined using alamarBlue™. The dose-response curves were fitted using the GraphPad Prism 9 software (GraphPad Software Inc., USA) to establish the IC50 for cell proliferation. Results were expressed as a percentage of the control.
ELISA
Sandwich ELISA (DuoSet ELISA R&D systems) for mouse IL-10 and TNF-α was performed according to the manufacturer's instructions using a microplate spectrophotometer Benchmark Plus BIO-RAD at 450 nm with correction at 540 nm.
Flow cytometry
Flow cytometry was carried out using the Flow cytometer BD FacsVerse (BD Biosciences, Breda, Netherlands) using a 640 nm probe for excitation and a 660/10 nm probe for emission when Nile Red was used. For DiOC6 and PI, a 488 nm probe for excitation and a 527/32 and 700/54 nm for emission were used, respectively. Data analysis was performed using the software FlowJo version 10.6.1.
Anti-inflammatory and antiproliferative capacity over time of Sq-GusNPs in macrophages and T cells
Anti-inflammatory capacity in mouse macrophages
Mouse macrophages of the cell line RAW-264.7 at a density of 5 × 104 cells/well were seeded in 24 well plates. Twenty-four hours later cells were washed with PBS and treated with free gusperimus or Sq-GusNPs in an equivalent concentration of 11.2 µg/mL of gusperimus. Two hours later a stimulus with LPS at a concentration of 100 ng/mL was applied and at 24-, 48-, 72-, and 96-h TNF-α and IL-10 secretion was determined by ELISA. As controls, we used cells stimulated with LPS and cells without any treatment.
Antiproliferative capacity in mouse cytotoxic T lymphocytes
CTLL-2 T cells were seeded at a density of 5 × 103 cells/well in 24 well plates and treated with free Gusperimus or Sq-GusNPs in an equivalent concentration of 1 µg/mL of Gusperimus. After 24-, 48-, and 72 h cell proliferation and viability were determined. Cell proliferation was followed using alamarBlue™ reagent as indicated in section Cell viability with alamarBlue™ and reading the produced absorbance. To determine whether the effects on cell proliferation were due to cytotoxicity of the applied treatments, we used flow cytometry counting 1000 events per sample at 20 min after adding 0.06 µg/mL DiOC6 and 1 µg/mL PI. As controls served cells without any treatment.
Quantitative reverse-transcription polymerase chain reaction (qRT-PCR)
A qRT-PCR was performed to determine the gene expression after inducing LDLR in mouse macrophages (RAW-264.7) as described below. After incubation with LPDS, macrophages were homogenized with TRIzol™ reagent and total RNA was isolated following the manufacturer’s instructions. Later cDNA was synthesized using SuperScript II Reverse Transcriptase. Finally, qPCR was performed with a FastStart Universal SYBR Green Master for the genes associated with mouse low-density lipoprotein receptors (LDLR) variants 1, 2, and 3 (primer sequences are listed in ). Reactions were carried out in 384-well PCR plates (Thermo Scientific) using a ViiA7 Real-Time PCR System (Applied Biosystems, Carlsbad, CA, USA). Delta Ct (ΔCt) values were calculated and normalized to the housekeeping genes GAPDH, and β-actin. Delta Delta Ct (ΔΔCt) values were used for the comparative quantification of gene expression.
Table 1. Primer sequences used for qRT-PCR.
Induction of low-density lipoprotein receptors (LDLRs) expression in macrophages
RAW-264.7 macrophages were seeded at a density of 5 × 105 cells/well in 24 well plates and cultured for 24 h. Subsequently, cells were washed with PBS and cultured with LPDS (10%) supplemented medium instead of FCS in a total volume of 1 ml culture medium for an additional 24 h. Finally, the supernatant was discarded, and cells were collected using TRIzol™ reagent to subsequently determine the expression of LDLR associated genes by qRT-PCR. As a control, we used cells cultured in a medium supplemented with 10% FCS.
Cellular uptake of Sq-GusNPs in mouse macrophages
Cellular uptake as a function of temperature
RAW-264.7 macrophages were seeded at a density of 5 × 105 cells/well in 12-well plates and cultured for 24 h. Later cells were washed with PBS, treated with Nile red labelled Sq-GusNPs at a concentration of 22.3 µg/mL, and cultured at 4 or 37 °C in a total volume of 1 ml culture medium. For the treatment at 4 °C, cells were cultured for 1 h at the same temperature before adding the NPs. Six hours later, cells were washed with PBS and trypsinized at 37 °C for 15 min with 300 µL of Trypsin EDTA 0.5%. The action of trypsin was stopped by adding 700 µL of culture medium. Cellular uptake of the labelled NPs was determined through flow cytometry.
Cellular uptake after induction of LDLR
RAW-264.7 macrophages were seeded at a density of 5 × 105 cells/well in 12-well plates and cultured for 24 h. Subsequently, cells were washed with PBS and cultured for an additional 24 h in a culture medium supplemented with 10% of LPDS or FCS. Next, cells were washed with PBS and treated with Nile red labelled Sq-GusNPs in a total volume of 1 ml of either LPDS or FCS supplemented medium. After 6 h of culture, cells were washed with PBS, trypsinized at 37 °C for 15 min with 300 µL of Trypsin EDTA 0.5%, and 700 µL of LPDS or FCS supplemented medium was added. Finally, cellular uptake of the labelled NPs was determined through flow cytometry.
Gusperimus quantification using fluorescamine
Gusperimus quantification using fluorescamine was carried out in black 96-well plates adding 75 µL of samples and standards diluted in PBS to each well. After this 25 µL of fluorescamine solution (3 mg/mL) in acetone was added and the plate was stirred for 1 min. Finally, fluorescence was measured in the plate reader CLARIOstarPlus using 400 ± 30 nm for excitation and 460 ± 40 nm for emission.
In-vitro release experiments for gusperimus from Sq-GusNPs
In-vitro release experiments for gusperimus were performed in 1 ml dialysis devices of 3.5–5 kD exclusion size immersed in 15 ml of PBS (external medium) under stirring at 37 °C for 72 h. The release of gusperimus from Sq-GusNPs was tested in the presence of cathepsins B and D using an equivalent concentration of 76.3 µg/mL of gusperimus. Sq-GusNPs were dispersed in 1 ml of sodium acetate buffer 0.3 M (pH 4.8) into the dialysis device. Aliquots of 500 µL of the external medium were removed at defined times, stored at −20 °C until quantification and the retrieved volume replenished. Gusperimus quantification was carried out using fluorescamine as indicated above. As control Sq-GusNPs and free gusperimus immersed in PBS were used.
Statistics
The experiments were performed at least 3 times and statistical analysis was carried out in GraphPad Prism, Version 9 (GraphPad Software Inc., USA). Normal distribution of data was confirmed using the D'Agostino-Pearson omnibus (K2) test. Comparisons between two groups were analyzed using two-tailed, unpaired t-tests. Comparisons for more than two groups were done using two-way ANOVA with Dunnett's post-hoc test. A p-value <.05 was considered statistically significant. Results are expressed as mean ± standard error of the mean (SEM).
Results
Sq-GusNPs enhances the anti-proliferative and anti-inflammatory effect of gusperimus
Although gusperimus has shown to be safe and effective as an immunosuppressive agent for the treatment of different autoimmune diseases as well as in organ transplantation [Citation3], it suffers from stability issues that limit its application. This instability in vivo is related to its high hydrophilicity [Citation5], which leads to loss of activity and enhanced cytotoxicity [Citation2,Citation6,Citation13]. To overcome this, we previously encapsulated gusperimus as Sq-GusNPs obtaining NPs leading to higher stability, high drug loading capacity, high uptake, lack of toxicity, and sustained anti-inflammatory activity over time [Citation2]. These NPs were obtained through nanoprecipitation [Citation14] by auto-assembly of the Sq-Gus bioconjugate synthesized from squalene and binding it to gusperimus in a multi-step reaction [Citation2].
As it is unknown how the Sq-GusNPs exerts their anti-inflammatory effects, we studied and compared the anti-proliferative and anti-inflammatory effect of the Sq-GusNPs with free gusperimus. This was done on RAW-264.7 macrophages and CTLL-2 cytotoxic T cells. To study the antiproliferative effect we determined the IC50 for the proliferation of RAW-264.7 macrophages when cultured with Sq-GusNPs and free gusperimus. To study anti-inflammatory effects we cultured the cells in the same fashion after which we applied a stimulus with LPS to induce an inflammatory response [Citation15]. Subsequently, we studied cytokine secretion to determine a possible reduction in cytokine release in the macrophages exposed to nanoencapsulated and non-encapsulated gusperimus. The anti-inflammatory effect on CTLL-2 T cells was studied by determining the inhibition on cell proliferation and the cytotoxicity induced after the addition of either free or encapsulated gusperimus.
shows the dose-response curves obtained for free gusperimus and Sq-GusNPs in RAW-264.7 macrophages. A 9-fold lower IC50 value was found for macrophages exposed to Sq-GusNPs (IC50 = 64.8 µM) compared to the free drug (577.0 µM). Effects on proliferation were concentration-dependent and it was not affected up to 50 µM for Sq-GusNPs. For free gusperimus, a more gradual decrease in cell proliferation was observed but only at higher concentrations compared to Sq-GusNPs.
Figure 3. Proliferation dose-response curves for Sq-GusNPs (blue) and free gusperimus (red) in mouse macrophages. A 9-fold lower IC50 value was observed for gusperimus when it was encapsulated as Sq-GusNPs compared with the free drug. Data represent mean values ± SEM of five experiments.
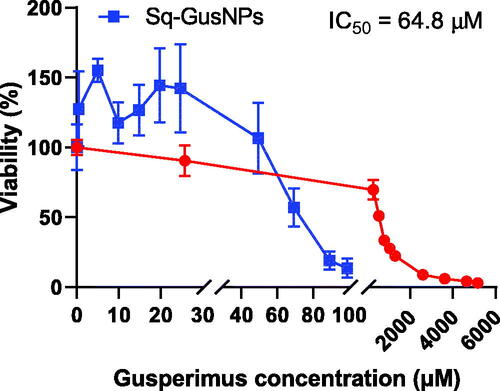
As shown in , Sq-GusNPs reduced the inflammatory response in macrophages. In RAW-264.7 macrophages Sq-GusNPs reduced the secretion of TNF-α and IL-10 to a higher extent than free gusperimus. For TNF-α a reduction of 8.98% (p = .3921), 47.00% (p < .0001), 55.59% (p < .0001), and 49.08% (p < .0001) for 24-, 48-, 72-, and 96 h was observed, respectively (). IL-10 was reduced by 55.25% (p < .0001), 92.67% (p < .0001), 89.35% (p < .0001), and 86.09% (p < .0001) at 24-, 48-, 72-, and 96 h, respectively (). For free gusperimus, a higher reduction compared to Sq-GusNPs of 15.95% (p = .0366) was observed only at 24 h, an increase of 13.65% (p = .0907) at 48 h, and a lower decrease by 15.65% (p = .0415) and 14.91% (p = .0559) at 72- and 96-h was observed for TNF-α secretion (). IL-10 was also reduced to a higher extent for free gusperimus than by Sq-GusNPs in 70.26% (p < .0001) only at 24 h, at 48-, 72-, and 96-h gusperimus reduced this cytokine by 60.41% (p < .0001), 78.52% (p < .0001), and 85.93% (p < .0001), respectively ().
Figure 4. Immunosuppressive effect of Sq-GusNPs and free gusperimus in macrophages and T cells. (A) TNF-α and (B) IL-10 secretion after stimulation of mouse macrophages with LPS and exposure to free or encapsulated gusperimus over time. The anti-inflammatory effect was maintained over time and was higher for cells treated with Sq-GusNPs. (C) Cell proliferation and (D) cytotoxicity of Sq-GusNPs and free gusperimus in mouse cytotoxic T lymphocytes. Sq-GusNPs inhibited cell proliferation without cytotoxic effects. Comparisons were made using two-way ANOVA with Tukey's multiple comparisons test. Macrophages: RAW—234.7 without treatment; T cells: CTLL-2 cells without treatment; LPS: macrophages or T cells stimulated with LPS; Gus: Macrophages or T cells treated with free gusperimus and stimulated with LPS; Sq-GusNPs: Macrophages or T cells treated with Sq-GusNPs and stimulated with LPS. Data represent mean values ± SEM of three experiments. ****p ≤ .0001; ***p < .0005; **p < .008; *p < .05; ns: non-significant difference.
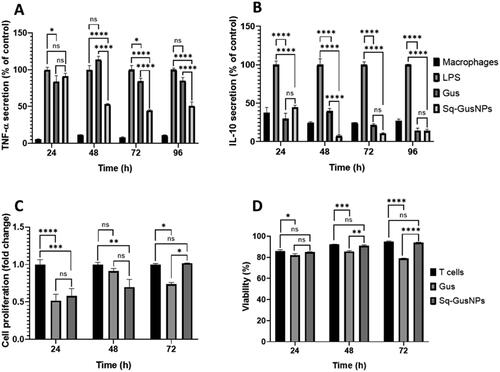
In T cells Sq-GusNPs showed an anti-proliferative effect. We found a reduction in cell proliferation at 24 and 48 h by 41.94% (p = .0004), and 30.28% (p = .0071), respectively (). For free gusperimus, a reduction in cell proliferation of 48.39% (p < .0001), 8.83% (p = .5767), and 26.26% (p = .0192) at 24-, 48-, and 72-h was observed, respectively but it was associated with cytotoxicity as shown in which was not observed with Sq-GusNPs.
Sq-GusNPs are internalized via LDLR mediated endocytosis
As we showed above, nanoencapsulation of gusperimus enhances its anti-inflammatory efficacy. It is unknown, however, how this nanoencapsulation has increased its efficacy. We therefore next studied its internalization process which might involve the LDLR as this receptor has been reported to be involved in endocytosis of squalene-derived NPs [Citation8,Citation11]. We did this in a stepwise strategy. First, we studied the possible endocytosis of the nanoparticles. As endocytosis requires energy, we studied the uptake of labelled Sq-GusNPs NPs at 4 °C, and 37 °C in RAW-264.7 macrophages. Next, to study the involvement of the receptor for low-density lipoproteins (LDL), we enhanced LDLR expression in RAW-264.7 macrophages by depriving the cells of lipoproteins [Citation8,Citation11] by culturing them in a culture medium supplemented with lipoprotein deficient serum instead of foetal calf serum. Next, the RAW-264.7 macrophages with enhanced LDLRs were exposed to labelled Sq-GusNPs after which NPs uptake was determined through flow cytometry.
shows that NPs uptake was significantly decreased by 26.5% (p < .0001) when cells were cultured at 4 °C. This demonstrates that the internalization of the Sq-GusNPs is an energy-dependent mechanism pointing to endocytosis as the principal internalization pathway. After depriving macrophages of lipoproteins, a higher expression of the LDLR gene was confirmed (). There are three variants of the LDLR which were all increased in RAW-264.7 macrophages. An increase of 39.5% (p = .0006), 31.9% (p = .0015), and 42.1% (p = .0110) in gene expression for variants 1, 2, and 3 were observed, respectively (). Next, we studied the uptake of labelled Sq-GusNPs and found a 3-fold increase in Sq-GusNPs uptake (p < .0001) in RAW-264.7 macrophages with enhanced LDLR expression (). This finding demonstrates that increased uptake of gusperimus after nanoencapsulation is via LDLR dependent endocytosis.
Figure 5. Uptake of Nile red labelled Sq-GusNPs by mouse macrophages. (A) Uptake of Nile red labelled Sq-GusNPs by macrophages cultured in complete medium at 37 and 4 °C. The lower uptake at 4 °C demonstrates that the internalization of the nanoparticles was mainly performed via an energy-dependent mechanism. (B–D) Are gene sequences associated with variants 1, 2, and 3 of the LDLR gene, respectively. In all cases, a higher expression of LDLR was observed after culturing the macrophages with LPDS for 24 h. (E) Uptake of Nile red labelled Sq-GusNPs by macrophages expressing normal and higher levels of LDLR. A 3-fold increase in Sq-GusNPs uptake was observed after inducing the expression of LDLRs in macrophages confirming the participation of LDLR in the internalization of Sq-GusNPs. Complete medium: macrophages incubated in culture medium supplemented with 10% FBS; LPDS medium: macrophages incubated in culture medium supplemented with 10% of LPDS instead of FBS. Comparisons were made using a two-tailed, unpaired t-test. Data represent mean values ± SEM of five experiments. ****p < .0001; ***p = .0006; **p = .0015; *p = .0110.
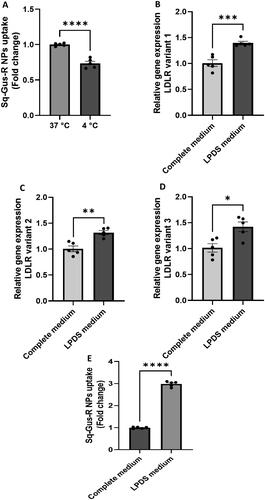
Gusperimus is released intracellularly after internalization
The Sq-GusNPs are formed by auto-assembly of the Sq-Gus prodrug molecules [Citation2]. After internalization, the prodrug needs to be cleaved to allow gusperimus to exert its effect. Prodrugs are bio-reversible derivatives that undergo an enzymatic or a chemical transformation to release the active drug [Citation12]. To demonstrate that gusperimus is released intracellularly after internalization followed by enzymatic degradation, we performed in vitro release studies simulating the intracellular environment in presence of proteases. To do this, we cultured the Sq-GusNPs in presence of cathepsins B and D in acidic conditions inside dialysis devices immersed in PBS followed by evaluation of the release of gusperimus.
shows the release profiles obtained for gusperimus from Sq-GusNPs at physiological pHs (7.4 and 4.8) and free gusperimus at pH 7.4. We choose for the Sq-GusNPs not only at physiological pH of 7.4 but also 4.8 as intracellularly, after endocytosis, acidic conditions of ∼4.8 may exist to which the Sq-GusNPs are exposed. At these lower pH values, more amide bonds can be cleaved by the action of proteases like cathepsins [Citation11,Citation16]. As shown in , the cathepsins in acidic conditions caused 3 times higher release of gusperimus from Sq-GusNPs in the first 24 h when compared to control NPs in PBS without exposure to cathepsin B and D. This demonstrates that after internalization the Sq-GusNPs release gusperimus intracellularly after being cleaved by the proteases like cathepsins B and D.
Figure 6. Release profile for free gusperimus (red) and Sq-GusNPs (blue) in PBS at pH 7.4, and for Sq-GusNPs in presence of cathepsins B (green) or D (black) at pH 4.8. After exposing Sq-GusNPs to cathepsins in acidic conditions gusperimus is released to a higher extend confirming that the Sq-GusNPs acts as a prodrug and release gusperimus intracellularly after being cleavage by proteases.
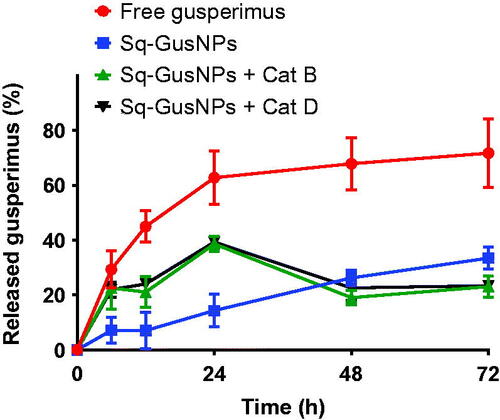
Discussion
This study aimed to characterize the in-vitro action of Sq-GusNPs as an anti-inflammatory agent as well as to elucidate how these NPs are internalized and maintain the therapeutic effect of gusperimus. As gusperimus has shown to exert its immunosuppressive effects in both innate and adaptative arms of the immune system [Citation3] we studied the anti-inflammatory and anti-proliferative effects of Sq-GusNPs on macrophages and T-cells. Macrophages are key players of the innate immune system. They have three major functions, i.e. serving as antigen-presenting cells, to phagocytose, and to immunomodulate the microenvironment through secretion of various cytokines and growth factors [Citation17]. T lymphocytes are in general the key players of the adaptative cellular immune responses and cytotoxic T lymphocytes are responsible for its effector function. Cytotoxicity towards T lymphocytes is exerted directly through the Fas or perforin pathway and/or indirectly by the release of cytokines [Citation18].
To evaluate the safety and efficacy of free gusperimus and Sq-GusNPs, we determined the IC50 in macrophages. We found that nanoencapsulation increased gusperimus antiproliferative effects. The enhanced efficacy was corroborated by the observation that cell proliferation was 50% decreased with a 9-fold lower dose of nanoencapsulated gusperimus compared to free gusperimus. More importantly, the Sq-GusNPs do not inhibit macrophages proliferation at equivalent concentrations as high as 50 µM of gusperimus. This shows that encapsulation of gusperimus as squalene-derived nanoparticles enhances efficacy, as Sq-GusNPs have a more potent and stronger anti-inflammatory effect at a relatively low equivalent concentration of gusperimus of 11.2 µg/mL which confirms previous observations [Citation2].
The enhanced anti-inflammatory effect of Sq-GusNPs over free gusperimus was also corroborated by the observation that secretion of TNF-α and IL-10 was reduced. This was done as gusperimus has been shown to reduce the level of both the pro-inflammatory cytokine TNF-α as well as that of the regulatory cytokine IL-10 [Citation3]. The reduction in both cytokines was most strong after 48 h and less pronounced at 24 h. The fact that for free gusperimus a higher reduction in cytokine secretion was obtained at 24 h may be associated with its immediate availability, since, unlike gusperimus, Sq-GusNPs need to be cleaved to release the free drug due to its prodrug nature which is a time-dependent process [Citation19]. The efficacy and safety of Sq-GusNPs on T-cells were also illustrated by its inhibiting effect on cell proliferation and lack of cytotoxicity. Our results also suggest that Sq-GusNPs interfere with the cell's response to IL-2 as the cells were stimulated with IL-2 which was a constituent of the medium and despite this was not able to stimulate proliferation. Even though free gusperimus also showed an anti-proliferative effect on T-cells this was probably not via the same mechanism by which Sq-GusNPs had antiproliferative effects. With the free form of gusperimus, we observed cell death probably due to the release of toxic metabolites of gusperimus after oxidation by amine oxidase which is present in the serum of the culture medium [Citation20]. This shows that encapsulation of gusperimus as Sq-GusNPs not only stabilizes gusperimus but also avoids cytotoxic effects associated with breakdown products of free gusperimus [Citation6,Citation13].
We found endocytosis to be the principal mechanism of the internalization of Sq-GusNPs. This was concluded after observing a decrease in the uptake rate at low temperature which lowers the energy that is needed for endocytic uptake [Citation8]. Additionally, the enhanced uptake of nanoparticles after increasing expression of LDLR on macrophages confirms the involvement of this receptor in the cellular internalization of Sq-GusNPs. This corroborates previous findings that LDLR mediated endocytosis is a pathway for the internalization of squalene-derived NPs as has been demonstrated for other squalene-derived nanoparticles like squalene-gemcitabine NPs [Citation8], squalene-adenosine NPs [Citation11], and squalene-cyanine NPs [Citation10]. Since cytotoxic T cells also possess an active cholesterol metabolism which is mediated by LDLR [Citation21], the Sq-GusNPs [Citation2] uptake probably follows the same pathway.
After being internalized, Sq-GusNPs release gusperimus which has an immunosuppressive effect by acting as an anti-proliferative and anti-inflammatory drug. By mimicking the acidic circumstances in endo-lysosomal compartments we demonstrated that Sq-GusNPs effectively release gusperimus when exposed to the lysosomal enzymes cathepsin B or D [Citation16]. This proves that after internalization Sq-GusNPs underwent degradation by cleavage of the Sq-Gus bio-conjugate and release gusperimus by the action of hydrolases, such as cathepsins which are present in varying amounts within endosomes and lysosomes [Citation16].
Based on our findings we proposed the mechanism of internalization of Sq-GusNPs and release of gusperimus from the NPs as shown in . LDLR binding facilitates cell internalization of Sq-GusNPs through the clathrin-dependent endocytosis [Citation22]. In this receptor-mediated endocytosis pathway, the LDLR recognizes the low-density lipoproteins as extracellular ligands which interact with squalene-derived nanoparticles on its surface [Citation7,Citation9–11]. The LDLR at the same time recruits the autosomal recessive hypercholesterolaemia (ARH) adaptor protein which is specific for this receptor [Citation23] leading to membrane invagination [Citation24]. Next, recruitment of clathrin coating complex results in vesicle formation that contains receptor-ligand complexes and Sq-GusNPs which are delivered to the endosomal compartment where NPs starts to disassembly in Sq-Gus biconjugate molecules and are transported to the lysosome for degradation [Citation24] by proteases, such as cathepsin B and D. This induces the release of gusperimus and the observed immunosuppressive effect.
Figure 7. Gusperimus release mechanism from Sq-GusNPs after internalization. LDL: low-density lipoproteins; Sq-GusNPs: squalene-gusperimus nanoparticles; LDLR: low-density lipoprotein receptor; ARH adaptor: autosomal recessive hypercholesterolaemia adaptor protein; Clathrin: clathrin protein; Sq-Gus: squalene-gusperimus bioconjugate.
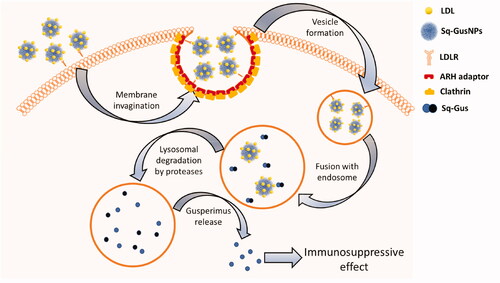
Conclusion
This study shows the safety and enhanced immunosuppressive effect of Sq-GusNPs. The NPs are internalized via LDLR-mediated endocytosis and intracellularly gusperimus is released after cleavage of the Sq-Gus bioconjugate to exert its immunosuppressive effect. These studies provide insight into the internalization and release mechanism of squalene-derived NPs as a general mechanism considering previous studies [Citation8,Citation10,Citation11]. The fact that Sq-GusNPs are internalized via LDLR makes these NPs an interesting approach to manage inflammatory responses in which macrophages are involved [Citation15] as they have an active LDL metabolism [Citation9]. This together with the immunosuppressive effect and lack of toxicity on T cells and macrophages make the Sq-GusNPs a potential therapeutic agent for different autoimmune diseases, such as vasculitis, glomerulonephritis, Wegener’s granulomatosis, systemic lupus erythematosus, rheumatoid arthritis, Crohn’s disease, or in the prevention of transplant rejection [Citation25].
Abbreviations | ||
Sq-GusNPs | = | squalene-gusperimus nanoparticles |
LDLR | = | low-density lipoprotein receptors |
NPs | = | nanoparticles |
LDL | = | low-density lipoproteins |
qRT-PCR | = | quantitative reverse-transcription polymerase chain reaction |
Sq-Gus | = | squalene-gusperimus |
FCS | = | foetal calf serum |
LPDS | = | lipoprotein deficient serum |
LPS | = | lipopolysaccharides |
IC50 | = | half-maximal inhibitory concentration |
Disclosure statement
The authors declare no conflict of interest.
Data availability statement
Data supporting the findings of this study are available within the article and from the corresponding author upon reasonable request.
Additional information
Funding
References
- Kälsch AI, Schmitt WH, Breedijk A, et al. In vivo effects of cyclic administration of 15-deoxyspergualin on leucocyte function in patients with Wegener's granulomatosis. Clin Exp Immunol. 2006;146(3):455–462.
- Navarro Chica CE, de Haan BJ, Faas MM, et al. Design and characterization of Squalene-Gusperimus nanoparticles for modulation of innate immunity. Int J Pharm. 2020;590:119893.
- Perenyei M, Jayne DRW, Floßmann O. Gusperimus: Immunological mechanism and clinical applications. Rheumatology. 2014;53(10):1732–1741.
- Kaufman DB, Gores PF, Kelley S, et al. 15-Deoxyspergualin: immunotherapy in solid organ and cellular transplantation. Transplant. Rev. 1996;10(3):160–174.
- Gusperimus|C17H37N7O3 – PubChem (n.d.) [cited 2019 Oct 23]. Available from: https://pubchem.ncbi.nlm.nih.gov/compound/55362#section=Experimental-Properties
- Fujii H, Takada T, Nemoto K, et al. In vitro immunosuppressive properties of spergualins to murine T cell response. J Antibiot. 1989;42(5):788–794.
- Yesylevskyy SO, Ramseyer C, Savenko M, et al. Low-density lipoproteins and human serum albumin as carriers of squalenoylated drugs: insights from molecular simulations. Mol Pharm. 2018;15(2):585–591.
- Sobot D, Mura S, Rouquette M, et al. Circulating lipoproteins: a trojan horse guiding squalenoylated drugs to LDL-accumulating cancer cells. Mol Ther. 2017;25(7):1596–1605.
- Brusini R, Dormont F, Cailleau C, et al. Squalene-based nanoparticles for the targeting of atherosclerotic lesions. Int J Pharm. 2020;581:119282.
- Sobot D, Mura S, Yesylevskyy SO, et al. Conjugation of squalene to gemcitabine as unique approach exploiting endogenous lipoproteins for drug delivery. Nat Commun. 2017;8:15678–15679.
- Rouquette M, Lepetre-Mouelhi S, Dufrançais O, et al. Squalene-adenosine nanoparticles: ligands of adenosine receptors or adenosine prodrug? J Pharmacol Exp Ther. 2019;369(1):144–151.
- Feng J, Lepetre-Mouelhi S, Couvreur P. Design, preparation and characterization of modular squalene-based nanosystems for controlled drug release. Curr Top Med Chem. 2017;17:2849–2865.
- Kerr PG, Ntkolic-Paterson DJ, Lan HY, et al. Deoxyspergualin suppresses local macrophage proliferation in rat renal allograft rejection. Transplantation. 1994;58:596–601.
- Barreras-Urbina CG, Ramírez-Wong B, López-Ahumada GA, et al. Nano- and micro-particles by nanoprecipitation: possible application in the food and agricultural industries. Int J Food Prop. 2016;19(9):1912–1923.
- Anderson JM, Rodriguez A, Chang DT. Foreign body reaction to biomaterials. Semin Immunol. 2008;20(2):86–100.
- Asokan A, Cho MJ. Exploitation of intracellular pH gradients in the cellular delivery of macromolecules. J Pharm Sci. 2002;91(4):903–913.
- Fujiwara N, Kobayashi K. Macrophages in inflammation. Curr Drug Targets Inflamm Allergy. 2005;4(3):281–286.
- Andersen MH, Schrama D, Thor Straten P, et al. Cytotoxic T cells. J Invest Dermatol. 2006;126(1):32–41.
- Rautio J, Kumpulainen H, Heimbach T, et al. Prodrugs: design and clinical applications. Nat Rev Drug Discov. 2008;7(3):255–270.
- Kerr PG, Atkins RC. Deoxyspergualin inhibits cytotoxic T lymphocytes but not NK or LAK cells. Immunol Cell Biol. 1991;69(3):177–183.
- Yuan J, Cai T, Zheng X, et al. Potentiating CD8+ T cell antitumor activity by inhibiting PCSK9 to promote LDLR-mediated TCR recycling and signaling. Protein Cell. 2021;12(4):240–260.
- Goldstein JL, Brown MS, Anderson RG, et al. Receptor-mediated endocytosis: concepts emerging from the LDL receptor system. Annu Rev Cell Biol. 1985;1:1–39.
- McMahon HT, Boucrot E. Molecular mechanism and physiological functions of clathrin-mediated endocytosis. Nat Rev Mol Cell Biol. 2011;12(8):517–533.
- Kzhyshkowska J, Neyen C, Gordon S. Role of macrophage scavenger receptors in atherosclerosis. Immunobiology. 2012;217(5):492–502.
- Slobbe L. Drugs that act on the immune system: immunosuppressive and immunostimulatory drugs. 1st ed. Amsterdam, The Netherlands: Elsevier B.V.; 2012.