Abstract
Endothelial dysfunction initiates the pathogenesis of a myriad of cardiovascular diseases, yet the precise underlying mechanisms remain unclear. Current model utilises mechanical denudation of arteries resulting in an arterial-injury model with onset of intimal hyperplasia (IH). Our study shows that 5 min enzymatic denudation of human umbilical artery (hUA) lumen at 37 °C efficiently denudes hUA while maintaining vessel integrity without significantly increase intima-media thickness after 7 days in culture. This ex-vivo model will be a valuable tool in understanding the mechanism of re-endothelialization prior to smooth muscle cells (SMC) activation thus placating IH at an early stage.
Introduction
Vascular endothelial cells (EC) that lines the vascular intima, the innermost layer of blood vessels acts as a selectively permeable barrier between the blood and surrounding tissues. Additionally, the endothelium plays a central role in regulating vascular functions, which includes controlling the vessel tone, regulating blood flow, preventing thrombosis and modulating the immune response [Citation1]. Intima damage or dysfunction contributes to increased inflammation, impaired vascular permeability, leukocyte transmigration, and thrombosis [Citation2–4]. Endothelial dysfunction can have significant implications on vascular health and is considered a key early event in the development of many cardiovascular related diseases [Citation5,Citation6].
Endothelial dysfunction begins with an initial injury or damage to the endothelial cells, which lead to changes in the endothelial function. These changes include impaired vasodilation, increased adhesion of leukocytes and platelets, increased vascular permeability and reduced production of nitric oxide [Citation2]. In addition, the dysfunction triggers a phenotypic switch in SMC at the media layer leading to its proliferative state. The activated SMC start to proliferate and migrated to the intimal layer leading to IH, which results in the formation of a thickened neointima layer [Citation7,Citation8]. This condition contributes to the narrowing of the vessel lumen, leading to stenosis. The thickened intimal layer and altered vessel architecture due to IH can exacerbate endothelial dysfunction and perpetuate the cycle of vascular injury, inflammation and proliferation [Citation9]. If the condition persists, it can lead to the development of several cardiovascular pathologies such as atherosclerosis, hypertension or vessel restenosis [Citation10,Citation11].
Hence, vessel re-endothelialization plays a critical role in attenuating IH. Once the integrity and function of the endothelial layer is restored, it helps to improve barrier function, reduce inflammation, inhibit thrombosis and regulate SMC behaviour, contributing to the long-term success of vascular repair and preventing complications associated with vascular injuries [Citation12,Citation13]. Over the years, various animal models were developed to understand the mechanism and potential of re-endothelialization following endothelial dysfunction. Numerous methods to introduce endothelial injury with varying degrees of modelling difficulties had been studied [Citation14]. A common disease model for IH following endothelial dysfunction is the murine carotid artery balloon injury model where mechanical injury of EC lining was established and subsequent endothelialisation were analyzed [Citation15]. In this model, injuries to the medial layer are expected, activating SMC over-proliferation due to phenotypic switching as a response to endothelial dysfunction resulting in accelerated progression of vessel stenosis [Citation14,Citation16,Citation17]. However, this model primarily focuses on analysing changes at later time points when the vessel stenosis has progressed significantly.
Our study aims to establish a gentle endothelial-denuded ex-vivo model with slower progression of vessel stenosis. This model can be a valuable tool to better understand the early cellular and molecular responses that occur following endothelial injury and how these events contribute to the subsequent re-endothelialization process. Insights gain may help in identifying potential therapeutic targets or strategies to enhance re-endothelialization process and mitigate the development of cardiovascular diseases resulting from endothelial dysfunction.
Materials and methods
This study was approved by Universiti Kebangsaan Malaysia Research Ethics Committee (UKMREC) (ethics no. UKM PPI/111/8/JEP-2021-193). Human tissues used in this study were discarded tissues and was collected following protocols approved from UKMREC.
Sample collection and isolation of human umbilical artery
Healthy donors undergoing caesarean delivery at Hospital Canselor Tuanku Mukhriz in Kuala Lumpur, Malaysia provided approximately 10 – 15 cm of umbilical cord samples. The patients scheduled for surgery were consented prior to their operation. The umbilical arteries were isolated from the cord in a sterile environment. To maintain sample quality, the time between delivery and sample processing was controlled and did not exceed 24 h. Isolated arteries were washed with Dulbecco’s Phosphate Buffered Saline (DPBS, Gibco) and 1% antibiotic-antimycotic solution (AA, Corning) prior to denudation protocol.
Endothelial denudation of human umbilical artery via enzymatic protocol
In a sterile condition, the denudation protocol was setup as shown in . To begin, a butterfly needle was carefully inserted into one end of the artery and secured in place using an arterial clamp. The artery was then gently flushed with 2–3 ml of DPBS to eliminate any remaining blood within the arterial lumen. Subsequently, the artery was flushed with 1 ml of 1X trypLE Select enzyme (Gibco) before clamping both ends of the artery with the arterial clamp. A small volume of the enzyme was injected into the artery, completely filling the lumen, and this process was stopped just before the artery stretched and expanded. The artery filled with trypLE Select enzyme was incubated with different temperature and incubation time. The incubation time and temperature were tested for 3, 5 and 10 min at room temperature and at 37 °C. After the designated incubation period, the arterial clamp was gently removed from one end of the artery. The artery was flushed with Dulbecco’s modified eagle’s medium (DMEM, Gibco) with 10% foetal bovine serum (FBS, Capricorn). The flushed solution was collected into a centrifuge tube for further culturing of EC. A summary of the tested parameters including enzyme type, temperature and incubation time was provided in the supplemental data.
Assessment on the efficiency of enzymatic denudation protocol
The denuded arteries were fixed in 10% neutral-buffered formalin (NBF, Leica) for histological sample processing and embedding into paraffin block. Haematoxylin and eosin (H&E) staining and immunofluorescent staining of EC and SMC markers were performed to evaluate the effectiveness of the denudation protocol, as shown in supplementary data. To determine the extent of EC removal, immunofluorescence images were captured and analysed using ImageJ software. Three sections per treatment were used to calculate the % EC coverage by measuring the lumen lining with EC over total lumen lining in one image frame. The EC coverage was recorded as percent coverage (EC coverage/total lumen lining) x 100%). Full staining protocol is available in the supplementary data.
Assessment on the distribution of glycosaminoglycans (GAG) in human umbilical arterial sections
Alcian blue staining was carried out using the Alcian Blue staining kit (Alcian Blue Staining Kit, pH 2.5 (mucin stain), Abcam). Images were captured using Olympus BX53 microscope. All images were taken with the same standardised settings for consequent GAG staining intensity analysis. The intensity of alcian blue staining was measure at the near lumen area of the arterial section. For each arterial section, five regions of interest were selected and captured for analysis. ImageJ software was used to measure the staining intensity by measuring the mean gray value of the staining and the resulting data was recorded as a percentage (% value).
Characterisation of collected cells post-denudation protocols
A 12-well tissue culture plate was coated with 5 μg/mL fibronectin for 1 h prior to EC isolation. The collected DMEM with 10% FBS suspension from the denudation protocol was centrifuged at 200 g for 10 min to obtain a pellet of endothelial-like cells. The isolated endothelial-like cells were cultured in Endothelial Basal Medium MV2 (PromoCell) supplemented with Endothelial Cell Growth Medium MV2 SupplementMix (PromoCell) at 37 °C and 5% CO2 until it reaches sub-confluent. Immunocytochemistry of EC markers were performed to characterise the isolated cells at passage 3. The full staining protocol is available in the supplementary data.
Denuded hUA ex-vivo culture
After denudation, the artery was cut open and cultured in DMEM and 10% FBS. The cultured artery was maintained on a seesaw rocker (Stuart SSM4, Cole Palmer) for a duration of 7 days. Subsequently, the arterial section was fixed in 10% NBF for histological sample processing and embedding into paraffin block before sectioned at 3 μm. The tissue sections were deparaffinized and rehydrated in xylene and graded alcohol prior to H&E staining. The analysis of intima-media thickness was conducted using Olympus BX53 microscope.
Statistical analysis
The experiments were conducted using 4–5 independent biological replicates to ensure robustness. Statistical analysis was performed using GraphPad Prism 9 software. The results were presented as mean ± standard deviation (SD). Prior to conducting statistical tests, the data were assessed and confirmed for normality. For % EC coverage and GAG intensity, comparison was made between control group (native artery) and treatment groups using two-way analysis of variance (ANOVA). For analysis of intima-media thickness, comparison between control group (denuded artery, day 0) and treatment group (denuded artery, day 7) was made using paired t-test. A statistical of p < 0.05 was considered significant.
Results
The gentle endothelial-denuded model was created through enzymatic process to remove the endothelial layer from the vessel (see ). The effectiveness of this denudation protocol was assessed based on three criteria: (1) the vessel’s structural integrity, (2) successful removal of EC layer, and (3) the preservation of GAG near the lumen area. Following the denudation procedure, the vessel structures remained intact as evidenced by H&E staining (). Unlike the denuded artery sections, the intima layer was still visible in the native tissue (). To analyse the denudation efficiency, EC marker CD31 expression on the vessel lumen was examined. The native artery’s luminal surface exhibited intact CD31+ EC (). On the contrary, the denuded artery showed a significant reduction in EC coverage (). Both the native and denuded artery sections displayed αSMA+ (alpha smooth muscle actin) SMC, demarking the vessel’s medial layer ().
Figure 2. (A,B) Representative staining of CD31 and αSMA on denudation protocol using trypLE Select and trypsin-EDTA at RT and 37 °C with its Respective incubation period. (C,D) Quantification of EC coverage post denudation protocol at RT and 37 °C respectively. ** p < 0.01 and * p < 0.05 compared to native artery using two-way ANOVA. (N = 4; Scale bar: 100 μm).
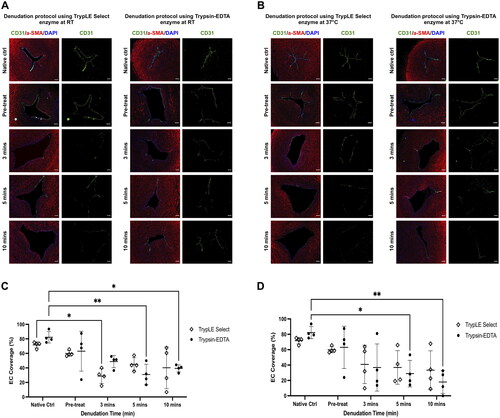
The preservation of GAG in the denuded artery was analysed through Alcian blue staining result. Tissue sections subjected to longer incubation period exhibited a noticeable reduction in GAG distribution compared to native tissue, especially at the near-lumen area (). Considering these observations, both denudation protocols of flushing the lumen with trypsin-EDTA for either 5 or 10 min at 37 °C effectively remove 71.7% ± 17.3% and 82.1% ± 14.8% of EC respectively whilst preserving GAG distribution (). The denuded artery was subsequently cultured for 7 days to investigate possible thickening of the intima-media layer. However, the result shows that no significant increase in intima-media thickness was observed in the denuded artery (). This gentle denudation protocol allows EC collection to be made for potential use in future studies. The isolated cells exhibited endothelial morphology with cobblestone-like polygonal appearance and expressed various EC markers; CD31, CD146, vWF (von Willebrand Factor) and VEGFR2 (Vascular endothelial growth factor receptor 2). Notably, these isolated cells did not express the SMC marker, αSMA ().
Figure 3. (A,B) Representative image of Alcian blue staining from denudation using trypLE Select and trypsin-EDTA enzyme at RT and 37 °C. (C,D) Intensity analysis of Alcian blue at RT and 37 °C respectively. ** p < 0.01 compared to native tissue using two-way ANOVA. (N = 4, scale bar: 200 μm).
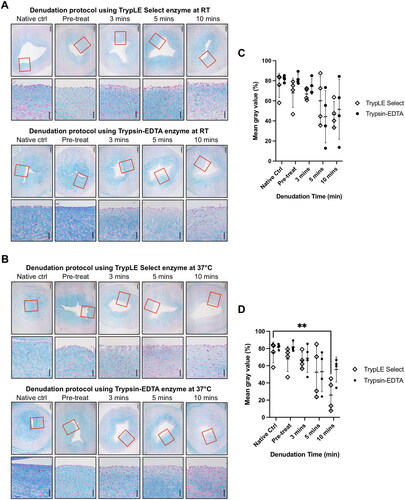
Figure 4. (A) Intima-media thickness of denuded hUA at day 0 and after cultured for 7 days (B). (C) Result shows non-significant increase of intima-media thickness at day 7 compared to day 0. L = lumen (paired t-test, N = 5, scale bar 200 μm) (D) immunophenotype of isolated cells is CD31+CD146+vWF+VEGFR2+αSMA-. (Scale bar: 100 μm).
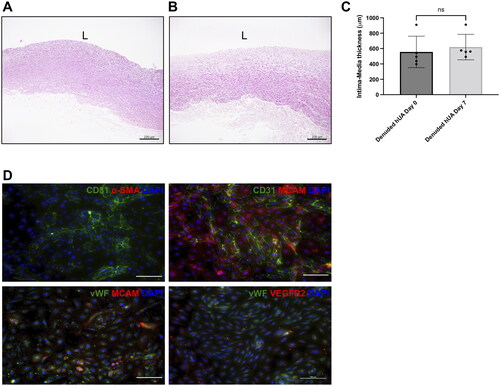
Discussion
Endothelial dysfunction is recognised as a key feature in various cardiovascular diseases [Citation6]. Currently, the most common method to induce endothelial injury is through mechanical means, such as using wires, balloon catheters or needles [Citation15]. Although this approach effectively causes endothelial injury, it comes with several drawbacks. One of the disadvantages is its non-specific nature, which does not only target the EC but also damages the underlying SMC. Consequently, this triggers inflammatory responses within minutes, activating various pro-inflammatory signalling pathways, particularly MAPK and NF-κB [Citation14]. Utilising this model to study re-endothelialization cannot address the cellular and molecular responses prior to IH as these studies usually done at late time points where IH has progressed significantly. Plus, the early inflammation may overshadow specific events involved in the re-endothelialization process, making it challenging to distinguish between the two processes.
In addition to mechanical denudation, chemical agents have been utilised to selectively remove EC layer. Chemical denudation is a laboratory technique employed to mimic certain aspects of pathophysiological conditions, specifically the loss of EC layer. Several common chemical agents used for endothelial denudation include proteolytic enzymes such as trypsin, collagenase and dispase, as well as ethylene diamine tetraacetic acid (EDTA) and detergent-based agents such as sodium deoxycholate [Citation18,Citation19]. While detergent-based agents were initially employed for endothelial denudation, they proved harmful to vessel tissue, prompting the exploration of alternative methods [Citation20]. In our study, we have chosen to use trypsin-based enzymes (trypLE Select and trypsin-EDTA) to gently denude the endothelial layer. The primary objective of our denudation protocol is to create an ex-vivo model of a denuded artery that can be later utilised to study EC-SMC interactions and mechanism involved during subsequent re-endothelialization.
In this study, various parameters were tested to optimiza a successful gentle denudation protocol that: (1) retain vessel’s integrity, (2) successful removal of EC layer, and (3) preservation of GAG near lumen area. Maintaining vessel integrity is crucial to establish a viable model for subsequent re-endothelialization studies. Previous work by Kural, et al. also demonstrated that enzymatic denudation resulted in lower percentage of SMC death and proliferation compare to mechanical denudation [Citation18]. In our study, denudation with trypsin-EDTA enzyme showed significant endothelial denudation compared to trypLE Select. This is due to the mechanism of trypsin, a proteolytic enzyme that breaks down protein anchoring EC to the underlying extracellular matrix (ECM) and EDTA, which chelates calcium ions that are crucial in maintaining cell-cell adhesion [Citation21]. The combined actions of these chemicals likely leads to a more efficient removal of EC compared to trypLE select. While collagenase is also effective in denuding the EC layer, it was not used in this study. Collagenase mechanism of action involves breaking down collagen protein in the ECM and thus inducing EC detachment and subsequently, denudation. However, this study employs trypsin over collagenase because it damages both SMC and vessel extracellular matrix, compromising the integrity and mechanical properties of the vessel [Citation22].
Chemical denudation offers several advantages over mechanical method, allowing alternatives intended in certain research scenarios. The chemical denudation technique is less invasive compared to mechanical method, which involves physical disruption of EC layer. Additionally, chemical denudation provides better reproducibility due to its ability to offer more precise control over the denudation process, leading to reduced variability in experimental outcomes. A summary of the differences between chemical and mechanical denudation can be found in .
Table 1. Comparison of chemical and mechanical endothelial denudation.
Preserving the distribution of GAG in the denuded artery model is of utmost importance because intact glycocalyx correlates with vessel’s integrity. GAG plays a vital role in regulating vascular physiological functions and pathophysiological events through its anti-thrombogenic and anti-inflammatory nature [Citation23,Citation24]. Additionally, GAG contributes to cellular recruitment by confining chemokines localisation, thereby attracting migrating cells to the site of injury [Citation25,Citation26]. The preservation or construction of the vascular glycocalyx has shown to improve the clinical outcome of tissue-engineered vascular graft [Citation23]. Hence, in this study, achieving a delicate balance between effective endothelial denudation while maintaining GAG integrity is crucial as enzyme’s proteolytic activity can partially removes GAG [Citation27]. Based on the results, the optimal denudation protocol involves 5-min incubation using trypsin-EDTA enzyme at 37 °C. These parameters demonstrate significant EC reduction while preserving GAG content, thereby meeting the critical criteria for this model.
Generally, the endothelium lining blood vessel lumens consist of EC. However, these EC exhibit distinct phenotypes based on their arterial or venous origin, which are genetically determined and related to their functional differences [Citation28]. A meta-analysis study investigating differentially expressed genes between HUAEC and human umbilical vein endothelial cells (HUVEC) has confirmed the preservation of arterial-venous identity, especially in hypoxic environment [Citation29]. Despite being later applied to an arterial system, the majority of recellularization research, particularly for cardiovascular diseases has been conducted using HUVEC. Therefore, the downstream application of HUAEC cannot be disregarded, making the isolation of HUAEC even more valuable. This protocol allows for the collection of HUAEC, which is often lost in the mechanical denudation protocol. The collected EC is characterised with EC phenotype, CD31+vWF+VEGFR2+ [Citation30].
An ex-vivo model proves valuable as it enables research to be done in a controlled environment, ensuring reproducibility within the laboratory setting. Moreover, it offers the advantage of focusing on specific cellular processes or mechanisms that may be challenging to study in-vivo due to complexity of the organism. Many studies have utilised short to moderate culture periods for ex-vivo tissues [Citation31]. Notably, in vascular tissue research, studies have demonstrated the viability of ex-vivo tissue culture for up to 14 days, facilitating real-time observations following various treatments [Citation18,Citation32]. Therefore, this endothelial-denuded artery model provides a controlled environment outside of a living organism, enabling researchers to investigate the process of EC regeneration and repair following endothelial injury. The ex-vivo model allows for a targeted study of specific cellular or molecular responses following endothelial injury, which is challenging to achieve in an in-vivo model. Moreover, as the denudation protocol specifically targets the EC layer without damaging the SMC, it becomes a valuable tool to evaluate tissue-engineered vascular grafts (TEVG).
In the development of (TEVG), endothelialization is crucial for the recellularization of decellularized scaffolds or synthetic grafts. Once the grafts are fabricated or decellularized, various recellularization approach are employed; in-vitro (static or dynamic perfusion system) or in-vivo (allowing native cells to repopulate the transplanted grafts) [Citation33,Citation34]. Despite studies showing successful recellularization, current methods often fail to replicate the natural blood vessel layer, as endothelial progenitor cells attach to the ECM of decellularized scaffolds, resulting in suboptimal outcomes [Citation35]. In addition, finding a balance to repopulate the grafts with SMC and EC proves to be challenging in-vitro or in-vivo. A study by Hsia, et al. has shown that in-vivo endothelialization of decellularized umbilical artery leads to neointimal hyperplasia as SMC were repopulated in the vessel graft prior to EC recruitment [Citation34]. The different rates of proliferation between SMC and EC may contribute to the occurrence of stenosis, which is proven to be difficult to be controlled in-vivo. This highlights the challenge of regulating the recruitment of both SMC and EC to create a fully functioning graft.
Our endothelial-denuded model offers a potential solution by providing insights into the EC-SMC interaction, thus enhancing our understanding of vascular graft endothelialization. We believe that with this reproducible protocol of developing endothelial-denuded ex-vivo umbilical artery, it is adaptable to different types of blood vessels, which is used in other research areas. With this improved understanding, we hope to advance the development of TEVG, making them more biologically relevant and capable of better mimicking the natural blood vessel structure and function.
Conclusion
In conclusion, our work has successfully optimised a gentle denudation protocol using enzymatic incubation with trypsin-EDTA for 5 min at 37 °C. This method efficiently removes EC whilst preserving vessel integrity and GAG content. The resulting endothelial-denuded ex-vivo model exhibits a slower progression of vessel stenosis, making it particularly valuable for studying re-endothelialisation immediately following endothelial dysfunction. This model offers new insights into the early stages of re-endothelialization and its potential implications for preventing or managing cardiovascular conditions associated with endothelial dysfunction.
Author’s contributions
S.S.A, M.D.Y, N.S designed research. S.S.A performed research. N.A.A.G, R.Z.A.R.S, M.R.A.R contribute reagents; provide clinical advice on sample handling and analysis. S.S.A, M.D.Y, N.S wrote the paper.
Supplemental Material
Download MS Word (128.5 KB)Disclosure statement
All authors declare no financial or non-financial competing interests.
Data availability statement
The data that support the findings of this study are available from the corresponding author, [NS], upon reasonable request.
Additional information
Funding
References
- Krüger-Genge A, Blocki A, Franke R-P, Jung F. Vascular endothelial cell biology: an update. IJMS. 2019;20(18):4411. doi: 10.3390/ijms20184411.
- Tritto I, Ambrosio G. The multi-faceted behavior of nitric oxide in vascular “inflammation”: catchy terminology or true phenomenon? Cardiovasc Res. 2004;63(1):1–4. doi: 10.1016/j.cardiores.2004.04.028.
- Zuchi C, Tritto I, Carluccio E, et al. Role of endothelial dysfunction in heart failure. Heart Fail Rev. 2020;25(1):21–30. doi: 10.1007/s10741-019-09881-3.
- Eckly A, Hechler B, Freund M, et al. Mechanisms underlying FeCL3‐induced arterial thrombosis. J Thromb Haemos. 2011;9(4):779–789. doi: 10.1111/j.1538-7836.2011.04218.x.
- Hirase T, Node K. Endothelial dysfunction as a cellular mechanism for vascular failure. Am J Physiol Heart Circulat Physiol. 2012;302(3):H499–H505. doi: 10.1152/ajpheart.00325.2011.
- Jay Widmer R, Lerman A. Endothelial dysfunction and cardiovascular disease. Glob Cardiol Sci Pract. 2014;2014(3):43. doi: 10.5339/gcsp.2014.43.
- Clowes AW, Clowes MM, Fingerle J, et al. Regulation of smooth muscle cell growth in injured artery. J Cardiovasc Pharmacol. 1989;14: s12–S15. doi: 10.1097/00005344-198900146-00005.
- Tong X, Khandelwal AR, Qin Z, et al. Role of smooth muscle nox4-based NADPH oxidase in neointimal hyperplasia. J Mol Cell Cardiol. 2015;89:185–194. doi: 10.1016/j.yjmcc.2015.11.013.
- Sun H-J, Wu Z-Y, Nie X-W, et al. Role of endothelial dysfunction in cardiovascular diseases: the link between inflammation and hydrogen sulfide. Front Pharmacol. 2020;10:10. doi: 10.3389/fphar.2019.01568.
- Mao Y, Jiang L. Effects of notch signalling pathway on the relationship between vascular endothelial dysfunction and endothelial stromal transformation in atherosclerosis. Artif Cells Nanomed Biotechnol. 2018;46(4):764–772. doi: 10.1080/21691401.2017.1337030.
- Gallo G, Volpe M, Savoia C. Endothelial dysfunction in hypertension: current concepts and clinical implications. Front Med. 2022;8:8. doi: 10.3389/fmed.2021.798958.
- Su JB. Vascular endothelial dysfunction and pharmacological treatment. WJC. 2015;7(11):719. doi: 10.4330/wjc.v7.i11.719.
- Chen J, Liu Y, Liu Y, et al. Resveratrol protects against ox-LDL-induced endothelial dysfunction in atherosclerosis via depending on circ_0091822/mir-106b-5P-mediated upregulation of TLR4. Immunopharmacol Immunotoxicol. 2022;44(6):915–924. doi: 10.1080/08923973.2022.2093740.
- Wu Y, Su S-A, Xie Y, et al. Murine models of vascular endothelial injury: techniques and pathophysiology. Thromb Res. 2018;169:64–72. doi: 10.1016/j.thromres.2018.07.014.
- Tulis DA. Rat carotid artery balloon injury model. Methods Mol Med. 2007;139:1–30. doi: 10.1007/978-1-59745-571-8_1.
- Yuan B, Liu H, Pan X, et al. LSD1 downregulates P21 expression in vascular smooth muscle cells and promotes neointima formation. Biochem Pharmacol. 2022;198:114947. doi: 10.1016/j.bcp.2022.114947.
- Ward AO, Caputo M, Angelini GD, et al. Activation and inflammation of the venous endothelium in vein graft disease. Atherosclerosis. 2017;265:266–274. doi: 10.1016/j.atherosclerosis.2017.08.023.
- Kural MH, Dai G, Niklason LE, et al. An ex vivo vessel injury model to study remodeling. Cell Transplant. 2018;27(9):1375–1389. doi: 10.1177/0963689718792201.
- Gustafsson H, Mulvany MJ, Nilsson H. Rhythmic contractions of isolated small arteries from rat: influence of the endothelium. Acta Physiologica Scandinavica. 1993;148(2):153–163. doi: 10.1111/j.1748-1716.1993.tb09545.x.
- Osol G, Cipolla M, Knutson S. A new method for mechanically denuding the endothelium of small (50–150 μm) arteries with a human hair. J Vasc Res. 1989;26(5):320–324. doi: 10.1159/000158781.
- Mutin M, George F, Lesaule G, et al. Reevaluation of trypsin-EDTA for endothelial cell detachment before flow cytometry analysis. Endothelium. 1996;4(4):289–295. doi: 10.3109/10623329609024704.
- Trabelsi O, Dumas V, Breysse E, et al. In vitro histomechanical effects of enzymatic degradation in carotid arteries during inflation tests with pulsatile loading. J Mech Behav Biomed Mater. 2020;103:103550. doi: 10.1016/j.jmbbm.2019.103550.
- Siren EMJ, Luo HD, Tam F, et al. Prevention of vascular-allograft rejection by protecting the endothelial glycocalyx with immunosuppressive polymers. Nat Biomed Eng. 2021;5(10):1202–1216. doi: 10.1038/s41551-021-00777-y.
- Kohli S, Shahzad K, Jouppila A, et al. Thrombosis and inflammation—a dynamic interplay and the role of glycosaminoglycans and activated protein C. Front Cardiovasc Med. 2022;9:866751. doi: 10.3389/fcvm.2022.866751.
- Proudfoot A, Johnson Z, Bonvin P, et al. Glycosaminoglycan interactions with chemokines add complexity to a complex system. Pharmaceuticals. 2017;10(4):70. doi: 10.3390/ph10030070.
- Proudfoot AEI, Handel TM, Johnson Z, et al. Glycosaminoglycan binding and oligomerization are essential for the in vivo activity of certain chemokines. Proc Natl Acad Sci USA. 2003;100(4):1885–1890. doi: 10.1073/pnas.0334864100.
- Lin C, Kaper HJ, Li W, et al. Role of endothelial glycocalyx in sliding friction at the catheter-blood vessel interface. Sci Rep. 2020;10(1):11855 doi: 10.1038/s41598-020-68870-x.
- Dela Paz NG, D’Amore PA. Arterial versus venous endothelial cells. Cell Tissue Res. 2009;335(1):5–16. doi: 10.1007/s00441-008-0706-5.
- Vega-Tapia F, Peñaloza E, Krause BJ. Specific arterio-venous transcriptomic and ncrna-RNA interactions in human umbilical endothelial cells: a meta-analysis. iScience. 2021;24(6):102675. doi: 10.1016/j.isci.2021.102675.
- Rakocevic J, Orlic D, Mitrovic-Ajtic O, et al. Endothelial cell markers from clinician’s perspective. Exp Mol Pathol. 2017;102(2):303–313. doi: 10.1016/j.yexmp.2017.02.005.
- Hughes DL, Hughes A, Soonawalla Z, et al. Dynamic physiological culture of ex vivo human tissue: a systematic review. Cancers. 2021;13(12):2870. doi: 10.3390/cancers13122870.
- Qu Q, Bing W, Meng X, et al. Upregulation of mir-126-3p promotes human saphenous vein endothelial cell proliferation in vitro and prevents vein graft neointimal formation ex vivo and in vivo. Oncotarget. 2017;8(63):106790–106806. doi: 10.18632/oncotarget.22365.
- Smith RJ, Nasiri B, Kann J, et al. Endothelialization of arterial vascular grafts by circulating monocytes. Nat Commun. 2020;11(1):1622. doi: 10.1038/s41467-020-15361-2.
- Hsia K, Wang T-S, Liu C-S, et al. Decellularized human umbilical artery exhibits adequate endothelialization in xenogenic transplantation. Biotechnol Bioproc E. 2023;28(3):439–450. doi: 10.1007/s12257-022-0256-9.
- Gui L, Muto A, Chan SA, et al. Development of decellularized human umbilical arteries as small-diameter vascular grafts. Tissue Eng Part A. 2009;15(9):2665–2676. doi: 10.1089/ten.tea.2008.0526.