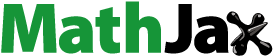
Abstract
The various therapeutic drugs that are currently utilized for the management of cancer, especially breast cancer, are greatly challenged by the augmented resistance that is either acquired or de novo by the cancer cells owing to the long treatment periods. So, this study aimed at elucidating the possible anticancer potential of four compounds 7, 4′, 7′′, 4′′′-tetra-O-methyl amentoflavone, hesperidin, ferulic acid, and chlorogenic acid that are isolated from Cycas thouarsii leaves n-butanol fraction for the first time. The MTT assay evaluated the cytotoxic action of four isolated compounds against MDA-MB-231 breast cancer cells and oral epithelial cells. Interestingly, ferulic acid revealed the lowest IC50 of 12.52 µg/mL against MDA-MB-231 cells and a high IC50 of 80.2 µg/mL against oral epithelial cells. Also, using an inverted microscope, the influence of ferulic acid was studied on the MDA-MB-231, which revealed the appearance of apoptosis characteristics like shrinkage of the cells and blebbing of the cell membrane. In addition, the flow cytometric analysis showed that the MDA-MB-231 cells stained with Annexin V/PI had a rise in the count of the cells in the early and late apoptosis stages. Moreover, gel electrophoresis detected DNA fragmentation in the ferulic acid-treated cells. Finally, the effect of the compound was tested at the molecular level by qRT-PCR. An upregulation of the pro-apoptotic genes (BAX and P53) and a downregulation of the anti-apoptotic gene (BCL-2) were observed. Consequently, our study demonstrated that these isolated compounds, especially ferulic acid, may be vital anticancer agents, particularly for breast cancer, through its induction of apoptosis through the P53-dependent pathway.
1. Introduction
Unfortunately, there is an increased incidence of cancer along with its high rates of mortality all over the world. Thus, the scientific community has greatly studied this problematic disease [Citation1]. Cancer has various therapies, including chemotherapy, immunotherapy, physical surgery, radiotherapy, and recently nanotherapy. All such methods have been commonly utilized for combating such fatal illnesses [Citation2,Citation3]. Chemotherapy, in particular, is the most frequently utilized therapeutic approach for many cancer patients [Citation4,Citation5].
The main alarm regarding cancer treatment is its numerous and severe side effects, which have detrimental impacts on the quality of life of the cancer patient. Cancer treatments harm cancer cells by producing reactive oxygen species [Citation6]. However, such cancer drugs also adversely affect normal cells [Citation7]. The side effects vary from vomiting, nausea, hair loss, and fatigue to even death in certain cases [Citation8,Citation9].
Breast cancer is the most common one in women. It has been documented that there were approximately two million new cancer cases in the previous decade. Breast cancer is about 11.6% of the total number of cancer cases [Citation10,Citation11]. The resistance of the cancer cells to the currently used cancer therapies is a great challenge. It commonly occurs due to the long treatment periods of cancer. This issue, in addition to the numerous and severe side effects of the chemotherapeutic drugs, represents a large obstacle to the treatment of cancer patients, particularly breast cancer, in clinical practice [Citation12,Citation13]. Thus, novel therapies are crucial for treating cancer patients [Citation14,Citation15]. Science and research are turning more and more attention to natural chemicals since they are believed to have less dangerous side effects than traditional therapies like chemotherapy. The plant kingdom yields naturally occurring secondary metabolites that are being evaluated for their anticancer properties, which will lead to the creation of novel therapeutic medications [Citation16]. These secondary metabolites may be flavonoids or other phenolic derivatives. Utilizing various approaches, several phenolic compounds derived from medicinal plants that are consumed have a considerable anticancer effect. According to reports, flavonoids may function as chemopreventive agents by interfering with a number of cancer pathways, including the activation of apoptosis and differentiation, the arrest of the cell cycle to reduce cell growth and proliferation, or a combination of these mechanisms [Citation17].
In this study, we elucidated the possible in vitro anticancer potential of four compounds first isolated from Cycas thouarsii R.Br. leaves n-butanol fraction as a trial to find substitutions for the chemotherapeutic drugs with various drawbacks.
2. Materials and methods
2.1. Plant
Cycas thouarsii R.Br. leaves (Figure S1) were gathered, identified, and described in accordance with Negm et al. [Citation63]. Methanol (4–5 L) was used to extract the ground leaves powder (1.75 kg). Under reduced pressure, the methanolic extract was evaporated to get a total residue that was then resuspended in methanol: water (1:1), then successfully partitioned with n-hexane, di chloro methane, ethyl acetate, and finally n-butanol saturated with water. The fraction of n-butanol (14.5 g) was utilized for phytochemical isolation of their major compounds. The fraction of n-butanol (9 g) dissolved in 10 ml methanol (thick paste). This paste was loaded on a VLC column (60 cm × 8 cm) stationary phase silica gel for VLC. Wash the column 3 times with 500 ml of n-hexane 60–80. Start a gradient elution mobile phase dichloromethane: methanol 99:1, 98:2, 97:3, till 90:10, then monitor the fraction with TLC to give 4 main fractions. The first fraction (201 mg) eluted at 99% was purified upon Sephadex LH 20 to give compound 1 (23 mg). Fraction 2 eluted from 93:7 (900 mg) was loaded on the open silica gel column with isocratic elution 95:5 mobile phase to yield three subfractions. Each subfraction was purified on Sephadex LH 20 to get high-purity compounds 2 (19 mg), 3 (31 mg), and 4 (21 mg), respectively.
2.2. Chemicals and cell lines
The utilized cell lines were purchased from Vacsera, Egypt. They included oral epithelial cells (normal cells, catalogue no. ABC-TC4365) and the human breast cancer cell (MDA-MB-231, catalogue no. 92020424). The two cell lines were conserved in Dulbecco’s Modified Eagle Medium (DEMEM) with 1% (v/v) penicillin/streptomycin as well as 10% (v/v) foetal bovine serum (FBS). The cells were incubated in 5% CO2 at 37 °C in a CO2 incubator (NUAIRE, USA). Regarding the chemicals and solvents used in the current study, they were obtained from Merck, UK.
2.3. MTT assay
The cell lines were put into 96-well plates with 104 cells/mL concentrations. After that, they were incubated in the previously mentioned conditions for 24 h to reach the exponential cellular growth. The cells were then exposed to several concentrations of the investigated compounds (6.25, 12.5, 25, 50, and 100 µg/mL) and incubated for 48 h. After that, the MTT reagent (5 mg/mL) was added to each well in the 96-well plate for four hours [Citation64–66]. The formazan crystals formed by the viable cells were identified via their dissolving by acidified isopropanol (100 µL). Finally, the obtained results were read at 630 nm by ELISA reader (Bio-RAD, Japan) [Citation67,Citation68].
2.4. Cell morphology
The impact of treatment with the tested drugs on the MDA-MB-231cell morphology was determined phenotypically after adding the tested compound (IC50) for 48 h. This was revealed using an inverted microscope (Leica, Germany) [Citation69].
2.5. Flow cytometric investigation
Flow cytometry was utilized to reveal the ability of the tested compounds to induce apoptosis and/or necrosis. This was performed after treatment with tested compounds (IC50) for 48 h using a Novocyte Flow Cytometer (Acea Biosciences, USA). In brief, the MDA-MB-231 cells were harvested, and then they were thoroughly washed with sterile water and suspended in phosphate-buffered saline (PBS). After that, the cells were stained with a solution of Annexin V-FITC/propidium iodide (PI) (10 μL). Flow cytometry analysis was accomplished after incubating the drug cell mixture in a dark room for 20 min [Citation66,Citation70–72].
2.6. DNA fragmentation
After extraction of DNA from the treated and untreated MDA-MB-231 cells by DNA extraction kit (Qiagen, USA), the quality of the extracted DNA was assessed by nanodrop spectrophotometer (Thermo Scientific, USA). After that, the extracted DNA was run on agarose gel after adding 1X loading dye. Finally, the DNA fragmentation was revealed by a UV transilluminator, and photos were captured by Gel Doc (Bio-rad, USA) [Citation54,Citation73].
2.7. qRT-PCR
The total RNA was extracted by PureLink™ RNA Kit (Invitrogen, USA), and its purity was evaluated by a nanodrop spectrophotometer (Thermo Scientific, USA). The tested genes were BAX, P53, and BCL-2. The B-actin gene was utilized as a housekeeping gene. The cDNA was then synthesized by SuperScript™ III First-Strand kit (Thermo Fischer, USA) using Rotor-Gene Q (Qiagen, USA). The utilized primer sequences are revealed in Table S1 [Citation74].
2.8. Statistics
The accomplished tests were performed thrice and expressed as mean ± standard deviation (SD). Here, we utilized ANOVA test to illuminate the alteration among the treated and untreated MDA-MB-231cells and it was regarded to be significant if p < 0.05. GraphPad Prism (version number 8) was employed for the statistical analysis.
3. Results
3.1. Phytochemical investigation
3.1.1. Spectroscopic data
Compound (1): C34H26O10, Pale-yellow amorphous powder.1H-NMR data (500 MHz, DMSO-d6, δ, ppm): 8.19 (1H, brs, H-6′), 8.05 (1H, brs, H-2′), 7.56 (2H, brs, H-2′′′,6′′′), 7.33 (1H, brs, H-5′), 7.04 (1H, s, H-3′′), 6.96 (1H, s, H-6′′), 6.90 (2H, brs, H-3′′′,5′′′), 6.73 (1H, brs, H-8), 6.63 (1H, s, H-3), 6.31 (1H, brs, H-6), 3.78 (3H, s, 4′′′-OMe), 3.76 (3H, s, 7-OMe), 3.75 (3H, s, 4′-OMe), 3.70 (3H, s, 7′′-OMe). APT-NMR spectrum (125 MHz, DMSO-d6, δ, ppm): 182.8 (C-4′′), 182.4 (C-4), 165.6 (C-7), 164.0 (C-2′′), 163.9 (C-2), 163.1 (C-4′′′), 162.8 (C-5), 162.0 (C-7′′), 161.6 (C-4′), 160.9 (C-5′′), 157.8 (C-9), 153.9 (C-9′′), 131.2 (C-6′), 128.4 (C-2′), 127.3 (C-2′′′,6′′′), 123.2 (C-1′′′), 123.0 (C-1′), 121.7 (C-3′), 115.0 (C-3′′′,5′′′), 112.3 (C-5′), 105.2 (C-10), 105.1 (C-10′′), 104.6 (C-8′′), 104.4 (C-3), 103.7 (C-3′′), 98.6 (C-6), 96.1 (C-6′′), 93.3 (C-8), 57.0 (4′′′-OMe), 56.6 (7-OMe), 56.5 (4′-OMe), 56.0 (7′′-OMe).
Compound (2): C28H34O15, White amorphous powder,1H-NMR (500 MHz, DMSO-d6, δ, ppm): 6.85–6.92 (3H, overlapped, H-2′, 5′, 6′), 6.10 (1H, d, J = 1.5 Hz, H-8), 6.08 (1H, s, H-6), 5.46 (1H, dd, J = 3, 12 Hz, H-2), 4.93 (1H, d, J = 7.5 Hz, H-1′′), 4.47 (1H, s, H-1′′′), 3.73 (3H, s, OMe), 3.15-3.76 (10H, m, sugar protons), 3.17 (1H, m, H-3ax), 2.71 (1H, dd, J = 3, 17 Hz, H-3eq), 1.04 (3H, d, J = 6 Hz, H-6′′′). APT-NMR (125 MHz, DMSO-d6, δ, ppm): 197.5 (C-4), 165.6 (C-7), 163.5 (C-5), 163.0 (C-9), 148.4 (C-4′), 146.9 (C-3′), 131.3 (C-1′), 118.4 (C-6′), 114.6 (C-2′), 112.5 (C-5′), 103.8 (C-10), 101.1 (C-1′′′), 99.8 (C-1′′), 96.8 (C-6), 96.0 (C-8), 78.9 (C-2), 76.7 (C-5′′), 76.0 (C-3′′), 73.4 (C-5′′′), 72.5 (C-4′′′), 71.2 (C-2′′), 70.7 (C-3′′′), 70.0 (C-2′′′), 68.8 (C-4′′), 66.5 (C-6′′), 56.1 (OMe), 42.5 (C-3), 18.3 (C-6′′′).
Compound (3): C10H10O4, Yellowish white needle-shaped crystals, mp 170 °C. 1H-NMR spectrum (500 MHz, DMSO-d6, δ, ppm): 7.43 (1H, d, J = 15 Hz, H-1′), 7.23 (1H, brs, H-3), 7.03 (1H, brd, J = 8.5 Hz, H-5), 6.74 (1H, d, J = 8.5 Hz, H-6), 6.32 (1H, d, J = 15 Hz, H-2′), 3.76 (3H, s, OMe). APT-NMR spectrum (125 MHz, DMSO-d6, δ, ppm): 168.5 (COOH), 149.5 (C-1), 148.4 (C-6), 144.9 (C-1′), 126.2 (C-4), 123.3 (C-3), 116.2 (C-2′), 115.9 (C-2), 111.5 (C-5), 56.1 (OMe).
Compound (4): C16H18O9, White amorphous powder, 1H-NMR spectrum (500 MHz, DMSO-d6, δ, ppm): 7.37 (1H, d, J = 16 Hz, H-7′), 6.99 (1H, d, J = 1.5 Hz, H-2′), 6.94 (1H, dd, J = 1.5, 8.5 Hz, H-6′), 6.72 (1H, d, J = 8.5 Hz, H-5′), 6.11 (1H, d, J = 16 Hz, H-8′), 5.02 (1H, dd, J = 7, 11 Hz, H-5), 4.89 (1H, brs, H-3), 3.87 (1H, dd, J = 3, 6.5 Hz, H-4), 1.94-1.98 (2H, m, H-6), 1.89 (1H, m, H-2ax), 1.72 (1H, m, H-2eq). APT-NMRspectrum (125 MHz, DMSO-d6, δ, ppm): 175.5 (C-7), 166.2 (C-9′), 148.8 (C-4′), 146.0 (C-3′), 145.4 (C-7′), 126.0 (C-1′), 121.9 (C-6′), 116.2 (C-5′), 115.2 (C-8′), 114.7 (C-2′), 74.0 (C-1), 71.4 (C-4), 71.0 (C-5), 70.9 (C-3), 37.7 (C-6), 37.6 (C-2).
3.1.2. Structure elucidation of the isolated compounds
Cycas thouarsii n-butanol fraction residue was subjected to column chromatography to yield four compounds for the first time identified as 4′, 7′′, 4′′′-tetra-O-methylamentoflavone, hesperidin, ferulic acid and chlorogenic acid. Their chemical structures were identified by NMR spectroscopic analysis using a JEOL ECA500 II NMR spectrometer (JEOL, Japan) and compared with the reported data. Their chemical structures are displayed in .
Figure 2. The values of IC50 of the four compounds against oral epithelial cells after treatment for 48 h: A) 7, 4′, 7′′, 4′′′-tetra-O-methylamentoflavone, B) hesperidin, C) ferulic acid, and D) chlorogenic acid.
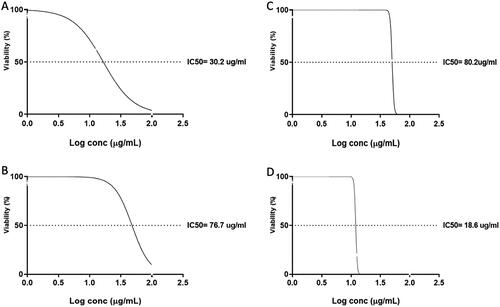
Compound (1): The para-substituted ring B of unit II was shown to have an AA′BB′ coupling system in compound 1’s 1H-NMR spectra at δH 6.90 (2H, brs, H-3′′′, 5′′′) and 7.56 (2H, brs, H-2′′′, 6′′′). The 1H-NMR spectra also revealed an ABX coupling system at δH 8.19 (1H, brs, H-6′), 8.05 (1H, brs, H-2′), and 7.33 (1H, brs, H-5′) of ring B of unit I, indicating that C-3′ was the location of the link of the two flavonoid units.
The meta-coupled protons of unit I’s ring A showed signals at δH 6.73 (1H, brs, H-8) and 6.31 (1H, brs, H-6). The APT-NMR spectrum showed two carbonyl signals at δAPT 182.8 and 182.4), suggesting that compound 1 has a biflavonoid structure. The downfield shift for C-3′ and C-8′′ signals at δAPT 121.7 and 104.6, respectively, compared to apigenin 13C-NMR spectral data, as well as the 1H-NMR signal at δH 6.96 (1H, s, H-6′′) indicated that there is no meta coupling between H-6′′ and H-8′′, confirmed the interflavonoid linkage between C-3′ and C-8′′ [Citation18]. Therefore, compound 1 was proposed to have a 3′, 8′′ bi-apigenin structure. The 1H-NMR spectrums also showed four signals corresponding to methoxy groups at δH 3.78, 3.76, 3.75, and 3.70 and their corresponding APT signals at δAPT 57.0, 56.6, 56.5, and 56.0, which suggested the presence of four methoxy substituents. The locations of these methoxy groups were confirmed at C-4′′′, C-7, C-4′ and C-7′′, respectively. The upfield shift of Δ 4.1 ppm at C-5′ (δAPT 112.3) and the downfield shift of Δ 2.7 ppm at C-1′ (δAPT 123.0) confirmed the methoxy group at C-4′ in addition, the upfield shift of Δ 1 ppm at C-3′′′, C-5′′′ (δAPT 115.0) and the downfield shift of Δ 1.8 ppm at C-1′′′ (δAPT 123.2) confirmed the methoxy group at C-4′′′. The location of methoxy groups at C-7 and C-7′′ was confirmed due to the upfield shift of Δ 0.9, 3 ppm at C-8 (δAPT 93.3) and C-6′′ (δAPT 96.1), respectively. Compound 1 was identified as 7, 4′, 7′′, 4′′′-tetra-O-methylamentoflavone by comparing the NMR spectral data to those described in the literature [Citation19–21].
Compound (2): The 1H-NMR spectrum of compound 2 showed an overlapped peak signal at δH 6.85–6.92 corresponding to aromatic protons B ring, indicating an ABX coupling system. The signals for the meta-coupled protons of ring A appeared at δH 6.10 (1H, d, J = 1.5 Hz, H-8), 6.08 (1H, s, H-6). Characteristic resonances at δH 5.46 (1H, dd, J = 3, 12 Hz, H-2), 3.17 (1H, m, H-3ax), and 2.71 (1H, dd, J = 3, 17 Hz, H-3eq) were observed due to diastreotopic effect of ring C, which suggested a flavanone moiety. APT-NMR signals confirmed the flavanone structure of compound 2 at δAPT 197.5, 78.9, and 42.5, corresponding to C-4, C-2 and C-3, respectively [Citation18]. Another characteristic signal at δH 3.73 and δAPT 56.1 suggested the presence of a methoxy group. The upfield shift of Δ 2.7 ppm at C-5′ (δAPT 112.3) and the downfield shift of Δ 2.4 ppm at C-1′ (δAPT 131.3) confirmed the methoxy group at C-4′ [Citation18]. Signals at δH 4.93 (1H, d, J = 7.5 Hz, H-1′′), 4.47 (1H, s, H-1′′′) and their corresponding carbon signals at δAPT 99.8 and 101.1 were assigned for anomeric protons of two sugar moieties. The characteristic doublet signal at δH 1.04 (3H, d, J = 6 Hz) was assigned to the characteristic methyl group of rhamnose sugar. The other carbon signal of the two sugars moieties appeared at δAPT 71.2, 76.0, 68.8, 76.7, 66.5, 70.0, 70.7, 72.5, 73.4, and 18.3 corresponding to C-2′′, C-3′′, C-4′′, C-5′′, C-6′′, C-2′′′, C-3′′′, C-4′′′, C-5′′′ and C-6′′′, respectively which were identical to glucose and rhamnose moieties [Citation22]. The location of sugar moiety was confirmed at C-7 due to the upfield shift of Δ 1.1 ppm (δAPT 165.6) compared to naringenin aglycone [Citation18]. In addition, the downfield shift of Δ 5.7 ppm at C-6′′ (δAPT 66.5) compared to the ideal glucose carbon signals indicated that C-6′′ of glucose is linked to C-1′′′ of rhamnose [Citation22]. The configuration of glucose moiety is β due to its anomeric proton’s high coupling constant value (J = 7.5). All these data were identical to those of hesperidin [Citation23,Citation24].
Compound (3): An ABX coupling system with three aromatic signals was observed in the compound 3 1H-NMR spectrum at δH 7.23 (1H, brs, H-3), 7.03 (1H, brd, J = 8.5 Hz, H-5), and 6.74 (1H, d, J = 8.5 Hz, H-6).
These signals indicated that compound 3 possesses an aromatic ring of 1, 2, and 4 trisubstituted. The existence of one methoxy group was revealed by a signal at δH 3.76 (3H, s, OMe). The 1H-NMR spectrum additionally displayed trans-ene double-bond signals at δH 7.43 (1H, d, J = 15 Hz, H-1′) and 6.32 (1H, d, J = 15 Hz, H-2′) accompanied by a high coupling constant (J = 15), demonstrating the two protons’ trans-position around the side chain’s double bond. All these signals suggested that compound 3 is a hydroxycinnamic acid methoxy derivative. The APT-NMR spectrum showed 10 signals, including 6 aromatic carbons and four aliphatic carbons comparable to ferulic acid [Citation25–28].
Compound (4): Three aromatic signals were observed in the 1H-NMR spectra of compound 4 at δH 6.99 (1H, d, J = 1.5 Hz, H-2′), 6.94 (1H, dd, J = 1.5, 8.5 Hz, H-6′), 6.72 (1H, d, J = 8.5 Hz, H-5′) indicating an ABX coupling system of the aromatic ring in addition, two doublets signals at δH 7.37 (1H, d, J = 16 Hz, H-7′) and 6.11 (1H, d, J = 16 Hz, H-8′), the high coupling constant of these protons signals indicated that the presence of two olefinic protons that is in conjugation with an aromatic ring similar to compound 4. The 1H-NMR spectrum showed multiplet signals at δH 5.02 (H-5), 3.87 (H-3), 1.94-1.98 (H-6), 1.72-1.89 (H-2), and a broad doublet signal at δH 3.51 (J = 4.5 Hz, H-4) which indicated the presence of aliphatic protons that are identical to those of quinic acid. The APT-NMR spectrum showed 16 carbon signals, including two carbonyl signals at δAPT 175.5 and 166.2, in addition to the carbon signals of the quinic acid moiety. All these signals suggested that compound 4 is a caffeoyl-substituted quinic acid derivative. It is possible to identify the location of the caffeoyl moiety by analysing the chemical shift and coupling constants of the oxygenated methine protons of the quinic acid moiety. When caffeoyl acetylated quinic acid’s oxygenated methine, its proton signal migrated down fielded. Generally, the H-3 signal appeared as a broad doublet or broad singlet peak, the H-4 signal appeared as a doublet of doublet peak with coupling constants at 6.0–8.0 and 2.0–3.0 Hz, while the H-5 signal appeared as a broad doublet of doublet peak with high coupling constants at 6.0–8.0 and 9.0–11.0 Hz. Therefore, caffeoyl moiety was confirmed at position 5 of quinic acid moiety due to the down fielded signal of H-5 at δH 5.02 with high coupling constant; however, H-3, H-4 signals appeared as broad singlet and doublet with lower coupling constant at δH 4.89 and 3.87, respectively [Citation29]. These NMR spectral data agreed with those published in the literature for chlorogenic acid [Citation29–33].
3.2. Biological investigation
3.2.1. Cytotoxic impacts on the oral epithelial cells as well as MDA-MB-231
The IC50 was determined for the tested compounds using MTT assay, as revealed in and . Compounds 7, 4′, 7′′, 4′′′-tetra-O-methylamentoflavone, hesperidin, ferulic acid and chlorogenic acid had IC50 of 30.2, 76.7, 80.2 and 18.6 µg/mL after 48 h, respectively, on the oral epithelial cells. Also, the compounds 7, 4′, 7′′, 4′′′-tetra-O-methylamentoflavone, hesperidin, ferulic acid and chlorogenic acid had IC50 of 40.32, 77.8, 12.52 and 57.55 µg/mL after 48 h, respectively, on the MDA-MB-231 cells. So, following the obtained results, ferulic acid revealed the highest cytotoxic effect among the studied compounds at the lowest concentration (IC50 of 12.52 µg/mL). Consequently, the potential mechanism of its cytotoxic activity was further elucidated at the molecular level.
3.2.2. Influence of ferulic acid on the cell morphology
The morphological alterations of the MDA-MB-231 after treatment with ferulic acid are shown in in comparison with the cells without treatment. Remarkably, certain changes were observed in the ferulic acid-treated cells, which included membrane blebbing, cell shrinkage, and apoptotic bodies formation, which are characteristics of apoptosis.
3.2.3. Flow cytometry
Flow cytometry was performed to assess the impact of ferulic acid on the apoptosis process of MDA-MB-231 cells. This was attained after staining the treated and untreated cells with Annexin V-FITC and PI stains. The flow cytometric results are shown in , , and . Interestingly, there was a substantial escalation in the count of the MDA-MB-231 cells in both the early and late apoptosis after treatment with ferulic acid when compared with the cells without treatment.
Figure 5. A and C show flow cytometric dot plots. B and D show flow cytometric histograms of the untreated MDA-MB-231 cells.
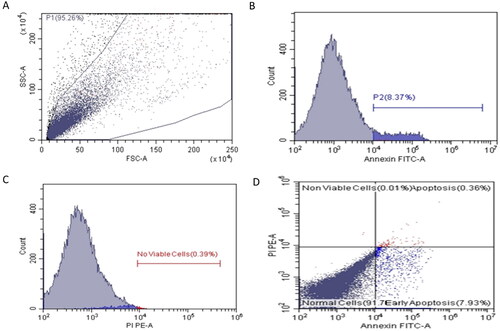
Figure 6. A and C show flow cytometric dot plots. B and D show flow cytometric histograms of compound ferulic acid-treated MDA-MB-231 cells.
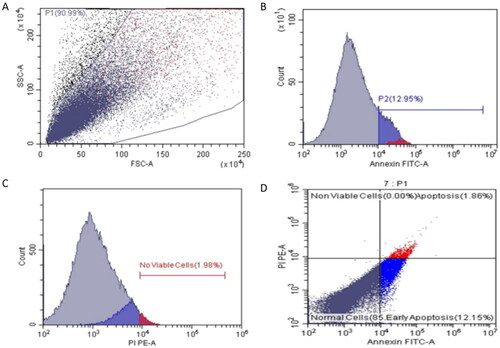
Figure 7. A bar chart showing the percentages of the early and late apoptotic cells in the untreated and treated cells.
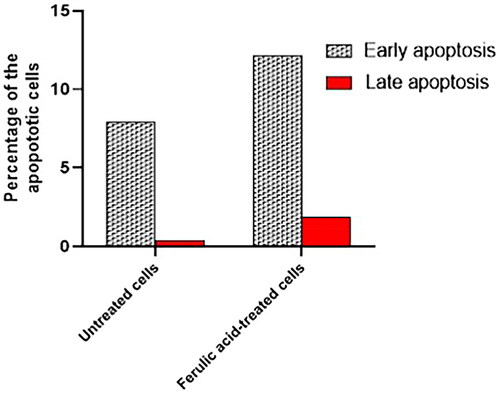
3.2.3.1. Influence on DNA fragmentation
Gel electrophoresis elucidated that ferulic acid’s impact on DNA fragmentation of the MDA-MB-231cells. As revealed in , it was observed that treatment with ferulic acid had resulted in internucleosomal DNA fragmentation.
3.2.4. Influence on apoptosis gene expression
The consequence of treating the MDA-MB-231 cells with ferulic acid was elucidated on the relative expression of the apoptosis genes, including BAX, P53, and BCL-2, by qRT-PCR . The ferulic acid has caused an increase in the expression of the BAX and P53 genes, while there was a downregulation in the expression of the BCL-2 gene.
4. Discussion
Recently, special attention has been drawn by the scientific community towards finding new compounds that possess selective cytotoxicity against cancer cells [Citation34]. Such new compounds could be better if they can hinder or even block the initiation of the carcinogenesis process and reverse its promotion either by activating the arrest of growth or via apoptosis of the cancer cells without any detrimental influences on the body’s normal cells [Citation35–36].
Many research studies have documented that various types of cancers are always activated via the dysfunction of different genes of the anti-apoptotic proteins and tumour suppressors. Therefore, these genes are the main targets for various cancer therapies [Citation37–39].
Different plants belonging to gymnosperms presented several biological activities, especially anticancer effects [Citation40]. Cycads are a very large category of plants containing several biologically active compounds such as flavonoids, biflavonoids and their methylated and glycosylated derivatives in addition to other phenolic compounds [Citation41,Citation42].
Here, we have studied the in vitro anticancer potential of the four isolated natural compounds against MDA-MB-231 cells using an MTT assay as well as an inverted microscope. Moreover, our study has determined the potential of ferulic acid to encourage the apoptosis process by staining with Annexin V/PI and flow cytometric analysis. Also, the possible capability to induce DNA fragmentation in MDA-MB-231 cells was studied after DNA extraction and gel electrophoresis. Finally, to reveal the potential effect at the genetic level, qRT-PCR was utilized to assess the potential effect on the relative expression of the apoptosis-related genes (BAX, P53, and BCL-2).
Remarkably, the results obtained by the MTT assay revealed that ferulic acid had the lowest IC50 on the MDA-MB-231 cancer cells, with IC50 of 12.52 µg/mL, and a higher IC50 on the oral epithelial cells with IC50 of 80.2 µg/mL. Thus, this compound was further studied to assess the potential mechanism of its anticancer potential on the MDA-MB-231 breast cancer cells. Using inverted microscope, ferulic acid was found to induce the different characteristics of apoptosis that, including shrinkage in the cells, formation of apoptotic bodies, and blebbing of the cell membrane [Citation43,Citation44].
The process of apoptosis is necessary for different tissues and organs to differentiate during the developmental stages. During this process, the damaged cells are lysed and removed [Citation45–47]. Such a process is controlled by different biochemical events as well as different cellular pathways [Citation48]. Throughout the apoptosis process, a sequence of alterations takes place in the cells, such as blebbing of the cellular membrane, shrinkage of the cell, DNA fragmentation, condensation of chromatin, and fragmentation of the nucleus. All such proceedings terminate with the death of the cell [Citation49,Citation50].
It was documented that the apoptotic cells exhibit many events during the early and late phases of the apoptosis process, such as their loss of phospholipid asymmetry, often resulting in exposure to the cell membrane [Citation51]. Annexin V is a stain usually used in the flow cytometric analysis of the apoptotic cells owing to its capacity to bind to the phosphatidylserine. Thus, staining of the cancer cells using Annexin V and PI could lead to differentiation between the early and late apoptotic cells. This is because the early apoptotic cells are usually stained with Annexin V and not stained with PI. Meanwhile, the late apoptotic cells are stained with both Annexin V and PI [Citation52,Citation53]. Interestingly, ferulic acid has persuaded the early and late phases of apoptosis in the ferulic acid-treated MDA-MB-231 breast cancer cells.
Chromosomal DNA fragmentation is an important feature of the apoptotic process [Citation54,Citation55]. So, in the current study, DNA was extracted from the ferulic acid-treated and untreated MDA-MB-231 breast cancer cells and visualized using gel electrophoresis. Interestingly, the extracted DNA from the treated cells was noticed to be fragmented, which confirms the induction of apoptosis by such a compound.
If P53 gene is activated, arresting the cell cycle and/or apoptosis are induced according to the level of P53 protein. It is documented that the P53 protein is a regulator that incites a response at the cellular level to different stress signals [Citation56,Citation57] as it has a tumour suppressor role. This occurs by inducing the arrest of growth as well as apoptosis, in addition to its ability to impair the angiogenesis process. Therefore, if P53 protein exists at low levels, it will potentiate the cell-cycle arrest. On the other hand, if its levels are high, induction of apoptosis would occur [Citation58,Citation59].
The apoptotic process is organized by cytochrome C that is controlled by some proteins that could be either pro-apoptotic, such as Bcl-2-associated X protein (BAX), or anti-apoptotic, like B-cell lymphoma 2 (BCL2) [Citation60–62]. Here, qRT-PCR was utilised to investigate the impact of ferulic acid on the gene expression levels of the pro and anti-apoptotic proteins. Remarkably, ferulic acid was noticed to increase the expression level of BAX and P53 genes that encode pro-apoptotic proteins. On the other hand, it declined the level of expression of BCL-2 gene, which encodes for anti-apoptotic protein. From the previous findings of the current study, we noticed that ferulic acid-induced apoptosis is through the P53-dependent pathway.
5. Conclusions
Four compounds 7, 4’, 7”, 4’’’-tetra-O-methylamentoflavone, hesperidin, ferulic acid and chlorogenic acid were successively isolated for first time from Cycas thouarsii n-butanol fraction. Ferulic acid was revealed from the current investigation to persuade cancer cell death through inducing apoptosis. This was deduced from the microscopic alterations of the treated cells that acquired the features of the apoptotic cells. In addition, the observed DNA fragmentation by gel electrophoresis in the ferulic acid-treated cells compared to the untreated cells could confirm the induction of apoptosis by ferulic acid. The flow cytometric analysis detected that ferulic acid has resulted in a rise in the count of cells in the early and late apoptosis stages. Finally, qRT-PCR was utilized to assess the anticancer activity of ferulic acid at the molecular level. It was established that the compound has induced the apoptosis process via a P53-dependant pathway. This is owing to its upregulation effect on the P53 and BAX genes as well as it’s downregulation influence on the BCL-2 gene. Therefore, more studies are essential to disclose the influence of ferulic acid on other types of cancers at the molecular level and to perform an in vivo study in animal models to assess its clinical benefit.
Authors’ contributions
Conceptualization, Engy Elekhnawy and Walaa A. Negm; Formal analysis, Engy Elekhnawy, Fatma A. Mokhtar and Hosam M. El-Seadawy; Investigation, Badriyah Alotaibi, Engy Elekhnawy, Fatma A. Mokhtar, Hosam M. El-Seadawy and Walaa A. Negm; Methodology, Fatma A. Mokhtar, Hosam M. El-Seadawy and Walaa A. Negm; Project administration, Thanaa A. El-Masry; Resources, Badriyah Alotaibi; Supervision, Thanaa A. El-Masry; Validation, Thanaa A. El-Masry; Writing – original draft, Badriyah Alotaibi, Engy Elekhnawy, Hosam M. El-Seadawy and Walaa A. Negm; Writing – review & editing, Engy Elekhnawy, Fatma A. Mokhtar and Walaa A. Negm. All authors have read and agreed to the published version of the manuscript.
Supplemental Material
Download MS Word (985 KB)Acknowledgments
Princess Nourah bint Abdulrahman University Researchers Supporting Project number (PNURSP2024R73), Princess Nourah bint Abdulrahman University, Riyadh, Saudi Arabia.
Disclosure statement
No potential conflict of interest was reported by the author(s).
Data availability statement
The data are available from the corresponding author upon reasonable request.
Correction Statement
This article was originally published with errors, which have now been corrected in the online version. Please see Correction (http://dx.doi.org/10.1080/21691401.2024.2321017)
References
- Sung H, Ferlay J, Siegel RL, et al. Global cancer statistics 2020: GLOBOCAN estimates of incidence and mortality worldwide for 36 cancers in 185 countries. CA Cancer J Clin. 2021;71(3):209–249. doi: 10.3322/caac.21660.
- Chen F, Zhuang X, Lin L, et al. New horizons in tumor microenvironment biology: challenges and opportunities. BMC Med. 2015;13(1):45. doi: 10.1186/s12916-015-0278-7.
- Ediriwickrema A, Saltzman WM. Nanotherapy for cancer: targeting and multifunctionality in the future of cancer therapies. ACS Biomater Sci Eng. 2015;1(2):64–78. doi: 10.1021/ab500084g.
- Debela DT, Muzazu SG, Heraro KD, et al. New approaches and procedures for cancer treatment: current perspectives. SAGE Open Med. 2021;9:20503121211034366. doi: 10.1177/20503121211034366.
- Falzone L, Salomone S, Libra M. Evolution of cancer pharmacological treatments at the turn of the third millennium. Front Pharmacol. 2018;9:1300. doi: 10.3389/fphar.2018.01300.
- Anand U, Dey A, Chandel AKS, et al. Cancer chemotherapy and beyond: current status, drug candidates, associated risks and progress in targeted therapeutics. Genes Dis. 2022;10(4):1367–1401. doi: 10.1016/j.gendis.2022.02.007.
- Navya P, Kaphle A, Srinivas S, et al. Current trends and challenges in cancer management and therapy using designer nanomaterials. Nano Converg. 2019;6(1):23. doi: 10.1186/s40580-019-0193-2.
- Aslam MS, Naveed S, Ahmed A, et al. Side effects of chemotherapy in cancer patients and evaluation of patients opinion about starvation based differential chemotherapy. JCT. 2014, 2014;05(08):817–822. doi: 10.4236/jct.2014.58089.
- Schirrmacher V. From chemotherapy to biological therapy: a review of novel concepts to reduce the side effects of systemic cancer treatment. Int J Oncol. 2019;54(2):407–419. doi: 10.3892/ijo.2018.4661.
- Łukasiewicz S, Czeczelewski M, Forma A, et al. Breast cancer—epidemiology, risk factors, classification, prognostic markers, and current treatment strategies—an updated review. Cancers. 2021;13(17):4287. doi: 10.3390/cancers13174287.
- Arnold M, Morgan E, Rumgay H, et al. Current and future burden of breast cancer: Global statistics for 2020 and 2040. Breast. 2022;66:15–23. doi: 10.1016/j.breast.2022.08.010.
- Nedeljković M, Damjanović A. Mechanisms of chemotherapy resistance in triple-negative breast cancer—how we can rise to the challenge. Cells. 2019;8(9):957. doi: 10.3390/cells8090957.
- Emran TB, Shahriar A, Mahmud AR, et al. Multidrug resistance in cancer: understanding molecular mechanisms, immunoprevention and therapeutic approaches. Front Oncol. 2022;12:891652. doi: 10.3389/fonc.2022.891652.
- Muss HB, Thor AD, Berry DA, et al. c-erbB-2 expression and response to adjuvant therapy in women with node-positive early breast cancer. N Engl J Med. 1994;330(18):1260–1266. doi: 10.1056/NEJM199405053301802.
- Zhong L, Li Y, Xiong L, et al. Small molecules in targeted cancer therapy: advances, challenges, and future perspectives. Signal Transduct Target Ther. 2021;6(1):201. doi: 10.1038/s41392-021-00572-w.
- Elshafie HS, Camele I, Mohamed AA. A comprehensive review on the biological, agricultural and pharmaceutical properties of secondary metabolites based-plant origin. Int J Mol Sci. 2023;24(4):3266. doi: 10.3390/ijms24043266.
- Raffa D, Maggio B, Raimondi MV, et al. Recent discoveries of anticancer flavonoids. Eur J Med Chem. 2017;142:213–228. doi: 10.1016/j.ejmech.2017.07.034.
- Markham KR, Sheppard C, Geiger H. 13C NMR studies of some naturally occurring amentoflavone and hinokiflavone biflavonoids. Phytochemistry. 1987;26(12):3335–3337. doi: 10.1016/S0031-9422(00)82499-1.
- Abdelaziz A, Sonbol F, Elbanna T, et al. Exposure to sublethal concentrations of benzalkonium chloride induces antimicrobial resistance and cellular changes in Klebsiellae pneumoniae clinical isolates. Microb Drug Resist. 2019;25(5):631–638.
- Yang F, Xu K-P, Shen J, et al. Anthraquinones and biflavonoids from selaginella delicatula. Chem Nat Compd. 2011;47(4):627–629. doi: 10.1007/s10600-011-0013-3.
- Li S, Zhao M, Li Y, et al. Preparative isolation of six anti-tumour biflavonoids from selaginella doederleinii hieron by high-speed counter-current chromatography. Phytochem Anal. 2014;25(2):127–133. doi: 10.1002/pca.2478.
- Mata R. Flavonoids, chemistry, biochemistry and applications by ØM Andersen (University of Bergen) and KR Markham (Industrial Research Ltd.). Boca Raton: CRC Press/Taylor & Francis. 2006. xiv+ 1237 pp. 7 × 101/4 in. $249.95. ISBN 0-8493-2021-6. 2007.
- El-Sayed MA, Al-Gendy AA, Hamdan DI, et al. Phytoconstituents, LC-ESI-MS profile, antioxidant and antimicrobial activities of citrus x limo. N L. Burm. f. Cultivar Variegated Pink Lemon. J Pharm Sci Res. 2017;9:375.
- El Kawy A, Michel MA, Kirollos CG, et al. M. In vitro MAO-B inhibitory effects of citrus trifoliata L. fruits extract, self-nano-emulsifying drug delivery system and isolated hesperidin: enzyme assay and molecular docking study. Egypt J Chem. 2020;63:897–906.
- López-Martínez LM, Santacruz-Ortega H, Navarro R-E, et al. A 1H NMR investigation of the interaction between phenolic acids found in mango (Manguifera indica cv Ataulfo) and papaya (Carica papaya cv Maradol) and 1, 1-diphenyl-2-picrylhydrazyl (DPPH) free radicals. PLOS One. 2015;10(11):e0140242. doi: 10.1371/journal.pone.0140242.
- Sajjadi SE, Shokoohinia Y, Moayedi N-S. Isolation and identification of ferulic acid from aerial parts of Kelussia odoratissima Mozaff. Jundishapur J Nat Pharm Prod. 2012;7(4):159–162.
- Kumar N, Pruthi V. Structural elucidation and molecular docking of ferulic acid from parthenium hysterophorus possessing COX-2 inhibition activity. 3 Biotech. 2015;5(4):541–551. doi: 10.1007/s13205-014-0253-6.
- Liu W, Nisar MF, Wan C. Characterization of phenolic constituents from prunus cerasifera ldb leaves. J Chem. 2020;2020:1–5. doi: 10.1155/2020/5976090.
- Wan C, Li S, Liu L, et al. Caffeoylquinic acids from the aerial parts of Chrysanthemum coronarium L. Plants. 2017;6(4):10. doi: 10.3390/plants6010010.
- Shu X, Wang M, Liu D, et al. Preparative separation of polyphenols from artichoke by polyamide column chromatography and high-speed counter-current chromatography. Quím Nova. 2013;36(6):836–839. doi: 10.1590/S0100-40422013000600017.
- El Hawary SSE, Fathy FI, El Kerdawy A, et al. Phytochemical profile and cytotoxic activity of selected organs of Sambucus nigra L. via enzyme assay and molecular docking study. Egypt J Chem. 2020;0(0):0–0. doi: 10.21608/ejchem.2020.31739.2674.
- Tošović J, Marković S. Structural and antioxidative features of chlorogenic acid. Croat Chem Acta. 2016;89(4):535–541. doi: 10.5562/cca3026.
- Yang J, Kwon YS, Kim MJ. Isolation and characterization of bioactive compounds from lepisorus thunbergianus (Kaulf.). Arabian J Chem. 2015;8(3):407–413. doi: 10.1016/j.arabjc.2014.11.056.
- Yuan M, Zhang G, Bai W, et al. The role of bioactive compounds in natural products extracted from plants in cancer treatment and their mechanisms related to anticancer effects. Oxid Med Cell Longev. 2022;2022:1429869–1429819. doi: 10.1155/2022/1429869.
- Fishbein A, Hammock BD, Serhan CN, et al. Carcinogenesis: failure of resolution of inflammation? Pharmacol Ther. 2021;218:107670. doi: 10.1016/j.pharmthera.2020.107670.
- Greenwell M, Rahman P. Medicinal plants: their use in anticancer treatment. Int J Pharm Sci Res. 2015;6(10):4103–4112. doi: 10.13040/IJPSR.0975-8232.6(10).4103-12.
- Pistritto G, Trisciuoglio D, Ceci C, et al. Apoptosis as anticancer mechanism: function and dysfunction of its modulators and targeted therapeutic strategies. Aging . 2016;8(4):603–619. doi: 10.18632/aging.100934.
- Hassin O, Oren M. Drugging p53 in cancer: one protein, many targets. Nat Rev Drug Discov. 2023;22(2):127–144. doi: 10.1038/s41573-022-00571-8.
- Neophytou CM, Trougakos IP, Erin N, et al. Apoptosis deregulation and the development of cancer multi-drug resistance. Cancers. 2021;13(17):4363. doi: 10.3390/cancers13174363.
- Negm W, Abo El-Seoud K, Kabbash A, et al. Investigation of the Biological activity some gymnosperm plants belong to cycadales order. J Adv Med Pharm Res. 2020;0(0):0–0. doi: 10.21608/jampr.2020.23512.1002.
- Ismail A, Hassan HM, Moawad AS, et al. Chemical composition and therapeutic potential of three Cycas species in brain damage and pancreatitis provoked by γ-radiation exposure in rats. J Radiat Res Appl Sci. 2020;13(1):38–52. doi: 10.1080/16878507.2020.1722908.
- Moawad A, Hetta M, Zjawiony JK, et al. Phytochemical investigation of Cycas circinalis and Cycas revoluta leaflets: moderately active antibacterial biflavonoids. Planta Med. 2010;76(8):796–802. doi: 10.1055/s-0029-1240743.
- Elmore S. Apoptosis: a review of programmed cell death. Toxicol Pathol. 2007;35(4):495–516. doi: 10.1080/01926230701320337.
- Alotaibi B, Negm WA, Elekhnawy E, et al. Antibacterial activity of nano zinc oxide green-synthesised from Gardenia thailandica triveng. Leaves against Pseudomonas aeruginosa clinical isolates: in vitro and in vivo study. Artif Cells Nanomed Biotechnol. 2022;50(1):96–106. doi: 10.1080/21691401.2022.2056191.
- Su TT. Non-apoptotic roles of apoptotic proteases: new tricks for an old dog. Open Biol. 2020;10(8):200130. doi: 10.1098/rsob.200130.
- Kumari R, Jat P. Mechanisms of cellular senescence: cell cycle arrest and senescence associated secretory phenotype. Front Cell Dev Biol. 2021;9:645593. doi: 10.3389/fcell.2021.645593.
- Hanahan D. Hallmarks of cancer: new dimensions. Cancer Discov. 2022;12(1):31–46. doi: 10.1158/2159-8290.CD-21-1059.
- Kari S, Subramanian K, Altomonte IA, et al. Programmed cell death detection methods: a systematic review and a categorical comparison. Apoptosis. 2022;27(7-8):482–508. doi: 10.1007/s10495-022-01735-y.
- Poon IK, Lucas CD, Rossi AG, et al. Apoptotic cell clearance: basic biology and therapeutic potential. Nat Rev Immunol. 2014;14(3):166–180. doi: 10.1038/nri3607.
- Battistelli M, Falcieri E. Apoptotic bodies: particular extracellular vesicles involved in intercellular communication. Biology. 2020;9(1):21. doi: 10.3390/biology9010021.
- Julian L, Olson MF. Apoptotic membrane dynamics in health and disease. CHC. 2015;14:133–142. doi: 10.2147/CHC.S57893.
- Lee S, Meng X, Flatten K, et al. Phosphatidylserine exposure during apoptosis reflects bidirectional trafficking between plasma membrane and cytoplasm. Cell Death Differ. 2013;20(1):64–76. doi: 10.1038/cdd.2012.93.
- Lecoeur H, PréVost M-C, Gougeon M-L. Oncosis is associated with exposure of phosphatidylserine residues on the outside layer of the plasma membrane: a reconsideration of the specificity of the annexin V/propidium iodide assay. Cytom: Je IntSoc Anal Cytol. 2001;44(1):65–72. doi: 10.1002/1097-0320(20010501)44:1<65::AID-CYTO1083>3.0.CO;2-Q.
- Samarghandian S, Shabestari MM. DNA fragmentation and apoptosis induced by safranal in human prostate cancer cell line. Indian J Urol. 2013;29(3):177–183. doi: 10.4103/0970-1591.117278.
- González-Marín C, Gosálvez J, Roy R. Types, causes, detection and repair of DNA fragmentation in animal and human sperm cells. Int J Mol Sci. 2012;13(11):14026–14052. doi: 10.3390/ijms131114026.
- Attallah NG, El-Sherbeni SA, El-Kadem AH, et al. Elucidation of the metabolite profile of Yucca gigantea and assessment of its cytotoxic, antimicrobial, and anti-inflammatory activities. Molecules. 2022;27(4):27–1329. doi: 10.3390/molecules27041329.
- Ozaki T, Nakagawara A. Role of p53 in cell death and human cancers. Cancers . 2011;3(1):994–1013. doi: 10.3390/cancers3010994.
- Almukainzi M, El-Masry TA, Negm WA, et al. Co-delivery of gentiopicroside and thymoquinone using electrospun m-PEG/PVP nanofibers: In-vitro and In vivo studies for antibacterial wound dressing in diabetic rats. Int J Pharmac. 2022;625:122106.
- Liebl MC, Hofmann TG. The role of p53 signaling in colorectal cancer. Cancers. 2021;13(9):2125. doi: 10.3390/cancers13092125.
- Qian S, Wei Z, Yang W, et al. The role of BCL-2 family proteins in regulating apoptosis and cancer therapy. Front Oncol. 2022;12:985363. doi: 10.3389/fonc.2022.985363.
- Kaloni D, Diepstraten ST, Strasser A, et al. BCL-2 protein family: attractive targets for cancer therapy. Apoptosis. 2023;28(1-2):20–38. doi: 10.1007/s10495-022-01780-7.
- Hussar P. Apoptosis regulators bcl-2 and caspase-3. Encyclopedia. 2022;2(4):1624–1636. doi: 10.3390/encyclopedia2040111.
- Negm WA, El-Aasr M, Kamer AA, et al. Investigation of the antibacterial activity and efflux pump inhibitory effect of Cycas thouarsii R. Br. Extract against Klebsiella pneumoniae clinical isolates. Pharmaceuticals. 2021;14(8):756. doi: 10.3390/ph14080756.
- Alotaibi B, Negm WA, Elekhnawy E, et al. Antibacterial, immunomodulatory, and lung protective effects of boswelliadalzielii oleoresin ethanol extract in pulmonary diseases: in vitro and in vivo studies. Antibiotics. 2021;10(12):1444. doi: 10.3390/antibiotics10121444.
- Attallah NG, El-Kadem AH, Negm WA, et al. Promising antiviral activity of agrimonia pilosa phytochemicals against severe acute respiratory syndrome coronavirus 2 supported with in vivo mice study. Pharmaceuticals. 2021;14(12):1313. doi: 10.3390/ph14121313.
- Ghasemi M, Turnbull T, Sebastian S, et al. The MTT assay: utility, limitations, pitfalls, and interpretation in bulk and single-cell analysis. Int J Mol Sci. 2021;22(23):12827. doi: 10.3390/ijms222312827.
- Malongane F, McGaw LJ, Olaokun OO, et al. Anti-inflammatory, anti-diabetic, anti-oxidant and cytotoxicity assays of South African herbal teas and bush tea blends. Foods. 2022;11(15):2233. doi: 10.3390/foods11152233.
- Alotaibi B, Mokhtar FA, El-Masry TA, et al. Antimicrobial activity of brassica rapa L. Flowers extract on gastrointestinal tract infections and antiulcer potential against indomethacin-Induced gastric ulcer in rats supported by metabolomics profiling. J Inflamm Res. 2021;14:7411–7430. doi: 10.2147/JIR.S345780.
- Rahman NA, Yazan LS, Wibowo A, et al. Induction of apoptosis and G 2/M arrest by ampelopsin E from dryobalanops towards triple negative breast cancer cells, MDA-MB-231. BMC Complement Altern Med. 2016;16(1):354. doi: 10.1186/s12906-016-1328-1.
- Noh J-I, Mun S-K, Lim EH, et al. Induction of apoptosis in MDA-MB-231 cells treated with the methanol extract of lichen physconia hokkaidensis. JoF. 2021;7(3):188. doi: 10.3390/jof7030188.
- Murad H, Hawat M, Ekhtiar A, et al. Induction of G1-phase cell cycle arrest and apoptosis pathway in MDA-MB-231 human breast cancer cells by sulfated polysaccharide extracted from laurencia papillosa. Cancer Cell Int. 2016;16(1):39. doi: 10.1186/s12935-016-0315-4.
- Pauzi AZM, Yeap SK, Abu N, et al. Combination of cisplatin and bromelain exerts synergistic cytotoxic effects against breast cancer cell line MDA-MB-231 in vitro. Chin Med. 2016;11(1):46. doi: 10.1186/s13020-016-0118-5.
- Koolivand M, Ansari M, Moein S, et al. The inhibitory effect of sulforaphane on the proliferation of acute myeloid leukemia cell lines through controlling miR-181a. Cell J . 2022;24:44.
- Ramadan MA, Shawkey AE, Rabeh MA, et al. Expression of P53, BAX, and BCL-2 in human malignant melanoma and squamous cell carcinoma cells after tea tree oil treatment in vitro. Cytotechnology. 2019;71(1):461–473. doi: 10.1007/s10616-018-0287-4.