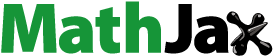
Abstract
Drug-loaded nanoparticles (NPs) allow specific accumulation and controlled release of drugs to infected tissues with minimal cytotoxicity. In this study, gemifloxacin conjugated silver nanoparticles (Gemi-AgNPs) were synthesized, and the amplification of their antibacterial potential against the human pathogen as well as their stability was monitored under physiological conditions. Fourier transform infrared spectroscopy (FTIR) analysis demonstrated the interaction between –NH2 and –OH functional moiety and the metal surface. The morphological analyses via transmission electron microscopy revealed that Gemi-AgNPs has a round oval shape and average particle size of 22.23 ± 2 nm. The antibacterial and antibiofilm activities of these NPS showed that Gemi-AgNPs exhibit excellent antimicrobial and biofilm inhibition activity against human pathogens, namely, Proteus mirabilis (P. mirabilis) and methicillin-resistant Staphylococcus aureus (MRSA). A significant increase in the antibiofilm activity of Gemi-AgNPs was confirmed by crystal violet, 3-(4,5-dimethylthiazol-2-yl)-2,5-diphenyltetrazolium bromide (MTT) staining, and microscopic analysis. Gemi-AgNPs exhibited the ability to inhibit urease with an IC50 value of 57.4 ± 0.72 µg/mL. The changes in the bacterial cell morphology were analyzed via TEM, which revealed that cell membranes disrupted and completely destroyed the cell morphology by the treatment of Gemi-AgNPs.
Introduction
Multidrug resistance (MDR) of bacteria, as a result of excessive use of drugs, has become a great concern, and its treatment is expensive but less effective [Citation1]. Silver and its compounds have been known since the early times; however, their application has decreased due to the emergence of antibiotics [Citation2]. Nanomedicine potency can be programmed by tuning the physicochemical parameters, namely, size, shape, and surface [Citation3]. Nanomaterials penetrate deep into the cells and tissues due to the size similarity between nanoparticles (NPs) and biological molecules [Citation4]. The similarity in NPs silver ions and its compounds was toxic to major bacterial species [Citation5]. Nanotechnology has proved effective in exploring the hidden secrets of already-known antibacterial agents by tuning the particle size and shape [Citation6,Citation7]. The mechanical, chemical, magnetic, and catalytic properties of nanomaterials are associated with their larger surface compared with bulk material [Citation8,Citation9]. Several methods, including physical, chemical, and biological methods, were employed for the synthesis of NPs, with the chemical method being the most effective [Citation10–12]. The emergence of numerous drug-loaded NPs resulted in the selective killing of microorganisms and the transport of drugs to the infected site [Citation13,Citation14]. Similarly, Jamshidifar et al. [Citation15] unravelled the potential of NiCoFe2O4@L-Silica-L@C-Niosome NPs against MDA-MB-231 and SK-BR-3 breast cancer cells. About 40% of bioactive compounds exhibit poor solubility in water; thus, efforts were made to increase their solubility via NP formation [Citation16,Citation17]. Samie et al. evaluated the antimicrobial activity of Pyrenacantha grandiflora tuber extracts via conjugation with penicillin, ampicillin, vancomycin, and silver nanoparticles (AgNPs) against Escherichia coli (E. coli), Staphylococcus aureus (S. aureus), and Klebsiella pneumoniae (K. pneumoniae). The conjugation of P. grandiflora with penicillin, ampicillin, and vancomycin in the presence of AgNPs improved their biological activities against K. pneumoniae and E. coli. Therefore, these conjugations are very important and can be used to improve the therapeutic potential of existing antibiotics against K. pneumoniae and E. coli [Citation18]. Anwar et al. conjugated nystatin (macrocyclic polyenes), amphotericin B, and fluconazole (azole) with AgNPs, and their results indicated that conjugation of amphotericin B and nystatin with AgNPs exhibited significant antiamebic potential as compared with drugs alone or AgNPs alone [Citation19]. Moreover, Targhi et al. [Citation20] used curcumin-loaded silver/copper nanoparticles (NPs) against Staphylococcus aureus (S. aureus) and Pseudomonas aeruginosa (P. aeruginosa). The drug-loaded niosomes and their hydrogel counterpart were screened for investigating their antibacterial activity against S. aureus and P. aeruginosa via disc diffusion and minimum inhibitory concentration (MIC). Other than that plants based AgNPs have significant antimicrobial, antioxidant and anti-cancer activities [Citation21,Citation22]. AgNPs exhibit durable optical topographies and large surface/volume ratios that make them appropriate for drug sensing, stacking, and controlled release [Citation23,Citation24]. Additionally, they are characterized as next-generation antimicrobial agents against multidrug-resistant bacteria [Citation25–28]. Several studies reported that AgNPs conjugated with different drugs, such as penicillin, amoxicillin, tetracycline, and neomycin enhanced their antimicrobial effects against nosocomial infection in comparison with AgNPs or antibiotics alone [Citation29,Citation30]. Gemifloxacin, a new class of compounds, belongs to the fluoroquinolone group and has a strong antibacterial activity against Gram-positive pathogens [Citation31–34]. Antibacterial resistance to fluoroquinolone antibiotics is an area of intense research [Citation35–38]. The bactericidal activity of gemifloxacin proceeds via the inhibition of topoisomerase II. Moreover, the use of gemifloxacin for the facile synthesis of AgNPs proved to be of utmost importance because of the presence of amine and carboxylic acid moieties in its structure. In recent studies, gemifloxacin conjugated silver nanoparticles (Gemi-AgNPs) were synthesized using a simple and facile chemical method. Nanoparticles synthesized from chemical methods are small in size and stable for up to the duration of two weeks. Moreover, the current chemical approach facilitates the rapid formation of NPs in contrast to any other methods that were unable to synthesize Gemi-Ag NPs. For in vivo application, the stability of synthesized NPs was ascertained against certain parameters, such as brine concentration, temperature, and pH. To the best of our knowledge, this is the first study on Gemi-AgNPs and their urease enzyme inhibition, antibacterial, and antibiofilm activities against Enterobacter aerogenes (E. aerogenes), Enterococcus faecalis (E. faecalis), K. pneumoniae, Proteus mirabilis (P. mirabilis), Streptococcus mutans (S. mutans), and methicillin-resistant S. aureus (MRSA). Moreover, the morphological changes in the bacterial cell in the presence Gemi-AgNPs were also analyzed via transmission electron microscopy (TEM).
Materials and methods
In this study, silver nitrate (AgNO3), sodium borohydride (NaBH4), hydrochloric acid (HCl), and sodium hydroxide (NaOH), which are of analytical grade and obtained from Sigma-Aldrich, United States, were used. Standard antibiotic gemifloxacin () was purchased from Abbott Pharmaceutical Company, Pakistan. Digital pH metre model 510 (Oakton, Eutech) with a glass working electrode was used for pH adjustment. UV–Vis spectra were recorded on Shimadzu UV-240, Hitachi U-3200 spectrometer. FTIR spectra were logged on the Shimadzu IR-460 spectrophotometer, and the size and morphology of the nanomedicine were checked via atomic force microscopy (AFM) and TEM.
Synthesis of nanoparticles
Around 1 mM solution of silver nitrate was prepared by dissolving 42.46 mg in 250 mL of double-distilled water. Similarly, a 1 mM solution of gemifloxacin was prepared by dissolving 97.35 mg in 250 mL in double-distilled water. The optimum metal/ligand ratio was determined based on the maximum absorption peak in the wavelength range of 300–400 nm for NP synthesis. The change in the colour of the solution is a primary indication of NP formation; subsequently, AgNP formation was further confirmed via UV–Vis spectrophotometry.
Stability of nanoparticles
The stability of NPs was optimized against diverse ranges of pH, temperature, and brine concentration to study their behaviour under physiological conditions. The UV–Vis spectra were recorded after 24 h; any increase or decrease in absorbance intensity gives information about the stability of NPs.
Fourier transform infrared spectroscopy (FTIR)
The FTIR spectra were documented via a spectrophotometer (IR-460 Shimadzu, Kyoto, Japan) to detect the occurrence of distinct functionalities in the drug and drug-loaded AgNPs. For analysis, the resulting suspension was washed with deionized water and then freeze-dried.
Transmission electron microscopy (TEM)
The TEM (Model: JEOL, JEM-1400 Electron Microscope) was used for the size and morphology of AgNPs operated at an accelerating voltage of 110 kV. The NPs samples were prepared for TEM analysis by drop-coating the AgNP solution on carbon-coated copper grids.
AFM analysis
To confirm the size and morphology of AgNPs, the AFM (Agilent Technologies 5500, USA) Agilent 5500 was used operated in a tapping mode.
Biological activities
Bacteria sample and growth media
The antibacterial efficacy of the synthesized NPs (Gemi-AgNPs) was evaluated against different Gram-positive and Gram-negative bacterial isolates. S. mutans (ATCC: 25175) was maintained and passaged using heart infusion (Oxoid, UK) broth and agar containing 1% sucrose. Conversely, MRSA (ATCC: 43300), K. pneumoniae (ATCC: 13882), P. mirabilis (ATCC: 12453), E. aerogenes (ATCC: 13048), E. faecalis (ATCC: 29212), and E. coli (ATCC: 25922) were maintained in trypticase soy agar/broth (TSA or TSB; Oxoid, UK). All the six strains of bacteria were kept at 37 °C for 24 h under aerobic conditions.
Minimum inhibitory concentration (MIC) and biofilm inhibition potential of the nanoparticles
The antibacterial potential of the newly synthesized NPs (Gemi-AgNPs) was determined by employing the broth microdilution method using a microtiter 96-well plate according to CLSI guidelines [Citation39,Citation40]. Gemifloxacin and Gemi-AgNPs were diluted 2-fold in Mueller Hinton broth (NutriSelect is a trademark of Merck KGaA, Darmstadt, Germany). The bacterial strains were subsequently added to the corresponding individual well at a strength of 5 × 105 CFU/mL. Each lane was augmented by providing a vehicle control prepared in 1% DMSO with positive and negative controls. After preparation, the plate was incubated overnight at 37 °C. The (MIC) was calculated as the lowest concentration at which the bacteria growth halted. For antibiofilm screening, the plate was prepared as mentioned above; however, after incubation, the biofilms were stained using 1% crystal violet as described previously [Citation41,Citation42]. Briefly, after incubation, the spent medium was decanted; then, the wells were washed with sterilized saline and temperature-fixed for 45 min at 65 °C. After fixation, the wells were stained with 1% crystal violet for 20 min at room temperature. The plate was washed to remove the unbound excess stain, and 30% glacial acetic acid (200 µL) was added to each well to solubilize the biofilm-bound dye; absorbance was then read at 590 nm. The lowest concentration which completely inhibited biofilm was considered as the minimum biofilm inhibitory concentration (MBIC). The percentage biofilm inhibition was evaluated using the following equations:
Effect of compounds on biofilm viability
Biofilm viability after treatment with newly synthesized NPs (Gemi-AgNPs) was evaluated using MTT dye [Citation41]. After overnight incubation, the nutrient-exhausted media and planktonic cells were removed aseptically, the wells were gently washed, and 200 µL of 0.5 µg/mL MTT solution was added, followed by incubation for 3 h at 37 °C. The actively metabolized cells within the biofilm will reduce the due resulted in the formation of formazan crystals which were dissolved in DMSO (200 µL). The absorbance value (570 nm) was calculated for each strain using a microtiter plate reader (Tecan, Austria USA). Metabolic inhibition was calculated by using the following equation:
Eradication of established biofilm
The eradication of established biofilm was also determined using the crystal violet method [Citation41]. The overnight static biofilms of MRSA, K. pneumoniae, and S. mutans were challenged with different concentrations of gemifloxacin and Gemi-AgNPs along with positive and negative controls, respectively. The eradication potential was evaluated via crystal violet staining and visualized using the Nikon TE 2000 Inverted microscope (Nikon, Japan).
Urease inhibition protocol
The in-vitro urease inhibition assay was performed in 96-well plates with 200 μL reaction volume, containing 25 μL of urease enzyme, 55 μL urea, and 5 μL of the test sample (0.2 mg/mL). The enzyme was incubated with urea and sample for 15 min at 30 °C and then added 45 μL phenol reagent and 70 μL of alkali reagent. Urease activity was determined by measuring the ammonia production using the indophenol method, as described by Weatherburn [Citation43]. The changes in absorbance were continuously measured for 50 min at 630 nm in a microplate spectrophotometer (Bio-Rad, USA). The percentage inhibitions were measured using the formula 100 − (OD testwell/OD control) × 100.
Cell wall damage by TEM
The antimicrobial potential of the freshly synthesized NPs (Gemi-AgNPs) was determined by employing the broth microdilution method using a microtiter 96-well plate [Citation39,Citation40]. In short, agar medium was used as a nutrient to grow E. coli, K. pneumoniae, and E. aerogenes at a concentration of 106 cells per mL. The 60-mm well was made using a borer. The 100 µg/mL standard solution of Gemi-AgNPs was used to evade a non-specific merged zone of inhibition. In each well, 5 µg/mL stock solution of Gemi-AgNPs was added, and the plate was incubated at room temperature for 2 h to allow the occurrence of the diffusion process before it was incubated for 24–48 h at 37 °C. After 24 h, both the control and Gemi-AgNP-treated bacteria were dissolved in high-performance liquid chromatography grade ethanol. Bacterial cell wall damage was analyzed via TEM. Before performing TEM analyses, the bacterial solution was treated with uranyl acetate solution (2 mg/100 mL) to make the bacteria visible in TEM.
Results and discussion
Synthesis of nanoparticles
Gemi-AgNPs were synthesized using the chemical method. About 1 mM solution of silver nitrate (42.46 mg/250 mL) and gemifloxacin (97.35 mg/250 mL) was prepared in double-distilled water. The reaction was optimized at different metal/drug ratios and kept on stirring at room temperature. No change in solution colour was noticed as evident with the naked eye. Therefore the addition of two to three drops of sodium borohydride (1 mM) solution in metal-drug solution will result in rapid variation in solution colour as evident from their surface plasmon. Different metal-drug/drug-metal ratio was used for Gemi-Ag NPs syntheses and kept for 24 h. However, after 24 h the best optimizing ratio was found to be 1:3 (metal/ligand). Additionally, Gemi-Ag nanoparticles synthesized from chemical methods exhibit small particles size and were found stable for more than two weeks. Furthermore, Gemi-Ag NPs synthesis has been carried out in an effective, fast, and low-cost manner. Stability study of synthesized Gemi-AgNPs was conducted against various ranges of temperature, pH, and brine concentration.
Reaction optimization
The reaction mixture of Gemi-AgNPs was optimized using different metal/ligand ratios. The ratio of 1:3 gave a strong surface plasmon peak at 425 nm and was selected for NP synthesis. An upsurge in drug concentration will result arise in surface plasmon resonance, which corresponds to a decrease in particle size. This means that an optimum amount of drug is necessary for the complete reduction of Ag+1 to Ag0. The change in the colour and appearance of plasmon at 425 nm is indicative of Gemi-AgNP formation [Citation21]. The appearance of plasmon at 425 nm revealed that NPs were small in size and have a large surface area [Citation22]. The UV–Vis spectra of gemifloxacin and Gemi-AgNP at different ratios are presented in .
pH effect
The stability of Gemi-AgNPs was checked under extremely acidic pH (2–3, 4–5), neutral pH (6–7), and extremely basic conditions (12–13). NPs were found to be acidic in nature with pH values of 4–5 as well as stable in neutral and slightly acidic and basic environments; however, their stability decreases in strongly acidic and basic media. The instability of NPs under extremely acidic and basic conditions is associated with the − OH (−C=O) and − NH2 groups. The effect of pH on NP stability is presented in .
Temperature effect
The NP solution was heated to 100 °C for 30 min, and the UV–Vis spectra were recorded. The NPs were found to be unstable with the increase in temperature, and the absorbance of the reaction mixture decreased with a rise in temperature. The instability of Gemi-AgNPs at a high temperature is associated with the electron dephasing mechanism [Citation44]. Moreover, with the decrease in particle size, the collision among conduction electron on NPs surface become dominant which results in the dephasing of electrons [Citation45,Citation46]. The effects of temperature on the stability of NPs are presented in .
FTIR analysis of Gemi-AgNPs
FTIR analysis plays a crucial role in the detection of functional moiety and its interaction with the metal surface. The appearance of a peak at 3398–2400 cm−1 is characteristic of hydrogen bonding among the –NH2 and –OH functional groups. Peaks at 1680 and 1560 cm−1 correspond to the stretching of carbonyl (amide functional moiety) and carbon–carbon double bond. The disappearance of peak broadening in NP spectra confirms the conjugation of −NH2 and −OH to the surface of NPs. When gemifloxacin was assigned with the metal surface, the blue shift was observed in the band (1560–1458 cm−1). Moreover, the peak related to the −NH2 and carbonyl groups significantly diminished after NP formation (). The FTIR spectra of Gemi-AgNPs and parent compounds are presented in . The mechanism of gemifloxacin conjugation with AgNPs is presented in .
Table 1. FTIR spectral assignment of free gemifloxacin and Gemi-AgNPs.
Atomic force microscopy
The surface topology of Gemi-AgNPs was studied via AFM. AFM gives information about the size of NPs. NPs were polydispersed and have an average particle size of 22.23 ± 2 nm. The AFM image of Gemi-AgNPs is presented in .
TEM analyses
The size and morphology of NPs were determined via TEM. NPs were found to have a round oval shape and differ in size from 5 to 50 nm. The TEM results were in close agreement with those of the AFM results. The average particle size of Gemi-AgNPs was found to be 22.23 ± 2 nm, calculated using the ImageJ software ().
Antibacterial and antibiofilm activities
AgNPs showed significant antibacterial and antibiofilm activity especially against the resistant pathogen, such as S. aureus, MRSA, and P. aeruginosa [Citation20–22,Citation27,Citation28]. Keeping in view this, antibiofilm activity was performed for gemifloxacin and its conjugated NPs using six different bacterial strains. Aside from MRSA, S. aureus, E. aerogenes, and K. pneumoniae, no significant different between activities were found between the drug and its NP. It is clear from that conjugated NPs were more effective and potent against S. aureus and K. pneumoniae compared with the other bacteria used in this study.
Table 2. Biofilm inhibition of gemifloxacin and Gemi-AgNPs.
The prepared gemifloxacin NPs significantly inhibited the biofilm of S. mutans and MRSA at sub-MIC levels (). They also inhibited the biofilm of other isolates, but not significantly compared with gemifloxacin alone; moreover, they required the same or higher concentration (). For S. mutans, gemifloxacin at 0.4 µg/mL inhibited 87.49% ±1.1 of biofilm; however, its prepared NP significantly inhibited the same amount of biofilm at 0.2 µg/mL. Similarly, for MRSA, gemifloxacin inhibited 90%± 5.3 of biofilm at 0.05 µg/mL; however, its AgNP inhibited more than 90% of biofilm at 0.025 µg/mL (). The biofilm eradication potential of the prepared NP was also checked against MRSA, S. mutans, and K. pneumoniae as presented in . Out of the tested strains, it significantly eradicated the preformed biofilm of MRSA. The prepared NP significantly eradicated 60% of the preformed biofilm at 1 µg/mL; however, gemifloxacin eradicated the same amount of biofilm at 10 µg/mL ( and ). The NP did not affect bacterial viability with the biofilm as evident by the less reduction in viability, suggesting its antivirulence property instead of bactericidal property. The excellent ability of Gemi-AgNP to inhibit and eradicate biofilm might be associated with its larger surface area and smaller particle size compared with the parent compound [Citation42]. This finding is in agreement with other similar studies, where conjugated NPs were found to be more potent and effective than the original drug molecule [Citation47,Citation48]. The exact mechanism of action of increased potency and biofilm inhibition in conjugated drug NPs is still unclear; therefore, future studies should be focussed on the evaluation of intracellular uptake to deal with intracellular infections.
Table 3. Biofilm eradication of gemifloxacin and Gemi-AgNPs.
Urease inhibition assay
The synthesized Gemi-AgNPs were also checked for in vitro urease inhibition potential to determine their activity against the urease enzyme. The results revealed that Gemi-AgNPs have significant activity against urease with an IC50 value of 57.4 ± 0.72 µg/mL. It has been reported that urease secreted by P. mirabilis increases the pH of the kidney and is responsible for several kidney diseases, such as infection stones, urolithiasis, acute pyelonephritis, and urinary tract infection [Citation49,Citation50].
Bacterial morphological changes under TEM
Cell wall damage after treatment with Gem-AgNPs was checked against three different bacterial strains via TEM. Literature revelled that the AFM, SEM, and TEM were used for morphological study of the bacterial cell wall and their damage after antibiotic treatment [Citation51–54]. In the current research, we first time study the bacterial cell morphology (K. pneumoniae, E. aerogenes, and E. coli) after treatment of Gem-AgNPs. The high affinity of Ag towards sulphur plays a vital role in the penetration of Gemi-AgNPs through the bacterial cell membrane via adhering to proteins in the cell membrane. The penetration of nanoconjugates into the cell disturbs protein functioning via binding with protein, DNA, and phosphorous, which increases the cell mortality rate. In the present study, 2 D TEM images of E. coli, K. pneumoniae, and E. aerogenes were produced. Detailed morphological studies of bacteria were conducted before and after treatment with Gemi-AgNPs. In the control group, typical healthy bacterial morphology was noticed; the regular nucleus and cytoplasm structure were determined, the cell wall and the membrane were observed as integrated. One of the most apparent findings is cytoplasm dissolutions and the abundance of vacuolar structures. Interestingly, cytoplasm dissolution is higher in the central parts of the majority of cells, and the cytoplasm content is drawn towards the periphery of the cell. Some cells were whole but they showed an electron-dense appearance. Another important ultrastructural finding is that the cells in intense contact with the nanoparticles have undergone the deformation of the membrane and wall structure. This change is generally in the form of outward curling and elongation. K. pneumonia (), E. aerogenes () and E. coli () control cells showed well-preserved cell membrane and wall structure. When cells of such bacterial strains were exposed to Gemi-Ag NPs at distinct concentrations, cellular damages appeared in many different ways. Completely emptied ghost cells are quite common. In some cells, membrane and wall damages and disorganization of cytoplasm were observed as in the case of K. pneumoniae and E. aerogenes (). A few cells exhibited cytoplasm condensation and electron-dense appearance. In general, nanoparticles are condensed in the cell membrane and wall as clusters and seen inside the cell in some regions. Conversely, moderate cell wall disruption was observed against E. coli (). Bacterial cell wall disruption by different bacterial strains is presented in .
Figure 12. Cell wall damage of K. pneumoniae. (a) Untreated K. pneumoniae at 500 nm and (b) at 1 µm was used as control, whereas (c) present the bacterial cell wall damage of K. pneumoniae after treatment with Gemi-AgNPs at 200 nm and (d) at 1 µm.
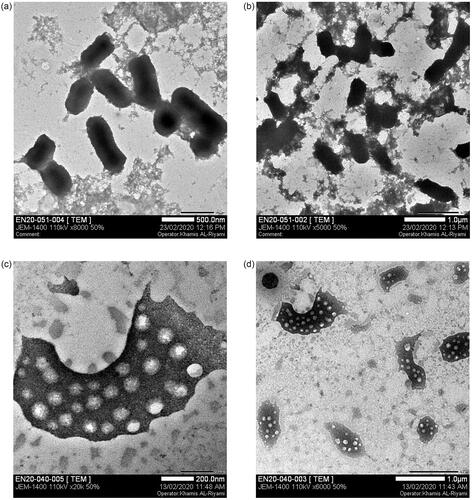
Conclusion
Since nanoparticles have a strong potential for solving the problem of microbial drug resistance, they are of great interest as an alternative to antibiotics. Gemifloxacin conjugated silver nanoparticles (Gemi-AgNPs) were synthesized using simple chemical methods. Synthesized AgNPs were found to be stable at all ranges of pH, and AgNP formation occurs at a high temperature and in extremely acidic and basic environments. The surface topology of Gemi-AgNPs was studied via AFM and TEM, which revealed that synthesized NPs were round oval in shape and have an average particle size of 22.23 ± 2 nm. The Fourier transform infra-red (FTIR) spectra demonstrated the interaction between the − OH and − NH2 functional moiety and the metal surface. The antibiofilm activity of synthesized NPs was performed against certain species of bacteria. Gemi-AgNPs exhibited cytotoxicity against K. pneumoniae and MRSA and were found to be less active against other bacterial strains. Our study has shown that these NPs reduce the bacterial growth of gram-positive and gram-negative pathogens that produce biofilm and the potential in various biomedical and environmental applications of silver nanoparticles synthesized. Additionally, the antibiofilm effect of silver nanoparticles on gram-negative bacteria was stronger than gram-positive bacteria. The researchers suggest that this difference may be due to the difference in composition on the cell wall. Confocal microscopic images revealed an increase in biofilm inhibition with the formation of NPs. A sufficient decrease in bacterial biofilms is attributed to the smaller size of NPs compared with the parent compound. Similarly, we observed cell wall, membrane, and cytoplasm damage. Morphological studies of cell wall damage of these bacteria via TEM revealed that Gemi-AgNPs significantly disrupt the cell wall of K. pneumoniae and E. aerogenes. The most important point here is that it is necessary to investigate the using potentials of them as an antimicrobial in low doses and safely. Especially, small amounts of silver nanoparticles combined use with existing antibiotics can significantly increase antimicrobial activity and prevent the development of multidrug resistance. Our findings have demonstrated in detail the ultrastructural effects of silver nanoparticles obtained via the chemical method in the cell, but it is very important to clearly identify which cellular metabolisms are used.
Author contributions
A.A.H. and A.K. conceived and designed the study. T.A., F.M., R.S., and A.I. performed most of the experiments, analyzed and interpreted the data, contributed to the drafting of the manuscript. M.K. and S.A. performed biological activities. K.A.R. performed J.U. and N.H. analyzed the data. A.A.K. and A.K. wrote the manuscript with inputs and comments from all co-authors. All authors approved the final version of the manuscript.
Acknowledgements
The authors also extend their appreciation to the Deanship of scientific research at King Khalid University for funding this work through the research groups program under Grant No. RGP.2/117/42. The authors have no other relevant affiliations or financial involvement with any organization or entity with a financial interest in or financial conflict with the subject matter or materials discussed in the manuscript apart from those disclosed.
Disclosure statement
All authors confirm that this article content has no conflict of interest.
Data availability statement
All data is included in the submission/manuscript file.
Additional information
Funding
References
- Jamrozik E, Selgelid MJ. Drug-resistant infection: causes, consequences, and responses. In: Ethics and drug resistance: collective responsibility for global public health. Cham: Springer; 2020. p. 3–18.
- Chen X, Schluesener H. Nanosilver: a nanoproduct in medical application. Toxicol Lett. 2008;176(1):1–12.
- Guo S, Xu C, Yin H, et al. Tuning the size, shape and structure of RNA nanoparticles for favorable cancer targeting and immunostimulation. Wiley Interdiscip Rev Nanomed Nanobiotechnol. 2020;12(1):e1582.
- Abeer MM, Rewatkar P, Qu Z, et al. Silica nanoparticles: a promising platform for enhanced oral delivery of macromolecules. J Control Release. 2020;326:544–555.
- Hamad A, Khashan KS, Hadi A. Silver nanoparticles and silver ions as potential antibacterial agents. J Inorg Organomet Polym. 2020;30(12):4811–4818.
- Cui H, Abdel-Samie MA-S, Lin L, et al. Application of antimicrobial-loaded nano/microcarriers in different food products. In: Application of nano/microencapsulated ingredients in food products. Academic Press: Elsevier; 2021. p. 469–517.
- Contera S. Nano comes to life: how nanotechnology is transforming medicine and the future of biology. Princeton, United States: Princeton University Press; 2019.
- Aqeel M, Ikram M, Asghar A, et al. Synthesis of capped Cr-doped ZnS nanoparticles with improved bactericidal and catalytic properties to treat polluted water. Appl Sci. 2020; 10: 2045–2055.
- Seifi H, Gholami T, Seifi S, et al. A review on current trends in thermal analysis and hyphenated techniques in the investigation of physical, mechanical and chemical properties of nanomaterials. J Anal Appl Pyrolysis. 2020;149:104840.
- Messaoudi O, Bendahou M. Biological Synthesis of Nanoparticles Using Endophytic Microorganisms: Current Development, Nanotechnology and the Environment, 2020; Mousumi Sen, IntechOpen, DOI:https://doi.org/10.5772/intechopen.93734. Available from: https://www.intechopen.com/chapters/73430
- Abdelnour SA, Alagawany M, Hashem NM, et al. Nanominerals: fabrication methods, benefits and hazards, and their applications in ruminants with special reference to selenium and zinc nanoparticles. Animals. 2021;11(7):1916.
- Pryshchepa O, Pomastowski P, Buszewski B. Silver nanoparticles: synthesis, investigation techniques, and properties. Adv Colloid Interface Sci. 2020; 284:102246.
- Jamaledin R, Yiu CK, Zare EN, et al. Advances in antimicrobial microneedle patches for combating infections. Adv Mater. 2020;32(33):2002129.
- Makabenta JMV, Nabawy A, Li C-H, et al. Nanomaterial-based therapeutics for antibiotic-resistant bacterial infections. Nature Reviews Microbiology 2021;19, 23–36.
- Jamshidifar E, Eshrati Yeganeh F, Shayan M, et al. Super magnetic niosomal nanocarrier as a new approach for treatment of breast cancer: a case study on SK-BR-3 and MDA-MB-231 cell lines. IJMS. 2021;22(15):7948.
- Durán-Iturbide NA, Díaz-Eufracio BI, Medina-Franco JL. In silico ADME/tox profiling of natural products: a focus on BIOFACQUIM. ACS Omega. 2020;5(26):16076–16084.
- Bamidele OP, Emmambux MN. Encapsulation of bioactive compounds by “extrusion” technologies: a review. Crit Rev Food Sci Nutr. 2021;61(18):3100–3119.
- Murei A, Ayinde WB, Gitari MW, et al. Functionalization and antimicrobial evaluation of ampicillin, penicillin and vancomycin with Pyrenacantha grandiflora baill and silver nanoparticles. Sci Rep. 2020;10(1):11596.
- Anwar A, Yi YP, Fatima I, et al. Antiamoebic activity of synthetic tetrazoles against Acanthamoeba castellanii belonging to T4 genotype and effects of conjugation with silver nanoparticles. Parasitology Research 2020; 119:1943–1954.
- Targhi AA, Moammeri A, Jamshidifar E, et al. Synergistic effect of curcumin-Cu and curcumin-Ag nanoparticle loaded niosome: enhanced antibacterial and anti-biofilm activities. Bioorg Chem. 2021;115:105116.
- Donga S, Chanda S. Facile green synthesis of silver nanoparticles using Mangifera indica seed aqueous extract and its antimicrobial, antioxidant and cytotoxic potential (3-in-1 system). Artif Cells Nanomed Biotechnol. 2021;49(1):292–302.
- Kavaz D, Umar H, Shehu S. Synthesis, characterization, antimicrobial and antimetastatic activity of silver nanoparticles synthesized from Ficus ingens leaf. Artif Cells Nanomed Biotechnol. 2018;46(sup3):S1193–S1203.
- Al‐Shidhani S, Rehman NU, Mabood F, et al. Quantification of incensole in three Boswellia species by NIR spectroscopy coupled with PLSR and cross-validation by HPLC. Phytochem Anal. 2018;29(3):300–307.
- Yadollahi M, Farhoudian S, Namazi H. One-pot synthesis of antibacterial chitosan/silver bio-nanocomposite hydrogel beads as drug delivery systems. Int J Biol Macromol. 2015;79:37–43.
- Fayaz AM, Balaji K, Girilal M, et al. Biogenic synthesis of silver nanoparticles and their synergistic effect with antibiotics: a study against gram-positive and gram-negative bacteria. Nanomedicine. 2010;6(1):103–109.
- Yang X, Yang J, Wang L, et al. Pharmaceutical intermediate-modified gold nanoparticles: against multidrug-resistant bacteria and wound-healing application via an electrospun scaffold. ACS Nano. 2017;11(6):5737–5745.
- Hedayati Ch M, Abolhassani Targhi A, Shamsi F, et al. Niosome-encapsulated tobramycin reduced antibiotic resistance and enhanced antibacterial activity against multidrug-resistant clinical strains of Pseudomonas aeruginosa. J Biomed Mater Res A. 2021;109(6):966–980.
- Mirzaie A, Peirovi N, Akbarzadeh I, et al. Preparation and optimization of ciprofloxacin encapsulated niosomes: a new approach for enhanced antibacterial activity, biofilm inhibition and reduced antibiotic resistance in ciprofloxacin-resistant methicillin-resistance Staphylococcus aureus. Bioorg Chem. 2020;103:104231.
- Li P, Li J, Wu C, et al. Synergistic antibacterial effects of β-lactam antibiotic combined with silver nanoparticles. Nanotechnology. 2005;16(9):1912–1917.
- McShan D, Zhang Y, Deng H, et al. Synergistic antibacterial effect of silver nanoparticles combined with ineffective antibiotics on drug resistant Salmonella typhimurium DT104. J Environ Sci Health C Environ Carcinog Ecotoxicol Rev. 2015;33(3):369–384.
- Zeleke D, Eswaramoorthy R, Belay Z, et al. Synthesis and antibacterial, antioxidant, and molecular docking analysis of some novel quinoline derivatives. J Chem. 2020;2020:1–16.
- Rusu A, Lungu I-A, Moldovan O-L, et al. Structural characterization of the millennial antibacterial (fluoro) quinolones—shaping the fifth generation. Pharmaceutics. 2021;13(8):1289.
- Thompson WG, Longstreth G, Drossman D, et al. Functional bowel disorders and functional abdominal pain. Gut. 1999;45(Supplement 2):II43–II47.
- Soman A, Honeybourne D, Andrews J, et al. Concentrations of moxifloxacin in serum and pulmonary compartments following a single 400 mg oral dose in patients undergoing fibre-optic bronchoscopy. J Antimicrob Chemother. 1999;44(6):835–838.
- Tischendorf J, Brunner M, Knobloch MJ, et al. Evaluation of a successful fluoroquinolone restriction intervention among high-risk patients: a mixed-methods study. PLOS One. 2020;15(8):e0237987.
- Girijan SK, Paul R, V J RK, et al. Investigating the impact of hospital antibiotic usage on aquatic environment and aquaculture systems: a molecular study of quinolone resistance in Escherichia coli. Sci Total Environ. 2020;748:141538.
- Jung J, Hwang SW, Kwak J, et al. Capsaicin binds to the intracellular domain of the capsaicin-activated ion channel. J Neurosci. 1999;19(2):529–538.
- Cormican MG, Jones RN. Antimicrobial activity and spectrum of LB20304, a novel fluoronaphthyridone. Antimicrob Agents Chemother. 1997;41(1):204–211.
- Farooq U, Ahmad T, Khan A, et al. Rifampicin conjugated silver nanoparticles: a new arena for development of antibiofilm potential against methicillin resistant Staphylococcus aureus and Klebsiella pneumoniae. Int J Nanomedicine. 2019;14:3983–3993.
- Iqbal E, Lim LB, Salim KA, et al. Isolation and characterization of aristolactam alkaloids from the stem bark of Goniothalamus velutinus (airy shaw) and their biological activities. J King Saud Univ Sci. 2018;30(1):41–48.
- Khan AK, Ahmed A, Hussain M, et al. Antibiofilm potential of 16-oxo-cleroda-3, 13(14) E-diene-15 oic acid and its five new γ-amino γ-lactone derivatives against methicillin resistant Staphylococcus aureus and Streptococcus mutans. Eur J Med Chem. 2017;138:480–490.
- Ahmed A, Khan AK, Anwar A, Ali SA, et al. Biofilm inhibitory effect of chlorhexidine conjugated gold nanoparticles against Klebsiella pneumoniae. Microb Pathog. 2016;98:50–56.
- Weatherburn M. Phenol-hypochlorite reaction for determination of ammonia. Anal Chem. 1967;39(8):971–974.
- Crane MJ, Jacoby LM, Cohen TA, et al. Coherent spin precession and lifetime-limited spin dephasing in CsPbBr3 perovskite nanocrystals. Nano Lett. 2020;20(12):8626–8633.
- Stewart S, Wei Q, Sun Y. Surface chemistry of quantum-sized metal nanoparticles under light illumination. Chem Sci. 2020;12(4):1227–1239.
- Link S, El-Sayed MA. Size and temperature dependence of the plasmon absorption of colloidal gold nanoparticles. J Phys Chem B. 1999;103(21):4212–4217.
- Ramos MADS, Da Silva PB, Spósito L, et al. Nanotechnology-based drug delivery systems for control of microbial biofilms: a review. Int J Nanomedicine. 2018;13:1179–1213.
- Sabaeifard P, Abdi-Ali A, Gamazo C, et al. Improved effect of amikacin-loaded poly(D,L-lactide-co-glycolide) nanoparticles against planktonic and biofilm cells of Pseudomonas aeruginosa. J Med Microbiol. 2017;66(2):137–148.
- Torzewska A, Wiewiura P, Brodecka D, et al. Potentially probiotic lactobacillus strains derived from food intensify crystallization caused by Proteus mirabilis in urine. Probiotics Antimicrob Proteins. 2021;13(2):441–452.
- Gaston JR, Johnson AO, Bair KL, et al. Polymicrobial interactions in the urinary tract: is the enemy of my enemy my friend? Infect Immun. 2021;89(4):e00652–20.
- Hartmann M, Berditsch M, Hawecker J, et al. Damage of the bacterial cell envelope by antimicrobial peptides gramicidin S and PGLa as revealed by transmission and scanning electron microscopy. Antimicrob Agents Chemother. 2010;54(8):3132–3142.
- Banner DJ, Firlar E, Jakubonis J, et al. Correlative ex situ and liquid-cell TEM observation of bacterial cell membrane damage induced by rough surface topology. Int J Nanomedicine. 2020;15:1929–1938.
- Campos JV, Assis OBG, Bernardes-Filho R. Atomic force microscopy evidences of bacterial cell damage caused by propolis extracts on E. coli and S. aureus. Food Sci Technol. 2020;40(1):55–61.
- Meincken M, Holroyd D, Rautenbach M. Atomic force microscopy study of the effect of antimicrobial peptides on the cell envelope of Escherichia coli. Antimicrob Agents Chemother. 2005;49(10):4085–4092.