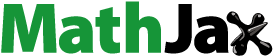
Abstract
Spinal cord injury (SCI) is a common pathology often resulting in permanent loss of sensory, motor, and autonomic function. Numerous studies in which stem cells have been transplanted in biomaterial scaffolds into animals have demonstrated their considerable potential for recovery from SCI. In the present study, a three-dimensional porous silk fibroin (SF) scaffold with a mean pore size of approximately 383 μm and nanofibrous structure was fabricated, the silk scaffold enabling the enhanced attachment and proliferation of bone marrow stromal cells (BMSCs). Investigation of its therapeutic potential was conducted by implantation of the nanofibrous SF scaffold seeded with BMSCs into a transected spinal cord model. Recovery of the damaged spinal cord was significantly improved after 2 months, compared with a non-nanofibrous scaffold, in combination with decreased glial fibrillary acidic protein (GFAP) expression and improved axonal regeneration at the site of injury. Furthermore, elevated Basso-Beattie-Bresnahan (BBB) scores indicated greatly improved hindlimb movement. Together, these results demonstrate that transplantation of neural scaffolds consisting of nanofibrous SF and BMSCs is an attractive strategy for the promotion of functional recovery following SCI.
Introduction
Spinal cord injury (SCI) is debilitating, causing severe motor and sensory paralysis [Citation1,Citation2] generally leading to life-long disability and loss of independence. After a primary injury of spinal cord tissue by direct mechanical force, a series of secondary events involving various pathological responses result in the substantial loss of cells, release of cytotoxic factors, and cystic cavitation [Citation3]. Despite advances in clinical medicine, there is no effective treatment for SCI due to neuronal loss and limited axonal regeneration [Citation4]. The transplantation of a stem cell-scaffold composite is a promising strategy for SCI, aiming to recover neural function.
Cell transplantation has been widely studied to examine its feasibility and therapeutic potential [Citation5]. The types of cells used for implantation include neural stem cells [Citation6], olfactory ensheathing cells (OECs) [Citation7], Schwann cells [Citation8], embryonic stem cells [Citation9], and bone marrow stromal cells (BMSCs) [Citation10]. It has already been demonstrated that cell transplantation can promote axonal growth and functional recovery in rat SCI models [Citation10,Citation11]. The transplanted stem cells differentiate into neuronal or neural support cells, secrete trophic factors, thereby enhancing the microenvironment surrounding the nerve injury and promoting repair [Citation12]. Of all these cell types, bone marrow stromal cells are considered among the most promising for therapeutic applications in SCI, mostly due to their favourable ethical profile, safety, and easy availability [Citation13].
However, it has been found that transplantation of BMSCs alone following SCI results in low cell viability and a limited capacity for axonal regeneration [Citation14,Citation15]. More positive outcomes may be observed when cells are implanted with a biomaterial scaffold [Citation16]. SCI repair has been explored using a variety of synthetic and natural materials that inhibit cell apoptosis, reduce glial scar formation, and alter the local microenvironment [Citation17]. Common synthetic scaffolds used for SCI repair include polylactic acid [Citation18], poly(lactic-co-glycolic acid) [Citation19], polycaprolactone [Citation20], polyethylene glycol [Citation21], although the clinical application of these materials is restricted due to their poor cell affinity and harmful degradation products. Many natural materials, including collagen [Citation22] and chitosan [Citation23] have also been investigated in SCI repair studies. Although considerable progress has been achieved in treating SCI with these materials, appropriate control of degradation and achieving an ideal environment remains challenging [Citation24,Citation25].
Silk fibroin, derived from cocoons, has been widely shown to be beneficial for the adhesion and growth of nerve cells, and axonal regeneration in SCI [Citation26]. Silk-based scaffolds have a number of unique characteristics, such as their biocompatibility, porosity, biodegradability, and permeability that assist the diffusion of ions, nutrients, and waste products [Citation27]. Furthermore, an ideal scaffold for SCI should provide a three-dimensional pore structure and mimic natural extracellular matrix (ECM), providing a more permissive environment for cell survival, growth, migration, and differentiation [Citation28–30]. A few studies have found that porous silk scaffolds support nerve cell adhesion and proliferation, while their implantation can reduce cavity formation and promote axonal regeneration and myelination [Citation31,Citation32]. Silk scaffolds with a nanofibrous architecture can provide nanoscale cues that induce oriented growth and neuronal differentiation of stem cells in vitro [Citation33], and direct and enhance axonal regeneration following SCI in vivo [Citation34].
We have previously reported our experience of a three-dimensional SF scaffold with a nanofibrous structure in which improved cell adhesion and proliferation were observed [Citation35]. In the present study, this nanofibrous SF scaffold was optimised for cell growth, seeded with BMSCs prior to transplantation into a Sprague-Dawley (SD) rat model of SCI. We found that the BMSCs adhered well to the SF scaffold and proliferated in vitro in scaffolds both with and without the nanofibrous structure. Rats in which BMSC-seeded nanofibrous SF scaffolds were implanted displayed a higher expression of neurofilament (NF-200) and a lower expression of the glial cell marker glial fibrillary acidic protein (GFAP) than those that received a non-nanofibrous BMSC-seeded SF scaffold. NF-200 is principally located in the axon and only rarely within the cell body. It plays an important role in neuronal differentiation, axonal regeneration, and the plasticity of neural structure and function. Glial scars are considered a physical barrier preventing the regeneration of nerve fibres growing into damaged tissue. Rats treated with BMSC-seeded nanofibrous SF scaffolds also displayed superior hindlimb movement, as the Basso, Beattie, and Bresnahan (BBB) scores suggest. Therefore, nanofibrous SF scaffolds combined with BMSCs represent a promising strategy for SCI.
Materials and methods
Isolation and culture of bone marrow stromal cells
As we described in a previous report [Citation32], all animal procedures were approved by the Institutional Animal Care and Use Committee, Soochow University. BMSCs were extracted from 4-week-old SD rats (Laboratory Animal Centre of Soochow University, Suzhou, China) weighing 100–150 g. The cells were cultured in standard medium composed of low-glucose Dulbecco's modified Eagle medium (L-DMEM, Gibco, Gaithersburg, MD) supplemented with 10% foetal bovine serum (FBS, Gibco), 100 U/mL penicillin, and 100 μg/mL streptomycin in an atmosphere continaing 5% CO2 at 37 °C. The culture medium was firstly replaced after 24 h, then after every 3 days. Cultures of BMSCs were dissociated using 0.25% trypsin-ethylene diamine tetraacetic acid (EDTA) solution (Sigma, St. Louis, MO) when 80–90% confluent, then subcultured at a ratio of 1:2. BMSCs at passage 3 were used for transplantation.
Preparation and characterisation of nanofibrous SF scaffold
Preparation of nanofibrous SF scaffold
The nanofibrous SF scaffold was prepared as described previously [Citation35]. Briefly, raw silk (Jiangsu SoHo International Group Corp., Nantong, China.) was degummed using 0.05% (w/v) Na2CO3, then directly dissolved in 4% CaCl2-formic acid solution, yielding a 10% (w/v) silk nanofiber solution [Citation36]. Sodium chloride particulates (particle size: 300–400 µm) were added into the silk solution at a weight ratio of sodium chloride to silk of 10:1. After evaporation of the solvent, the silk/porogen composite was immersed in water for 48 h to completely remove the porogen. The hydrated silk scaffold was frozen at −20 °C for 24 h then lyophilised for 72 h to generate SF scaffolds with a nanofibrous structure, termed as nanofibrous SF scaffolds. As a control, SF scaffolds without a nanofibrous structure were prepared from Hexafluoroisopropanol (HFIP) in accordance with a previous report [Citation37], termed as non-nanofibrous SF scaffold.
Characterisation of nanofibrous SF scaffold
The morphology of silk scaffolds was observed by scanning electron microscopy (SEM, 8100, Hitachi, Tokyo, Japan) at a magnification of 30× and 5000× after sputter-coating with gold.
Fourier transform infra-red (FTIR) spectra of the silk scaffolds were obtained using a Magna spectrometer (Nicolet 5700, Unites States of America) over the spectral region 400–4000 cm−1. Sample crystallinity was defined using the following equation [Citation38]:
where A1260cm−1: Absorbance at 1260 cm−1; A1235cm−1: Absorbance at 1235 cm−1
Cultivation and observation of BMSCs on SF scaffold
Cultivation of BMSCs on SF scaffold
Two mm-thick SF scaffolds were cut into 3 mm diameter discs, suitable for placing in 96-well plates. The discs were sterilised with 60Co γ-irradiation at a dose of 50 kGy prior to culture with BMSCs. The scaffolds were then soaked in culture medium for 2 h. Passage 3 BMSCs were cultured in Petri dishes in DMEM supplemented with 10% FBS and streptomycin-penicillin. When 80–90% confluent, the cells were detached and seeded onto the SF scaffold discs placed in the wells of a 96-well plate. A total of 1 × 105 cells suspended in 10 μl DMEM medium supplemented with serum was seeded onto each scaffold for examination by confocal laser scanning microscopy (CLSM) and proliferation assay. The culture medium was replaced every 3 days.
Scanning electron microscopy (SEM)
The morphology of the cells on the different scaffolds was observed by SEM (8100, Hitachi, Tokyo, Japan) at a magnification of 1000×. Briefly, the cell-seeded scaffolds were washed with PBS three times. Prior to dehydration through an alcohol gradient (50%, 70%, 80%, 90%, and 100%), each sample was fixed in 4% paraformaldehyde for 30 min. After sputtered coating with gold, the scaffolds were inspected with the SEM at 3 kV. The scaffold was inspected at several different random regions.
Confocal laser scanning microscopy
The morphology of the cells on the experimental and control SF scaffolds was evaluated using confocal laser scanning microscopy (Olympus, Nagano, Japan). After 1 week of cell culture, the scaffolds were washed with PBS three times and then fixed in 4% paraformaldehyde for 30 min. The cells were permeabilized with 0.1% Triton X-100, then incubated with TRITC-Phalloidin (Sigma, USA) at room temperature for 2 h. Confocal laser scanning microscopy was used to obtain representative fluorescence images of the stained samples. After culture in 96-well plates for 1 week, scaffolds seeded with BMSCs were transplanted.
Cell proliferation assay
A CCK8 assay was used to analyse cell proliferation on the SF scaffolds. Cell-seeded scaffolds were incubated for 3 h with 1 ml of fresh DMEM/F12 medium at 37 °C within an atmosphere of 5% CO2 after the addition of 10 μl of CCK8 reagent (Sigma, USA). A 100 μl aliquot of the culture medium-CCK8 mixture was transferred to another 96-well plate and the absorbance at 450 nm measured using a microplate reader. Scaffolds with medium but without cells were used to measure the background absorbance. Cell proliferation was determined after 1, 3, 6, 9, and 12 days of culture.
Preparation of transected SCI rats
Adult male SD rats (Laboratory animal of Soochow University, Suzhou, China) with a bodyweight of 200–230 g were used for surgical procedures (n = 30) [Citation32]. Rats were utilised in accordance with the guide for care and use of laboratory animals of the National Institutes of Health and approved by the Animal Care and Use Committee of Soochow University. The rats were anaesthetised with sodium pentobarbital (50 mg/kg body weight) and the T9–T11 vertebral body exposed after creating an incision in the dorsal skin and splitting the muscle tissue. Laminectomy was performed at the T9–T11 level to expose the spinal cord, and a 3 mm segment was transected and removed. After hemostasis, a nanofibrous or non-nanofibrous SF scaffold seeded with 5 × 105 cells suspended in 10 μl serum-supplemented medium was implanted into the site of the lesion (n = 10 for each group). The muscle layers and skin were individually sutured. To prevent infection, penicillin (20,000 U/kg) was administered by intramuscular injection daily after surgery for 3 days. The bladder was emptied manually twice daily until recovery of autonomic micturition.
Hindlimb behavioral tests
Functional recovery was measured using the Basso-Beattie-Bresnahan (BBB) locomotor rating scale [Citation39], which ranges from 0 to 21 points, 0 points indicating no spontaneous hindlimb movement and 21 points indicating normal movement. Hindlimb locomotion was evaluated on days 1, 7, 14, 28, 42, and 64 following SCI. During the evaluation, the rats were placed in an open field and observed for 5 min at each time point independently by two observers who were blind to the experimental protocol. Observers measured each rat twice. Final scores were obtained by averaging the scores of both limbs. At least eight rats were tested at each time point in each treatment group.
Tissue processing, histology, and immunofluorescence staining
Heart perfusion was performed on 8 animals in each group 8 weeks postoperatively after SCI, after which the spinal cord was harvested at the site of injury. A 9-mm segment of spinal cord at the centre of the implantation/injury site was dissected following anaesthesia and embedded in 1.5% low melting-temperature agarose in PBS. Twenty μm-thick sections were sliced from the resultant block and mounted on gelatin-coated slides for haematoxylin and eosin (H&E) and immunofluorescence staining.
H&E staining was performed as described previously [Citation32]. Spinal cord tissue sections were rehydrated in 0.1 mol/l PBS, blocked in 5% bovine serum albumin (BSA) and 0.3% Triton X-100 for 1 h in preparation for immunofluorescent labelling. The sections were stained with appropriate antibodies, including against glial fibrillary acetic protein (GFAP, Service-Bio) for identification of glial scars, mouse anti-NF-200 (Service-Bio) for the detection of axonal regeneration, against growth-associated protein-43 (GAP-43) to evaluate axonal growth, and mouse anti-β‐tubulin III (Service-bio). The nuclei were visualised using Hoechst 33258 (1:100 dilution, Service-Bio). A confocal laser scanning microscope (FV10 inverted microscope, Olympus, Nagano, Japan) was used to obtain representative fluorescence images of the stained samples. Single-channel immunofluorescence images were converted to greyscale and the area of staining above a threshold value was recorded for different tissue compartments using NIH ImageJ (v.1.51) software [Citation32]. For immunohistochemical staining, Image-Pro Plus (IPP, Media Cybernetics, USA) software was used to quantitatively measure the integrated optical density (IOD), IOD = density (mean) × area.
Statistical analyses
Image pro plus 6.0 Image analysis software was used to gauge integral absorbance (IA) values of positive staining in each group of immunohistochemical digital images. Values are presented as means and SEM. Statistical analysis was performed using SPSS v17.0 software. The significance of differences was determined using a one-way ANOVA with a post-hoc Dunnett's test. p < .05 was considered significant.
Results
Isolation and observation of BMSCs
BMSCs are among the most promising cells for neural tissue engineering of SCI [Citation40]. In the present study, MSCs were successfully isolated from the marrow of the rat femurs and tibias. BMSCs were cultured to passage 3 and observed by light microscopy. As shown in , the cells were mostly bipolar and tripolar, consistent with the typical shape of BMSCs. The BMSCs were immunohistologically positive for CD29 and CD90, and negative for CD34 and CD45 [Citation41].
Observation of 3 D SF scaffolds
Both macropores and microscopic structures are equally important in neural scaffolds as environments suitable for cell and tissue growth. SF scaffolds with a porous structure were prepared using salt particles that created pores in the range 300–500 μm with a mean pore diameter of 383 ± 56 μm, as displayed in . Closer observation of the pores indicated the presence of a substructure surface suggestive of a nanofibrous structure () originating from the nanofibrils preserved from the dissolution phase [Citation42]. The FTIR spectrum of the SF porous scaffold is presented in which demonstrates absorption peaks at 1620 and 1515 cm−1, with a shoulder at 1265 cm−1, attributed to the β-sheet conformation [Citation38]. The crystallinity index, calculated from the amide III band, was approximately 25.4%, close to that of degummed silk [Citation38], suggesting the SF scaffold was highly crystalline.
Observation of BMSC growth on SF scaffolds
Successful treatment of a defect with cells depends mostly on the precise transplantation of cells to the site of injury. In the present study, the influence of the nanofibrous structure on cell adhesion and proliferation was evaluated in in vitro cell culture, aiming to identify a scaffold suitable for the loading of BMSCs. As a control, salt-leached SF scaffolds without a nanofibrous structure were prepared from HFIP. Cell morphology and their growth on SF scaffolds after 3 and 7 days of culture as observed by confocal microscopy and SEM are displayed in . BMSCs proliferated normally on the surface of the SF scaffolds from days 3–7. Cell adhesion and uniform spreading were observed on the surfaces of SF scaffolds after 3 days, with clearly apparent proliferation by day 7. Compared with non-nanofibrous SF scaffold (), SEM images indicate that the cells on the nanofibrous SF scaffold () displayed superior spreading and sheet-like structures, suggesting that the nanofibrous SF biomaterials displayed better biocompatibility. A CCK8 assay was conducted to evaluate cell proliferation behaviour. As demonstrated in , the proliferation of BMSCs continued throughout the 12 day culture period, indicating that room sufficient for cell proliferation was provided by the porous structure of the SF scaffolds. It was noted that the cell proliferation behaviour varied among the different scaffolds. Higher cell numbers were achieved on the SF scaffolds with a nanofibrous structure, suggesting superior cell compatibility. In the following experiment, BMSCs were seeded on the SF scaffolds to further evaluate SCI repair in vivo.
Figure 3. Confocal microscopy (A1, A2, B1, B2) and SEM (A3, B3) images of BMSCs on nanofibrous SF scaffold at day 3 (A1), and day 7 (A2) and (A3); and non-nanofibrous SF scaffold at day 3 (B1), and day 7 (B2) and (B3). TRITC labelled phalloidin (red) stained F-actin, DAPI (blue) stained cell nuclei on the SF scaffolds. Cell Counting Kit-8 (CCK-8) assay demonstrating the difference in the rate of proliferation of BMSCs on different scaffolds (C). BMSCs seeded on nanofibrous SF scaffolds (group B) proliferated more rapidly than on the non-nanofibrous SF scaffold (group A). Difference between Group A and Group B at different culture times were compared. *statistically significant p < .05.
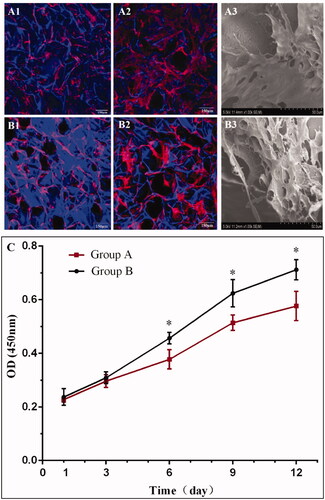
Transplantation of cell-seeded nanofibrous scaffolds promoted neuronal regeneration
To assess the effect of nerve injury repair, nanofibrous and non-nanofibrous SF scaffolds seeded with BMSCs were implanted into the site of SCI. As displayed in , the spinal cord of the rats was entirely exposed after which it was transected, creating a 3-mm gap representing an SCI. An SF scaffold seeded with BMSCs was placed at the site of injury ().
Figure 4. Spinal cord of rats was exposed (A), and a 3-mm SF scaffold seeded with BMSCs implanted into an SCI that was subsequently created (B). BBB scores indicate the significantly increased motor functional recovery of hindlimbs after the implantation of nanofibrous SF scaffold (C). Difference was considered statistically significant when *p < .05 compared with the SCI group, #p < .05 compared with the non-nanofibrous SF scaffold group.
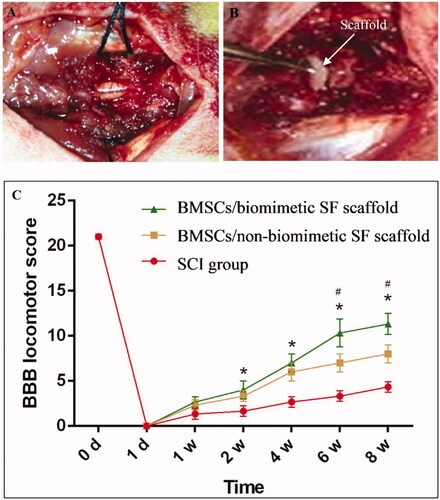
BBB scores were calculated to evaluate motor functional recovery of the hindlimbs following SCI, as shown in . Recovery of motor function was observed from weeks 1 to 4 following surgery, with significant differences observed between the two groups. The BBB score of the non-nanofibrous SF group was 2.4 at week 1, increasing to 6.1 at week 4 and 8.1 at week 8. Compared with the non-nanofibrous SF group seeded with BMSCs, the BBB score of the BMSC-seeded nanofibrous SF group was significantly greater, 2.5 at week 1, 6.83 at week 4, and 11.3 at week 8. More importantly, the recovery of motor function did not stop at the end of the observation period, as projected by the continual increase in BBB score in the two groups. Therefore, transplantation of a nanofibrous SF scaffold seeded with BMSCs could significantly enhance the recovery of motor function following SCI.
Immunofluorescence staining
Immunofluorescent staining indicated that axons regenerated well in the BMSC-seeded SF scaffolds transplanted into rats while scar tissue and a severe cavity were formed in the Control group. This was also clearly observed by immunohistochemical staining.
The SF scaffold was selected for transplantation into a spinal cord injury because of its outstanding bioactivity. We aimed to confirm the capability of the nanofibrous SF scaffold seeded with BMSCs to prevent glial scar formation and promote axonal regeneration after spinal cord injury in rats. The rats were sacrificed and the spinal cord sections prepared for immunofluorescence staining 8 weeks post-surgery. As indicated in , compared with the non-nanofibrous SF scaffold group (), a small degree of GFAP expression was observed at the centre of the injury of the nanofibrous SF scaffold group (). Furthermore, in the non-nanofibrous SF scaffold group, NF-positive nerve fibres were observed at the rostral and the caudal regions close to the lesion, but only rarely within the centre of the injury (). However, significantly more NF-positive nerve fibres were found within the lesion treated with the nanofibrous SF scaffold group (), indicating that axons had regenerated.
Figure 5. Double immunostaining of the site of injury of spinal cords for astrocytes (GFAP, green) and axons (neurofilament-200, NF-200, red). Compared with BMSCs seeded on the non-nanofibrous SF scaffold (Group A), the BMSC-seeded nanofibrous SF scaffold (Group B) displayed lower positive glial area and higher NF positive axons. Comparison of the fluorescence area of NF-200 positive fibres (C) and GFAP positive area (D) in the rostral (a1, b1), centre (a2, b2), and caudal (c1, c2) of the injury/scaffold site. Difference was considered statistically significant when *p < 0.05.
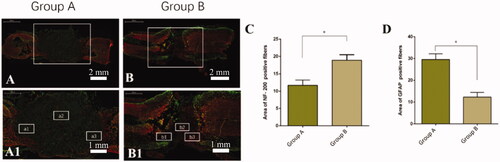
The spinal cord tissue was also stained with haematoxylin and eosin (H&E), indicating that the silk scaffolds provided a physical support for tissue regeneration in the area of SCI. The scar cavity in the nanofibrous SF scaffold group () was smaller than that in the non-nanofibrous group (), likely due to the enhanced cell proliferation in nanofibrous SF scaffold (). To assess neurite growth in the SCI, GAP-43 was evaluated by immunohistochemistry. Treatment of the resected tissue with the nanofibrous SF scaffold group () significantly decreased GAP-43 expression compared with the non-nanofibrous group (). Glial scars are physical barriers that prevent nerve regeneration following SCI. Immunofluorescence staining for GFAP was performed to evaluate the glial scar formation following SCI. In the nanofibrous SF scaffold treated rats, a dramatic reduction in GFAP was observed compared with the non-nanofibrous group ().
Figure 6. Spinal cord injury with HE staining, indicating that scar tissue in the nanofibrous SF scaffold group (B) was less than in the non-nanofibrous SF scaffold group (A). Transplantation of the nanofibrous SF scaffold group integrated well with the existing spinal cord, with less scar tissue (B). There was partial breakdown of the transplant in Group A, with a cavity observed within the spinal cord. Immunohistochemical staining was conducted to detect the expression of GAP-43 (C, D) and GFAP (E, F) 8 weeks after transplantation. Significant differences were observed in the absorbance value ratios for GAP-43 and GFAP in each group (p < .05), IOD: Integrated Optical Density, Control: Non-nanofibrous SF scaffold, BMSCs/Tussah SF: Nanofibrous SF scaffold, bar = 100 μm.
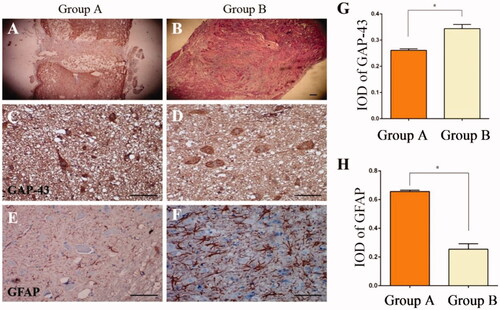
Discussion
Acute spinal cord injury typically results in discontinuation of the function of neuronal axons, blood vessels, and cell membranes. Within seconds of spinal cord injury, spinal cord tissues suffer ischaemic edoema and nerve cell membrane rupture, combined with ionic dysregulation due to Na+ and Ca2+ influx, followed by destruction of the spinal microvascular system [Citation43]. Reactive cytotoxic substances are released into the injured area, resulting in secondary spinal cord injury. During the chronic stage, the injured tissue becomes consolidated into fibrotic scar tissue surrounded by a dense rim of reactive astrocytes within the surviving white and grey matter, while voids form around it.
Silk fibroin is a degradable natural material that is safe, non-toxic with controllable degradation in vivo with excellent mechanical, physical, and chemical properties. The silk-based materials used in the present study have unique mechanical properties [Citation35]. In previous studies, we have shown that SF scaffolds exhibit good biocompatibility towards nerve cells, supporting cell adhesion, growth, proliferation, and differentiation [Citation35,Citation44]. In particular, the structure of SF nanofibers provides the capability to guide the direction of olfactory ensheathed cell migration, significantly increasing the speed and distance of travel, and supporting the expression of characteristic factors and proteins, including nerve growth factor receptor p75 and glial fibrillary acidic protein [Citation45]. Therefore, the nanofibrous structure of an SF scaffold can be designed for enhanced biocompatibility in neural applications [Citation46,Citation47]. In the current study, an SF scaffold with a 3 D porous and nanofibrous structure was designed for superior cell adhesion and proliferation to allow implanted cell-seeded scaffolds to promote SCI repair [Citation6].
The optimised 3 D porous silk scaffold is a promising design because it possesses the space required for cell proliferation and new tissue formation [Citation48]. The specific requirements for micro-porosity and nanostructure, in addition to the physical and chemical properties of tissue-engineering scaffolds, depend on the injured tissue. The specific nanostructure of SF scaffolds is challenging to manufacture for optimal neural tissue development due to the complexity of the scaffold architecture [Citation49]. For example, electrospinning is commonly used to prepare SF nanofiber mats, but the resulting SF 2 D mats are unsuitable for physiological tissue formation [Citation50]. In addition, it has previously been reported that SF nanofibers can be fabricated through self-assembly techniques to prepare ECM-mimetic scaffolds which display improved cell growth and proliferation [Citation47]. Recently, we reported methods for the fabrication of SF nanofibers capable of the construction of 3 D porous SF scaffolds with a nanofibrous structure [Citation35] and therefore a promising candidate device for cell loading and implantation for the repair of SCI. The in vivo study reported here demonstratess that the transplantation of BMSCs within the nanofibrous SF scaffold into a transected lesion promoted improvements in locomotion and tissue repair, including axonal regeneration, in rats with SCI. These results suggest that the nanofibrous SF scaffolds not only provide the necessary mechanical support for implanted BMSCs, they also promote the secretion of a number of neurotrophic factors required for locomotor recovery and axonal regeneration in injured spinal cords in rats.
Neurofilament-200 (NF-200) is a cytoskeletal protein characteristic of neurons and an important component of axons. In pathological conditions such as ischaemia and trauma, NF-200 disintegrates and depolymerises, destroying the cell structure and causing disruption to neural connections and neuronal death, manifested as a loss of neurological function. NF-200 is restored during nerve structure regeneration, and its expression is conspicuous [Citation51]. In the present study, spinal cord tissue junctions were observed after BMSCs + SF scaffold grafting using confocal microscopy by immunofluorescence staining. It was found that the grafts exhibited excellent compatibility with spinal cord tissue, and the expression of NF-200 was apparent in the junction region, with NF-200 immunoreactive axons entering the scaffold. Functional scoring results indicate that BMSCs combined with 3 D SF scaffolds promoted axonal regeneration and nerve reparation.
GFAP is a marker of astrogliosis and astrocyte activation commonly used in several conditions involving cerebral ischaemia [Citation52]. To ascertain whether the increase in nerve fibres within the centre of the injury was also related to the development of glial scarring, we also performed immunofluorescence staining for GFAP. Through dynamic investigation by immunohistochemical staining, significantly increased levels of GFAP were observed within the spinal cord lesion after spinal cord injury, while treatment with the BMSC-seeded nanofibrous SF scaffold inhibited GFAP expression. Greater expression was observed in the BMSC-seeded non-nanofibrous SF scaffold group. Thus, glial scar formation was lower in the BMSCs + nanofibrous SF scaffold, allowing axons to grow into the injured area.
Conclusions
In the present study, SF nanofibrils were directly extracted from native silk by solvation with CaCl2/formic acid. The resultant SF nanofibril solution was processed into a porous SF scaffold having an ECM-mimetic nanofibrous structure. Compared with a non-nanofibrous SF scaffold, the nanofibrous SF scaffold displayed superior cell adhesion and proliferation in in vitro cell experiments. Nanofibrous SF scaffolds loaded with BMSCs allowed regeneration in complete transverse SCI in vivo. The combination of nanofibrous SF scaffold seeded with BMSCs, which displayed good compatibility with BMSCs in vitro and improved neuronal regeneration after SCI in vivo, is therefore promising for clinical applications in SCI.
Authors’ contributions
XHW and XCT contributed to the experimental design, data interpretation and manuscript writing; XHW and XL performed the experiments, collected the data and wrote the manuscript; YXS, JZQ and TTD provided financial support, data analysis and interpretation, and manuscript writing. All the authors approved the final manuscript to be submitted.
Disclosure statement
No potential conflict of interest was reported by the author(s).
Data availability statement
The data that support the findings of this study are available from the corresponding author, [TTD], upon reasonable request.
Additional information
Funding
References
- Goel A. Stem cell therapy in spinal cord injury: hollow promise or promising science? J Craniovertebr Junction Spine. 2016;7(2):121–126.
- Tan JW, Wang KY, Liao GJ, et al. Neuroprotective effect of methylprednisolone combined with placenta-derived mesenchymal stem cell in rabbit model of spinal cord injury. Int J Clin Exp Pathol. 2015;8(8):8976–8982.
- Ahuja CS, Wilson JR, Nori S, et al. Traumatic spinal cord injury. Nat Rev Dis Primers. 2017;3:17018.
- Ahuja CS, Nori S, Tetreault L, et al. Traumatic spinal cord injury-repair and regeneration. Neurosurgery. 2017;80(3S):S9–S22.
- Lu P. Stem cell transplantation for spinal cord injury repair. Prog Brain Res. 2017;231:1–32.
- Farrag M, Leipzig ND. Subcutaneous maturation of neural stem cell-loaded hydrogels forms region-specific neuroepithelium. Cells. 2018;7(10):173.
- Gilmour AD, Reshamwala R, Wright AA, et al. Optimizing olfactory ensheathing cell transplantation for spinal cord injury repair. J Neurotrauma. 2020;37(5):817–829.
- Cerqueira SR, Lee YS, Cornelison RC, et al. Decellularized peripheral nerve supports Schwann cell transplants and axon growth following spinal cord injury. Biomaterials. 2018;177:176–185.
- Shroff G, Gupta R. Human embryonic stem cells in the treatment of patients with spinal cord injury. Ann Neurosci. 2015;22(4):208–216.
- Ritfeld GJ, Nandoe Tewarie RD, Vajn K, et al. Bone marrow stromal cell-mediated tissue sparing enhances functional repair after spinal cord contusion in adult rats. Cell Transplant. 2012;21(7):1561–1575.
- Johnson PJ, Tatara A, Shiu A, et al. Controlled release of neurotrophin-3 and platelet-derived growth factor from fibrin scaffolds containing neural progenitor cells enhances survival and differentiation into neurons in a subacute model of SCI. Cell Transplant. 2010;19(1):89–101.
- Gong Z, Xia KS, Xu AK, et al. Stem cell transplantation: a promising therapy for spinal cord injury. Curr Stem Cell Res Ther. 2020;15(4):321–331.
- Wright KT, El Masri W, Osman A, et al. Concise review: bone marrow for the treatment of spinal cord injury: mechanisms and clinical applications. Stem Cells. 2011;29(2):169–178.
- Gransee HM, Zhan WZ, Sieck GC, et al. Localized delivery of brain-derived neurotrophic factor-expressing mesenchymal stem cells enhances functional recovery following cervical spinal cord injury. J Neurotrauma. 2015;32(3):185–193.
- Nandoe Tewarie RD, Hurtado A, Levi AD, et al. Bone marrow stromal cells for repair of the spinal cord: towards clinical application. Cell Transplant. 2006;15(7):563–577.
- White SV, Czisch CE, Han MH, et al. Intravenous transplantation of mesenchymal progenitors distribute solely to the lungs and improve outcomes in cervical spinal cord injury. Stem Cells. 2016;34(7):1812–1825.
- Straley KS, Foo CWP, Heilshorn SC. Biomaterial design strategies for the treatment of spinal cord injuries. J Neurotrauma. 2010;27(1):1–19.
- Liu ZH, Huang YC, Kuo CY, et al. Docosahexaenoic acid-loaded polylactic acid core-shell nanofiber membranes for regenerative medicine after spinal cord injury: in vitro and in vivo study. IJMS. 2020;21(19):7031.
- Wang XY, Li B, Fan JJ, et al. Novel nanoformulated combination of Se and CeO2 particles loaded polylactic-co-glycolic acid vesicle to improved anti-inflammation and auto-regenerative for the treatment and care of spinal cord injury. Appl Organomet Chem. 2021;35(8):e6269.
- Zhang D, Wang Q, Wang S, et al. Astragoloside IV loaded polycaprolactone membrane repairs blood spinal cord barrier and recovers spinal cord function in traumatic spinal cord injury. J Biomed Nanotechnol. 2019;15(4):799–812.
- Yari-Ilkhchi A, Ebrahimi-Kalan A, Farhoudi M, et al. Design of graphenic nanocomposites containing chitosan and polyethylene glycol for spinal cord injury improvement. RSC Adv. 2021;11(33):19992–20002.
- Zou YL, Ma DZ, Shen H, et al. Aligned collagen scaffold combination with human spinal cord-derived neural stem cells to improve spinal cord injury repair. Biomater Sci. 2020;8(18):5145–5156.
- Chedly J, Soares S, Montembault A, et al. Physical chitosan microhydrogels as scaffolds for spinal cord injury restoration and axon regeneration. Biomaterials. 2017; 138:91–107.
- Zhang QZ, Shi B, Ding JX, et al. Polymer scaffolds facilitate spinal cord injury repair. Acta Biomater. 2019; 88:57–77.
- Shekh MI, Amirian J, Stadler FJ, et al. Oxidized chitosan modified electrospun scaffolds for controllable release of acyclovir. Int J Biol Macromol. 2020; 151:787–796.
- 46th ESAO Congress 3–7 September 2019 Hannover, Germany Abstracts. Int J Artif Organs. 2019;42:386–474.
- Sun WZ, Gregory DA, Tomeh MA, et al. Silk fibroin as a functional biomaterial for tissue engineering. IJMS. 2021;22(3):1499.
- Amirian J, Zeng Y, Shekh MI, et al. In-situ crosslinked hydrogel based on amidated pectin/oxidized chitosan as potential wound dressing for skin repairing. Carbohydr Polym. 2021; 251:117005.
- Jung A, Makkar P, Amirian J, et al. A novel hybrid multichannel biphasic calcium phosphate granule-based composite scaffold for cartilage tissue regeneration. J Biomater Appl. 2018;32(6):775–787.
- Amirian J, Linh NT, Min YK, et al. Bone formation of a porous gelatin-pectin-biphasic calcium phosphate composite in presence of BMP-2 and VEGF. Int J Biol Macromol. 2015; 76:10–24.
- You KM, Chang HZ, Zhang F, et al. Cell-seeded porous silk fibroin scaffolds promotes axonal regeneration and myelination in spinal cord injury rats. Biochem Biophys Res Commun. 2019;514(1):273–279.
- Hu Y, Zhang F, Zhong W, et al. Transplantation of neural scaffolds consisting of dermal fibroblast-reprogrammed neurons and 3D silk fibrous materials promotes the repair of spinal cord injury. J Mater Chem B. 2019;7(47):7525–7539.
- Qing HB, Jin GR, Zhao GX, et al. Heterostructured silk-nanofiber-reduced graphene oxide composite scaffold for SH-SY5Y cell alignment and differentiation. ACS Appl Mater Interfaces. 2018;10(45):39228–39237.
- You RC, Zhang Q, Li XF, et al. Multichannel bioactive silk nanofiber conduits direct and enhance axonal regeneration after spinal cord injury. ACS Biomater Sci Eng. 2020;6(8):4677–4686.
- Zhong WT, Hu YN, Li J, et al. In vitro biocompatibility study of a water-rinsed biomimetic silk porous scaffold with olfactory ensheathing cells. Int J Biol Macromol . 2019; 125:526–533.
- Zhang F, You XR, Dou H, et al. Facile fabrication of robust silk nanofibril films via direct dissolution of silk in CaCl2-formic acid solution. ACS Appl Mater Interfaces. 2015;7(5):3352–3361.
- Nazarov R, Jin HJ, Kaplan DL. Porous 3-D scaffolds from regenerated silk fibroin. Biomacromolecules. 2004;5(3):718–726.
- Kim HJ, Kim MK, Lee KH, et al. Effect of degumming methods on structural characteristics and properties of regenerated silk. Int J Biol Macromol. 2017;104(Pt A):294–302.
- Basso DM, Beattie MS, Bresnahan JC. Graded histological and locomotor outcomes after spinal cord contusion using the NYU weight-drop device versus transection. Experimental Neurology. 1996;139(2):244–256.
- Takahashi A, Nakajima H, Uchida K, et al. Comparison of mesenchymal stromal cells isolated from murine adipose tissue and bone marrow in the treatment of spinal cord injury. Cell Transplant. 2018;27(7):1126–1139.
- Zheng B, Wang C, He L, et al. Neural differentiation of mesenchymal stem cells influences chemotactic responses to HGF. J Cell Physiol. 2013;228(1):149–162.
- Zhang F, Lu Q, Ming JF, et al. Silk dissolution and regeneration at the nanofibril scale. J Mater Chem B. 2014;2(24):3879–3885.
- Rowland JW, Hawryluk GW, Kwon B, et al. Current status of acute spinal cord injury pathophysiology and emerging therapies: promise on the horizon. Neurosurg Focus. 2008;25(5):E2.
- Qu J, Wang D, Wang HH, et al. Electrospun silk fibroin nanofibers in different diameters support neurite outgrowth and promote astrocyte migration. J Biomed Mater Res A. 2013;101(9):2667–2678.
- Shen Y, Qian Y, Zhang H, et al. Guidance of olfactory ensheathing cell growth and migration on electrospun silk fibroin scaffolds. Cell Transplant. 2010;19(2):147–157.
- Reynolds LF, Bren MC, Wilson BC, et al. Transplantation of porous tubes following spinal cord transection improves hindlimb function in the rat. Spinal Cord. 2008;46(1):58–64.
- Zhang ZS, Ding ZZ, Huang JW, et al. Green process to prepare water-insoluble silk scaffolds with silk I structure. Int J Biol Macromol . 2018; 117:144–151.
- Vepari C, Kaplan DL. Silk as a biomaterial. Prog Polym Sci. 2007;32(8–9):991–1007.
- Rockwood DN, Preda RC, Yücel T, et al. Materials fabrication from Bombyx mori silk fibroin. Nat Protoc. 2011;6(10):1612–1631.
- Zhang X, Reagan MR, Kaplan DL. Electrospun silk biomaterial scaffolds for regenerative medicine. Adv Drug Deliv Rev. 2009;61(12):988–1006.
- Ueno Y, Chopp M, Zhang L, et al. Axonal outgrowth and dendritic plasticity in the cortical peri-infarct area after experimental stroke. Stroke. 2012;43(8):2221–2228.
- Ricci G, Volpi L, Pasquali L, et al. Astrocyte-neuron interactions in neurological disorders. J Biol Phys. 2009;35(4):317–336.