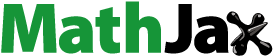
Abstract
Malaria is a mosquito-borne infectious disease that is caused by the Plasmodium parasite. Most of the available medication are losing their efficacy. Therefore, it is crucial to create fresh leads to combat malaria. Green silver nanoparticles (AgNPs) have recently attracted a lot of attention in biomedical research. As a result, green mediated AgNPs from leaves of Terminalia bellirica, a medicinal plant with purported antimalarial effects, were used in this investigation. Initially, cysteine-rich proteins from Plasmodium species were studied in silico as potential therapeutic targets. With docking scores between −9.93 and −11.25 kcal/mol, four leaf constituents of Terminalia bellirica were identified. The green mediated silver nanoparticles were afterward produced using leaf extract and were further examined using UV-vis spectrophotometer, DLS, Zeta potential, FTIR, XRD, and FESEM. The size of synthesized TBL-AgNPs was validated by the FESEM results; the average size of TBL-AgNPs was around 44.05 nm. The zeta potential study also supported green mediated AgNPs stability. Additionally, Plasmodium falciparum (3D7) cultures were used to assess the antimalarial efficacy, and green mediated AgNPs could effectively inhibit the parasitized red blood cells (pRBCs). In conclusion, this novel class of AgNPs may be used as a potential therapeutic replacement for the treatment of malaria.
Introduction
Malaria is a serious and sometimes fatal disease caused by the protozoan parasite Plasmodium. An infected female Anopheles mosquito transmits it to humans. Malaria afflicted 247 million people worldwide in 2021, with 6,19,000 deaths in tropical and subtropical regions, according to WHO [Citation1–3]. Malaria causes uneven high-grade fevers, anaemia, muscle spasms, and neurological problems such as severe brain injury and coma. Plasmodium falciparum (P. falciparum), Plasmodium vivax, Plasmodium ovale, Plasmodium malariae, and Plasmodium knowlesi are the most common parasites species that cause malaria [Citation4,Citation5]. Among the five Plasmodium species, Plasmodium falciparum causes the most deadly and lethal malaria for mammals, including humans. Malaria needs frequent screening and proper medication at the earliest to combat the disease. A few antimalarials, such as chloroquine, quinine, amodiaquine, mefloquine, atovaquone, halofantrine, primaquine, lumefantrine, etc., are available in the market, but most of them have adverse effects. The WHO-recommended artemisinin-based combination therapy (ACT) is the most effective antimalarial treatment. However, increased ACT resistance and side effects have been recorded in numerous South Asian and African regions [Citation6,Citation7]. Moreover, there is still no effective malaria vaccine available, despite the fact that clinical testing results for the RTS and S/AS01 vaccines are promising, and the new generation malaria vaccines (R21/Matrix-M) are currently in clinical trials [Citation4,Citation8].
In recent times, nanotechnology has gained tremendous global interest in dynamic engineering and material science to discover various promising technologies at the molecular level to fabricate metallic nanoparticles (NPs) [Citation9]. Silver nanoparticles (AgNPs) are particularly interesting in the current scenario due to their unique physicochemical properties such as shape, size distribution, surface charge, excellent catalytic activity, high electrical and thermal stability, and antibacterial activity [Citation9,Citation10]. Furthermore, their significance in biomedical applications such as anticancer [Citation11], antibacterial [Citation12], mosquitocidal action, antifungal [Citation13], antiinflammatory [Citation14], and antiviral activity [Citation15] has propelled them to the forefront of medical study. For the synthesis of NPs, there are two approaches: (a) top-down and (b) bottom-up [Citation16]. Physical, chemical, and biological approaches could all be used to create AgNPs. The chemical approach is quick, but it requires the employment of a reducing and capping chemical to create NPs, which is expensive, dangerous to humans, and detrimental to the environment. It creates a significant need for biologically synthesized AgNPs employing fungi, bacteria, and plants, which is simple, cost-effective, and environmentally benign. Because a large range of plant metabolites are specifically water-soluble flavones, quinones, and so on, which help to reduce silver nitrate salt (AgNO3), the universal solvent water is used to produce AgNPs utilizing plants [Citation10]. As a result, unlike bacteria and fungi, plant-based nanoparticles are currently an important area of study and have attracted great biotechnological attention due to their diverse applications; it does not require a sophisticated protocol such as maintaining sterile microbial cultures. Furthermore, plants are widely available, making them a more desirable resource for producing NPs than fungi and bacteria [Citation17]. Plant-mediated nanoparticle production is advantageous and promising in comparison to other approaches due to these distinct features [Citation18,Citation19]. The green synthesized AgNPs are used in the diverse biological application such as antimicrobial activity, insecticidal activity, antioxidant activity wound healing properties, antidiabetic activity, immunomodulatory activity, hepatoprotective activity and anticancer activity [Citation20,Citation21]. Recently, Avitabile et al. and Hawadak et al. reported the antimalarial activity against the P. falciparum parasite with the help of plant mediated AgNPs made up by Artemisia species and Azadirachta indica, respectively [Citation7,Citation22]. The actual mechanism of plant mediated NPs synthesis is unclear and unresolved, even though many researchers reported that some biomolecules from plants, like flavonoids, alkaloids, glycosides, proteins, phenolics, etc., play a significant role in the reduction of silver ions to their corresponding AgNPs. These biomolecules also serve as capping and stabilizing agents for the biologically synthesized AgNPs [Citation9,Citation23].
In our study, we have used an ayurvedic plant, Beric (Terminalia bellirica). It is a large deciduous tree in the Combretaceae family with broadly elliptical leaves that cluster at the branch tips. It is found across the world but is most common in the Indian subcontinent (Sri Lanka, Pakistan, Nepal, and Southeast Asia). It is widely used in conventional medicine due to its antiinflammatory, immunomodulatory, antioxidant, antibacterial, anticancer, and antidiabetic characteristics [Citation24]. The presence of several bioactive metabolites such as glucosides, tannins, gallic acid, ellagic acid, ethyl gallate, galloylglucose, chebulanic acid, and others is thought to be primarily responsible for their extensive therapeutic qualities that could be used as antimalarials [Citation10,Citation24]. Hence, synthesis of plant mediated AgNPs with anti-parasitic capabilities from Terminalia bellirica was done. For characterization of synthesized AgNPs, various techniques such as UV spectrophotometer, DLS, FTIR, zeta potential, XRD, and FESEM were utilized. Till now, many plant-mediated green synthesized AgNPs were tested against various infectious and non-infectious diseases but Terminalia bellirica leaves mediated AgNPs have not been broadly explored yet. In this study, for the first time, both computational (molecular docking) and experimental research, such as nanoparticle production, antimalarial activity, hemocompatibility, and MTT assay, were used to evaluate the efficacy of Terminalia bellirica leaves mediated silver nanoparticles (TBL-AgNPs) as potential drugs for malaria.
Materials and methodology
Silver nitrate (AgNO3, 99.9%, molecular weight =169.87), Dulbecco’s Modified Eagle Medium high glucose (DMEM HG), Foetal Bovine Serum (FBS), and 0.25% Trypsin-EDTA were procured from Life Technologies (Carlsbad CA), Gentamicin sulphate (Sigma-Aldrich), Hypoxanthine (Sigma-Aldrich), RPMI 1640 (Sigma-Aldrich), AlbuMAX I (Gibco™), HEPES 1 M (Sigma-Aldrich), Sorbitol (Sigma-Aldrich), Percoll (GE Healthcare), Dulbecco’s phosphate-buffered saline (PBS) deprived of calcium and magnesium chloride, sodium bicarbonate and resazurin sodium salt was obtained from Sigma-Aldrich. The filtration was done using Whatman filter paper grade 1 was used for filtration. The chemicals used in the research experiments were ACS grade ≥95% reagents.
The physiochemical characterization was done by UV-visible spectrophotometer (Thermo Scientific Evolution 201, Madison, USA), dynamic light scattering (DLS) and zeta potential (Malvern Zetasizer Nano), FTIR (Perkin Elmer Spectrum two), rotary evaporator (Hei-VAP Precision, Heidolph, Schwabach, Germany), X-ray crystallography (PANalytical-Empyrean, Almelo, The Netherlands), field emission scanning electron microscope (JEOL JSM-7400F, Tokyo, Japan), Centrifugation (UniCen MR Herolab GmbH)
Identification of target protein/s and retrieval of leaf constituents
According to the PlasmoDB database, the Plasmodium parasite contains approximately 5200 proteins and 1814 protein structures available in the Protein Databank (https://www.rcsb.org/).
In this study, cysteine and methionine rich domains of Plasmodium falciparum proteins were selected as drug targets because these amino acids contain a sulphur moiety that plays a significant role in the interaction of AgNPs, which results in the disruption of the tertiary and quaternary structure of the target proteins [Citation25–27]. In addition, literature also suggests that the AgNPs primarily interact with cysteine and methionine-rich regions of proteins [Citation25–27]. A Perl script was written to filter (protein with less than 800 amino acids and more than 15 cysteine residues) the cysteine-rich protein from the total Plasmodial crystallised proteins. 13 proteins were identified, and 4 proteins were selected (based on the availability of their binding/active site information) for further investigations. In addition, the chemical structure of 14 leaf constituents of Terminalia bellirica which are reported in the literature or available on the natural product database, were downloaded from PubChem (https://pubchem.ncbi.nlm.nih.gov/) [Citation28].
Molecular docking
Molecular docking is a powerful computational technique used to forecast the binding position of a ligand or drug in a target protein’s active site. It is a simple and inexpensive computational method for studying protein-ligand interactions. Molecular docking is now a well-accepted alternative to X-ray crystallography and NMR spectroscopy in studying protein-drug interactions. Several researchers have used the molecular docking approach to identify the exact binding confirmation of a small molecule with their potent targets [Citation29]. In this study, blind docking between the silver ion and the target malarial proteins was performed using AutoDock [Citation30,Citation31].
In contrast, site-specific docking between 14 leaf constituents of T. bellirica and targets was performed using Schrödinger software [Citation32]. In the docking studies, the target/s proteins were prepared by adding polar hydrogen, correcting bond orders and bond lengths, adding missing residues, removing water molecules, etc. In addition, the target proteins were minimized at neutral pH using the OPLS 2005 force field. Next, the plant constituents (without changing the chiral center) and silver ions were prepared. Further, a grid box was generated around the binding site (predicated using the Sitemap module of Schrödinger or information gathered from literatures) of the target protein using the Glide module of Schrödinger in the case of protein-small molecules docking studies, whereas a grid box was generated around the whole protein (blind docking) in the case of protein-silver ion docking using AutoDock tool. Finally, docking between targets and plant constituents/silver ions was analyzed carefully [Citation29,Citation33].
Plant sample collection and aqueous extract preparation
In April 2021, the Terminalia bellirica plant leaves were obtained from Fathebad (Latitude 29.52° N and longitude 75.45° E), Haryana, India. The Terminalia bellirica plant leaves (TBL) were washed with tap water and rinsed thrice with double distilled water (ddH2O) and dried in the shade for 12–16 days at room temperature (27–30 °C), and finally crushed with mortar and pestle. Accurately, 5 gm of the plant powder (leaves) were measured and mixed with 150 ml of ddH2O and put on the hot plate with a magnetic bead on a continuous stirring at 400 rpm (Stuart hot plate stirrer, model UC152) for 20 min at 60 °C. The obtained mixture was cooled at room temperature and filtered with the Whatman filter paper grade 1. The filtrate was collected and kept at 4 °C for the next 15–20 days maximum because of the gradual denaturation of the plant’s secondary phytometabolites in the aqueous extract at room temperature.
Optimization of reaction parameters
The biosynthesis process of AgNPs is sensitive and depends on several critical factors, i.e. reaction time, AgNO3 solution concentration, and reaction temperature [Citation34]. These factors contribute to qualitatively estimating the particle’s (green synthesized AgNPs) size, shape, yield, and aggregation phase. The present study has optimized these factors for the green synthesis of TBL-AgNPs. Initially, the time optimization reactions for TBL-AgNPs were performed at different time durations, i.e. 20, 40, 80, 120 and 160 min with a concentration of 1 mM AgNO3. Afterward, all reactions were centrifuged, washed thrice with ddH2O, and analyzed using a UV-visible spectrophotometer and further optimized.
Likewise, the concentration of AgNO3 was optimized using various AgNO3 concentrations, i.e. 0.5, 1, 1.5, 2 and 2.5 mM, to keep the other two factors (time and temperature) fixed. Furthermore, the amount of plant extract and the reaction temperature were optimized in the same way by maintaining various plant aqueous extract to AgNO3 solutions ratios (1:40, 1:20, 1:6.5 and 1:5) and different temperature conditions (20, 50, 70 and 100 °C) for the biosynthesis reaction.
Biosynthesis of green silver nanoparticles
In the AgNPs synthesis, an aqueous extract of leaves of Terminalia bellirica was added dropwise to the AgNO3 (1 mM) solution with constant stirring at 400 rpm at 50 °C for 80 min. The colour shift of the reaction mixture from pale yellow to dark brown produced by the reduction of Ag+ to Ag0 indicates the formation of AgNPs in Terminalia bellirica leaves (TBL-AgNPs) (). Subsequently, the TBL-AgNPs were accumulated after washing thrice with ddH2O by centrifugation at 10,000 rpm for 12 min. TBL-AgNPs were characterized via UV spectrophotometer, DLS, FTIR, zeta potential, XRD and FESEM techniques.
Blood stage culturing of Plasmodium falciparum
The blood-stage parasites were cultivated in-vitro by the standard procedure of using O+ve human erythrocytes taken from healthy voluntarily donated by individuals in the EDTA-coated sterile tubes [Citation34]. Using 5% sorbitol, parasites were synchronized regularly. A percoll gradient was used to enrich late-stage parasites [Citation35,Citation36].
In-vitro antimalarial activity of “green synthesized” AgNPs on the parasitized red blood cells (pRBCs)
Plasmodium falciparum strain 3D7 was used for in-vitro assay to determine the antiplasmodial effectiveness of T. bellirica leaves aqueous extract and their subsequent TBL-AgNPs. To perform the growth inhibitory assay (GIA), the ring stage intraerythrocytic parasites were grown in the presence or absence of the aqueous plant extract and green TBL-AgNPs with the corresponding control (Ctrl+/Ctrl−) for 48 h at a starting parasitemia of 1 and 1% Haematocrit, respectively. In order, pRBC were treated with the escalating amount of all aqueous plant extract and TBL-AgNPs (18.75, 37.5, 75, 150 and 300 ng/mL) at 48 h to determine the effect of parasite growth inhibition. For the scoring, the parasite numbers were smeared on the glass slides with Giemsa stained and calculated the parasitemia using the bright field microscope. The percentage of relative inhibition was calculated using the below formula [Citation37].% of relative inhibition = 100–(percent parasitemania in test / percent parasitemia in the control * 100)
Hemocompatibility assay
The haemolytic activities assay was carried out against the TBL-AgNPs according to the protocol by Elisabetta et al. [Citation7]. The previously acquired pRBCs cultures were used for haemolysis testing. Fresh RBCs were added to keep the pRBC cultures at 1% haematocrit. Freshly human heparinized whole blood was taken from the healthy individual volunteer donors. After 48 h of incubation, the plates were removed, and then RBCs were separated from blood by centrifugation at 200 g for 5 min to separate plasma and leukocytes. RBCs were subsequently washed three times in sterile complete growth media as described previously [Citation38–40]. The pRBCs cultures were preserved at 1–5% parasitemia (1% haematocrit) 37° C in a 95/5% (vol/vol) air/CO2 atmosphere. The haemolysis buffer (5 mmol/L sodium phosphate, 1 mmol/L EDTA, pH 8.0) was employed as the positive control (Ctrl+), whereas 1X PBS and 5 mM glucose were utilized as the negative control (Ctrl-). The below formula was used to identify the haemolytic analysis on the pRBCs TBL-AgNPs suspension (stock = 0.5 mg/mL of TBL-AgNPs) [Citation22].
Cell viability assay by MTT
Human embryonic kidney cell lines (HEK293) were obtained from NCCS, Pune, India, and cultured. The cells maintained viability in DMEM with 10% FBS and 1% (v/v) penicillin-streptomycin antibiotics, 1% L-glutamine, and 1% non-essential amino acids in an atmosphere of humidity containing 5% CO2 at 37 °C. The HEK293 cell lines were used to see the effect of the developed green mediated TBL-AgNPs. The HEK293 cells were seeded in a 96-cell culture plate at an initial concentration of 1 × 104 cells/well to investigate any possible cytotoxic effects under the above-described conditions. HEK293 cells were treated with different concentrations of 150, 200, 250, 300 and 600 ng/mL of TBL-AgNPs along with fresh culture media DMEM and 5% FBS, only (DMEM+ 5% FBS and 4 μL autoclaved ddH2O) as a negative control (Ctrl−). After TBL-AgNPs treatment, the cultured plate was incubated for 24 h to evaluate the cell viability count [Citation41]. MTT solution was prepared by dissolving the 3-(4,5-dimethylthiazol-2-yl)-2,5-diphenyl tetrazolium bromide at a concentration of 5 mg mL−1 in 1X PBS. After 24 h, 10 μL of MTT solution was added to each well, and the plate was further incubated for 4 h. Adding 100 μL dimethyl sulphoxide (DMSO) was used to dissolve the purple-colored crystals that had developed in the cell culture plate. The scanning of the cell culture plate was measured at 530 nm wavelength using a multimode microplate reader.
Statistical analysis
Data was entered into a spreadsheet (Microsoft Office, US) and subsequently exported in GraphPad v5.03 (GraphPad PRISM, California US) was used to generate a graph. All the experiments were conducted in triplicates (n = 3) and data are presented as mean ± standard deviation, and we used a t-test for the statistical studies with significant results of p < 0.05.
Result and discussion
Target selection and molecular docking
A total of 4 cysteine/methionine-rich proteins (PDB ID: 3SRI- Apical Membrane Antigen 1, 6PEV- M18 aspartyl aminopeptidase, 7KJ7- Pf12p, 3VGJ-tyrosyl-tRNA synthetase) were selected from Plasmodium species for in-silico investigations based on availability of information on their binding sites or their structures were co-crystallized with inhibitors. The particulars of active site/s of the chosen targets were extracted from the above-mentioned experimentally verified structures available in the PDB.
Molecular docking was performed between the silver ion and the selected target proteins. The top-ranked minimized protein-ion complexes were further analyzed using the AutoDock software, and we observed that the silver ion was present near the cysteine/methionine-rich region of the targets (). In addition, site-specific molecular docking studies were also performed between the selected protein-ion complex, and plant constitutes and investigated carefully. We found a few common compounds interacting with three target proteins out of four. Rutin (PubChem Id: 5280805) compound was efficiently interacting with M18 aspartyl aminopeptidase, tyrosyl-tRNA synthetase, and Pf12p, with an average docking score of −11.25 kcal/mol, and an average glide energy −114.98 kcal/mol. Similarly, Astragalin (PubChem Id: 5282102), Quercetin 3-O-alpha-L-rhamnopyranoside (PubChem Id: 5280459), and Isoquercitrin (PubChem Id: 5280804) were binding to the selected drug targets (M18 aspartyl aminopeptidase, tyrosyl-tRNA synthetase, and Pf12p) with docking score of −11.02, −10.45 and −9.93 kcal/mol and glide energy of −97.05, −104.80 and −91.22 kcal/mol, respectively. Rutin, Astragalin, Quercetin 3-O-alpha-L-rhamnopyranoside, and Isoquercitrin were forming hydrogen bonds with the binding site residues of the target proteins- M18 aspartyl aminopeptidase (Cys112, Tyr 175, Glu176, and His378), Pf12p (Asp61, Lys91, Ile172, Met173, Gln175, Tyr 188, Gly207, Asp209 and Lys247), and tyrosyl-tRNA synthetase (Gly5, His41 and Val43) and hydrophobic interactions with nearby residues of the active site (). The computational investigation suggests that these metabolites with a good docking score and glide energy bind efficiently with the selected drug targets and probably inhibit the growth of the parasite.
Optimization of TBL-AgNPs
The master reaction was optimized by various factors such as (plant extract and AgNO3 solutions ratios, reaction time, AgNO3 concentration and reaction temperature [Citation10,Citation34]. The stability of synthesized green TBL-AgNPs was carefully examined. Based on reaction optimization by various factors, we discovered that our TBL-AgNPs synthesis occurs at a concentration of 1 mM AgNO3, a temperature of 50 °C an incubation time 80 min, 1:20 ratio between TBL-PS aqueous extract and AgNO3 solutions, and continuous stirring at 400 rpm.
Physicochemical characterization of “green” mediated TBL-AgNPs
UV-visible spectrophotometer
The master reaction mixture was centrifuged, washed with ddH2O for stored at 4 °C, and evaluated further using UV-visible spectrophotometer. UV-vis spectroscopy was used to conduct a preliminary physicochemical assessment of the synthesized TBL-AgNPs, with a scan range of 300–700 nm. This type of study is an indirect way to confirming AgNPs development. It also provides the qualitative characteristics of AgNPs, such as their size, shape, production, and agglomeration conditions in a solution due to their unique optical properties. According to Truong et al. the AgNPs show the maxima absorbance peak at 427 nm and frequently have spherical shapes [Citation42]. Singh et al. reported a similar type of UV spectrum peak at 427 nm [Citation43]. The height of the absorption spectra increased in proportion to the concentration of AgNPs. The synthesized TBL-AgNPs were also scanned, and they showed distinct bands near 427 nm () and this confirms the formation of TBL-AgNPs [Citation44,Citation45].
Dynamic light scattering
Dynamic light scattering (DLS) is an analytical technique for determining the hydrodynamic particle size of AgNPs in liquid conditions. The average hydrodynamic size observed by DLS of TBL-AgNPs was 176.4 nm (). This includes the particle and suspension media, which greatly increases the actual size of the AgNPs observed. Our findings are like those of Paloma et al. who also observed a AgNPs size of 176.4 nm [Citation46,Citation47].
Zeta potential
Zeta potential (ZP) is a type of electric potential of AgNPs that emerges from the solution by forming a double electrical layer. ZP is typically examined for determining the surface charge and stability of AgNPs [Citation48]. When the NPs ZP ranges between −10 and +10 mV, it is considered neutral. When it is higher than +30 mV or lower than −30 mV, it is considered strongly cationic and anionic AgNPs, respectively. The harmful AgNPs are reported to be positively charged, while the least toxic AgNPs are negatively charged [Citation20,Citation49]. In general, the value of ZP between −30 mV to +30 mV indicates that good stability of AgNPs while the ZP values above this range, which indicate the aggregation, sedimentation and flocculation [Citation50]. The ZP result for the synthesized TBL-AgNPs revealed a net surface charge negative (−9.24 mV). Therefore, TBL-AgNPs are less toxic than strong cationic AgNPs. The −9.24 mV surface charge indicates a lower electrostatic barrier, which enhances the interaction between cell and silver nanoparticles (). TBL-AgNPs had low polydispersity index (PDI) of 0.395, indicating that the AgNPs were nearly identical [Citation51,Citation52].
FTIR
Fourier transform infra-red spectroscopy (FTIR) analysis was primarily used to determine the number of functional groups present in the plant aqueous extract, as well as any potential interactions that may have occurred during of AgNPs synthesis and play an important role as capping agents to make a stable AgNPs. It was accomplished by concentrating the TBL-AgNPs using the rotary evaporator after drying in the desiccator. The dry powder was combined with KBr to form a pellet, which was then scanned with a blank potassium bromide (KBr) pellet by an FTIR spectrometer within the range of 4000–400 cm−1 at the resolution 2 cm−1. FTIR spectrum of the TBL-AgNPs shows several prominent solid peaks in the 4000–400 cm−1 range. The distinct peak indicates that various aromatic compounds and proteins are involved in the interaction for the stability of TBL-AgNPs [Citation53]. The obtained TBL-AgNPs FTIR spectrum peak at 3300.34 and 3306.12 cm−1 suggesting the O–H stretching indicates the presence of alcoholic, phenolic or hydroxy compounds. The FTIR spectrum peak at 2104.19, 2126.86 cm−1 and 1638.15, 1636.18 cm−1 indicates that C = C and N–H stretching between the bonds, respectively [Citation54–56]. The finding of this study suggested the existence of the polyphenol, phytosterol, alkaloids, terpenoids, and flavonoids found on the surface of TBL-AgNPs, which served as reducing silver salt during the production of TBL-AgNPs and played an essential role in capping and stabilizing agents [Citation10,Citation57] (). The plant’s secondary metabolites are reported to have antimalarial activities. For example, quinine and artemisinin act as an antimalarial and widely used to combat malaria [Citation7,Citation54,Citation58–61]. The presence of reported metabolites on TBL-AgNPs might serve the purpose of antimalarial activity of the proposed nanoparticles.
XRD patterns
X-ray crystallography was used to determine the state of matter, which is crystallinity or amorphous at distinct angles. The XRD patterns also determine the cell dimension of synthesized AgNPs, with different Bragg’s peaks and sets of lattice planes. In the XRD result, the five pre-dominant peaks appear at 2 θ of 32.19°, 37.97°, 44.26°, 64.48° and 77.41° and their intensity at 172, 357, 997, 201, 255 and 249 arbitrary units (au), respectively for TBL-AgNPs (). The XRD peaks illustrate that TBL-AgNPs are face-centered cubic patterns and demonstrate that AgNPs synthesized by T. bellirica aqueous reduction of silver ions are crystalline in nature. Different types of reducing agents are present in the plant aqueous extract, which stabilizes the AgNPs and, as a result, provides the crystalline structure of the AgNPs, which has been comprehensively investigated in the many biosynthesized AgNPs [Citation21,Citation57]. The NPs size will considerably impact the XRD peak patterns The results presented here correspond with previous AgNPs studies conducted by Hemlata et al. [Citation62,Citation63].
FESEM experiment
Scanning electron microscope (SEM) experiments have been mainly used to examine the surface morphology, particle size distribution, crystal or particle structure, and texture of AgNPs. 1 ml solution of AgNPs was mixed by vortexing, dispersed on an aluminium stub, and determined from an operating distance of 3.2 mm. An SEI detector was used to take an image at 15 kV. The FESEM results showed the average size of synthesized TBL-AgNPs is 44.05 nm (). The image sufficiently demonstrates that these NPs were spherical in shape and did not aggregate. The FTIR results also show the presence of amino acid/proteins on the surface of AgNPs that protects them from agglomeration together (). AgNPs, which are in spherical shapes and have an average particle size of 40–50 nm, are more efficient and more specific [Citation10,Citation64–67]. Based on the biophysical characterization, we confirmed that the developed AgNPs from the leaf extract of T. bellirica were synthesized successfully and may be used for further investigations.
Antimalarial activity and haemolysis assay
Antimalarial activity and hemocompatibility assays have been tested on Plasmodium falciparum (3D7) cultures to determine the true impact of the produced AgNPs. In this experiment, the 3D7 strain culture was treated with escalating concentrations of T. bellirica aqueous plant extract and TBL-AgNPs (18.75, 37.5, 75, 150 and 300 ng/mL). Based on the experimental data, we observed that the produced TBL-AgNPs have significantly higher antimalarial activity than the plant aqueous extracts (). The IC50 values for TBL-AgNPs against the 3D7 strain were 94.70 ng/mL (). Several researchers examined the effect of “green” mediated NPs on the human pRBCs infected by P. falciparum We also compared the antimalarial activity of synthesized TBL-AgNPs with the previous research articles () [Citation7,Citation22]. We discovered that our synthesized TBL-AgNPs have a higher antimalarial activity at lower concentration when compared to previous AgNPs reports. Therefore, we also conducted haemolysis experiment and the haemolysis was negligible at the aforementioned IC50 (). Overall, TBL-AgNPs showed better antimalarial activity than the plant aqueous extract of leaves against the 3D7 strain, and in-vitro parasite growth inhibition was confirmed by light microscopic (). In addition, the developed TBL-AgNPs showed no toxicity to the normal cell line (HEK 293) ().
Figure 5. (A) Antimalarial activity of TBL-AgNPs in-vitro 3D7 strain. The percentage of parasite relative inhibition on pRBC was treated with an increasing dose (18.75–300 ng/mL) at 48 h of TBL-AgNPs, and (B) IC50 calculations of the TBL-AgNPs (IC50 = 94.70 ng/mL).
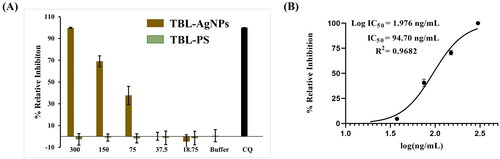
Figure 6. (A) Haemolysis assay on human pRBCs treated for 48 h at 37 °C with progressive doses of TBL-AgNPs and TBL-PS was found negligible, and (B) MTT assay cell viability counts on HEK293 cell lines. The TBL-AgNPs are not significantly affecting HEK239 at dosages of 150, 200, 250, 300, and 600 ng/mL.
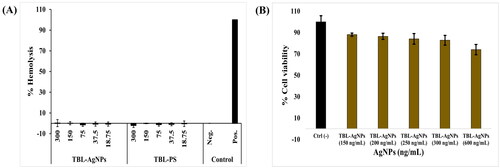
Figure 7. An aspect of culture showing with arrows parasite control at the ring stage. Culture was also used for all tested compounds. Aspect of culture after 48 h of incubation TBL-PS and TBL-AgNPs at 48 h of 300 ng/ml.
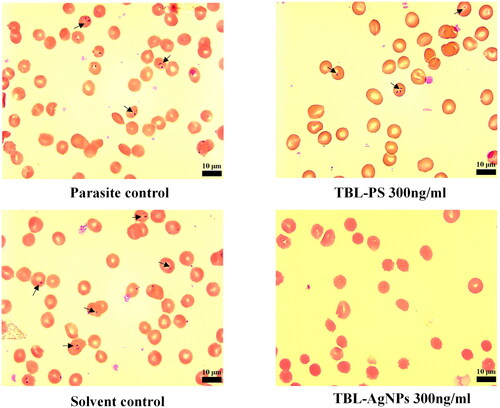
Table 1. Comparison the antimalarial activity of previous published literature with the current study result.
Conclusions
Malaria continues to be the most serious parasitic illness in many tropical and subtropical nations. The FDA has authorized various malaria medications, but most of them have resistance concerns. Green mediated NPs are essential in drug development programmes all over the world. These NPs are used to detect and cure diseases such as cancer, cancer treatment, neurological disease, HIV/AIDS, ophthalmic disease, and respiratory disease. This study effectively produced a green synthesis of colloidal phyto-functionalized AgNPs from T. bellirica leaf aqueous extract. TBL-AgNPs were synthesized and characterized using conventional procedures and were found to be within the acceptable range. They had a negative charge, were cubic in shape, and were crystalline. The synthesized AgNPs (TBL-AgNPs) were less than 45 nm in size. The in-vitro results exhibited potential antimalarial activity against P. falciparum (3D7) by TBL-AgNPs, probably due to its bioactive secondary metabolites that have the potential for antimalarial activity. In addition, in-silico studies of TBL-AgNPs binding to cysteine/methionine-rich P. falciparum proteins (M18 aspartyl aminopeptidase, tyrosyl-tRNA synthetase and Pf12p) revealed stable contacts (hydrogen and hydrophobic) with high docking and glide energy scores. In conclusion, the computational and experimental studies indicated that the synthesized TBL-AgNPs employing T. bellirica could be exploited as novel antimalarials drug. The extensive pre-clinical and clinical investigations will result in the development of a new and effective class of drug for the treatment of malaria.
Ethical approval
All authors have read and provided consent for the manuscript to be published.
Author contributions
Concept and design: SS and TKB. In-silico studies: HA, SS, BSA, MAG, and HHA. Data analysis and interpretation: SS, KBGH, MRH, and SR. In-vitro parasite culture: WS and KSR. Drafting of the paper: SS, HA, SN and TKB. Revision and intellectual critic: SN, BSA, MAG, HHA, KBGH, MRH, and SR. Supervision: TKB
Plant sample collection
Terminalia bellirica leaves of wild type plant were obtained from Fathebad, Haryana, India. The information for the same was given to competent authority of Central University of Rajasthan.
Acknowledgments
Sujeet Singh thanks the Department of Biotechnology, GOI, for project funding (BT/PR24504/NER/95/746/2017), Hemant Arya thanks the Indian Council of Medical Research, GOI, for the Research associate (ISRM/11(35)/2019), and the Central University of Rajasthan for providing infrastructure facilities.
Welka Sahu thanks Indian Council of Medical Research (ICMR), GOI, for the Senior Research Fellowship (2021-13362/CMB/BMS)
Disclosure statement
No potential conflict of interest was reported by the author(s).
Data availability statement
The published article contains all the data that was created or examined during this study.
Correction Statement
This article has been corrected with minor changes. These changes do not impact the academic content of the article.
Additional information
Funding
References
- Rajakumar G, Rahuman AA, Chung IM, et al. Antiplasmodial activity of eco-friendly synthesized palladium nanoparticles using Eclipta prostrata extract against Plasmodium berghei in Swiss Albino mice. Parasitol Res. 2015;114(4):1397–1406. doi: 10.1007/s00436-015-4318-1.
- Soni R, Sharma D, Rai P, et al. Signaling strategies of malaria parasite for its survival, proliferation, and infection during erythrocytic stage. Front Immunol. 2017;8:349. doi: 10.3389/fimmu.2017.00349.
- Soni R, Sharma D, Bhatt TK. Plasmodium falciparum secretome in erythrocyte and beyond. Front Microbiol. 2016;7:194. doi: 10.3389/fmicb.2016.00194.
- Tripathi H, Bhalerao P, Singh S, et al. Malaria therapeutics: are we close enough? Parasit Vectors. 2023;16(1):130. doi: 10.1186/s13071-023-05755-8.
- Sharma D, Soni R, Patel S, et al. In-silico studies on DegP protein of Plasmodium falciparum in search of anti-malarials. J Mol Model. 2016;22(9):201. doi: 10.1007/s00894-016-3064-3.
- Abuaku B, Duah-Quashie NO, Quashie N, et al. Trends and predictive factors for treatment failure following artemisinin-based combination therapy among children with uncomplicated malaria in Ghana: 2005–2018. BMC Infect Dis. 2021;21(1):1255. doi: 10.1186/s12879-021-06961-4.
- Hawadak J, Kojom Foko LP, Pande V, et al. In vitro antiplasmodial activity, hemocompatibility and temporal stability of Azadirachta indica silver nanoparticles. Artif Cells Nanomed Biotechnol. 2022;50(1):286–300. doi: 10.1080/21691401.2022.2126979.
- Takashima E, Tachibana M, Morita M, et al. Identification of novel malaria transmission-blocking vaccine candidates. Front Cell Infect Microbiol. 2021;11:805482. doi: 10.3389/fcimb.2021.805482.
- Okaiyeto K, Hoppe H, Okoh AI. Plant-based synthesis of silver nanoparticles using aqueous leaf extract of Salvia officinalis: characterization and its antiplasmodial activity. J Clust Sci. 2021;32(1):101–109. doi: 10.1007/s10876-020-01766-y.
- Arya G, Kumari RM, Gupta N, et al. Green synthesis of silver nanoparticles using prosopis juliflora bark extract: reaction optimization, antimicrobial and catalytic activities," (in eng). Artif Cells Nanomed Biotechnol. 2018;46(5):985–993. doi: 10.1080/21691401.2017.1354302.
- Bhatnagar S, Kobori T, Ganesh D, et al. Biosynthesis of silver nanoparticles mediated by extracellular pigment from talaromyces purpurogenus and their biomedical applications. Nanomaterials. 2019;9(7):1042. doi: 10.3390/nano9071042.
- Balachandar R, Gurumoorthy P, Karmegam N, et al. Plant-mediated synthesis, characterization and bactericidal potential of emerging silver nanoparticles using stem extract of Phyllanthus pinnatus: a recent advance in phytonanotechnology. J Clust Sci. 2019;30(6):1481–1488. doi: 10.1007/s10876-019-01591-y.
- Jamdagni P, Khatri P, Rana J-SJ. Green synthesis of zinc oxide nanoparticles using flower extract of nyctanthes arbor-tristis and their antifungal activity. J King Saud Univ Sci. 2018;30(2):168–175. doi: 10.1016/j.jksus.2016.10.002.
- Singh P, Ahn S, Kang J-P, et al. In vitro anti-inflammatory activity of spherical silver nanoparticles and monodisperse hexagonal gold nanoparticles by fruit extract of Prunus serrulata: a green synthetic approach. Artif Cells Nanomed Biotechnol. 2018;46(8):2022–2032. doi: 10.1080/21691401.2017.1408117.
- Haggag EG, Elshamy AM, Rabeh MA, et al. Antiviral potential of green synthesized silver nanoparticles of Lampranthus coccineus and Malephora lutea. Int J Nanomedicine. 2019;14:6217–6229. doi: 10.2147/ijn.S214171.
- Arya G, Kumari RM, Sharma N, et al. Catalytic, antibacterial and antibiofilm efficacy of biosynthesised silver nanoparticles using Prosopis juliflora leaf extract along with their wound healing potential. J Photochem Photobiol B. 2019;190:50–58. doi: 10.1016/j.jphotobiol.2018.11.005.
- Galatage ST, Hebalkar AS, Dhobale SV, et al. Silver nanoparticles: properties, synthesis, characterization, applications and future trends In Silver Micro-Nanoparticles - Properties, Synthesis, Characterization, and Applications, Edited by Kumar S, Kumar P, Pathak CS 2021;266. DOI: 10.5772/intechopen.99173.
- Sharma N, Singhal M, Kumari RM, et al. Diosgenin loaded polymeric nanoparticles with potential anticancer efficacy. Biomolecules. 2020;10(12):1679.) doi: 10.3390/biom10121679.
- Vijayaram S, Razafindralambo H, Sun YZ, et al. Applications of green synthesized metal nanoparticles—a review. Biologic. Trace Element Res. 2023;202:1–27. https://doi.org/10.1007/s12011-023-03645-9.
- Druzian DM, Machado AK, Pappis L, et al. Synthesis, characterization, cytotoxicity and antimicrobial activity of a nanostructured mineral clay. Ceram Int. 2023;49(19):31066–31076. doi: 10.1016/j.ceramint.2023.07.051.
- Pinheiro LDSM, Sentena NZ, Sangoi GG, et al. Copper nanoparticles from acid ascorbic: biosynthesis, characterization, in vitro safety profile and antimicrobial activity. 2023; 307:128110. https://doi.org/10.1016/j.matchemphys.2023.128110.
- Avitabile E, Senes N, D’Avino C, et al. The potential antimalarial efficacy of hemocompatible silver nanoparticles from Artemisia species against P. falciparum parasite (in eng). PLOS One. 2020;15(9):e0238532. doi: 10.1371/journal.pone.0238532.
- Behravan M, Hossein Panahi A, Naghizadeh A, et al. Facile green synthesis of silver nanoparticles using berberis vulgaris leaf and root aqueous extract and its antibacterial activity. Int J Biol Macromol. 2019;124:148–154. doi: 10.1016/j.ijbiomac.2018.11.101.
- Gahatraj S, Bhusal B, Sapkota K, et al. Common medicinal plants of Nepal: a review of Triphala: Harro (Terminalia chebula), Barro (Terminalia Bellirica), and Amala (Emblica Officinalis). Asian J.Pharmacogn. 2020;4(3):5–13.
- Mnkandhla D, Marwijk JV, Hoppe H, et al. In vivo; in vitro interaction of silver nanoparticles with leucine aminopeptidase from human and Plasmodium falciparum. J Nanosci Nanotechnol. 2018;18(2):865–871. doi: 10.1166/jnn.2018.13966.
- Klein MM, Gittis AG, Su H-P, et al. The cysteine-rich interdomain region from the highly variable plasmodium falciparum erythrocyte membrane protein-1 exhibits a conserved structure. PLOS Pathog. 2008;4(9):e1000147. doi: 10.1371/journal.ppat.1000147.
- De Moor W, van Marwijk J, Wilhelmi BS, et al. Interaction of silver nanoparticles with triosephosphate isomerase from human and malarial parasite (Plasmodium falciparum): a comparative study. J Biomed Nanotechnol. 2015;11(6):1071–1079. doi: 10.1166/jbn.2015.2003.
- Kumar N, Khurana SMP. Phytochemistry and medicinal potential of the Terminalia bellirica roxb.(bahera). Indian J Nat Prod Resour. 2018;9(2):97–107.
- Arya H, Syed SB, Singh SS, et al. In silico investigations of chemical constituents of Clerodendrum colebrookianum in the anti-hypertensive drug targets: ROCK, ACE, and PDE5. Interdiscip Sci. 2018;10(4):792–804. doi: 10.1007/s12539-017-0243-6.
- Forli S, Huey R, Pique ME, et al. Computational protein-ligand docking and virtual drug screening with the AutoDock suite. Nat Protoc. 2016;11(5):905–919. doi: 10.1038/nprot.2016.051.
- Karthik CS, Chethana MH, Manukumar HM, et al. Synthesis and characterization of chitosan silver nanoparticle decorated with benzodioxane coupled piperazine as an effective anti-biofilm agent against MRSA: a validation of molecular docking and dynamics. Int J Biol Macromol. 2021;181:540–551. doi: 10.1016/j.ijbiomac.2021.03.119.
- Indari O, Kumar Singh A, Tiwari D, et al. Deciphering antiviral efficacy of malaria box compounds against malaria exacerbating viral pathogens-Epstein Barr virus and SARS-CoV-2, an in silico study. Med Drug Discov. 2022;16:100146. doi: 10.1016/j.medidd.2022.100146.
- Arya H, Yadav CS, Lin S-Y, et al. Design of a potent anticancer lead inspired by natural products from traditional indian medicine. J Biomol Struct Dyn. 2020;38(12):3563–3577. doi: 10.1080/07391102.2019.1664326.
- Ahmed Q, Gupta N, Kumar A, et al. Antibacterial efficacy of silver nanoparticles synthesized employing terminalia arjuna bark extract. Artif Cells Nanomed Biotechnol. 2017;45(6):1–9. doi: 10.1080/21691401.2016.1215328.
- Radfar A, Méndez D, Moneriz C, et al. Synchronous culture of Plasmodium falciparum at high parasitemia levels. Nat Protoc. 2009;4(12):1899–1915. doi: 10.1038/nprot.2009.198.
- Sahu W, Bai T, Panda PK, et al. Plasmodium falciparum HSP40 protein eCiJp traffics to the erythrocyte cytoskeleton and interacts with the human HSP70 chaperone HSPA1. FEBS Lett. 2022;596(1):95–111. doi: 10.1002/1873-3468.14255.
- Reddy KS, Amlabu E, Pandey AK, et al. Multiprotein complex between the GPI-anchored CyRPA with PfRH5 and PfRipr is crucial for ierythrocyte invasion," (in eng). Proc Natl Acad Sci USA. 2015;112(4):1179–1184. doi: 10.1073/pnas.1415466112.
- De Lucia S, Tsamesidis I, Pau MC, et al. Induction of high tolerance to artemisinin by sub-lethal administration: a new in vitro model of P. falciparum. PLOS One. 2018;13(1):e0191084. doi: 10.1371/journal.pone.0191084.
- Lambros C, Vanderberg JP. Synchronization of Plasmodium falciparum erythrocytic stages in culture. J Parasitol. 1979;65(3):418–420. doi: 10.2307/3280287.
- Trager W, Jensen JB. Human malaria parasites in continuous culture. Science. 1976;193(4254):673–675. doi: 10.1126/science.781840.
- Ahmed T, Shahid M, Noman M, et al. Bioprospecting a native silver-resistant Bacillus safensis strain for green synthesis and subsequent antibacterial and anticancer activities of silver nanoparticles. J Adv Res. 2020;24:475–483. doi: 10.1016/j.jare.2020.05.011.
- Trung TT, Van Cuong N, Hong LTT, et al. Study on synthesizing silver nanoparticles by using Muntingia calabura leaf extract: insights from experimental and theoretical studies. Vietnam J Chem. 2021;59(5):606–611. doi: 10.1002/vjch.202100012.
- Singh S, Bharti A, Meena VKJ. Green synthesis of multi-shaped silver nanoparticles: optical, morphological and antibacterial properties. J Mater Sci Mater Electron. 2015;26(6):3638–3648. doi: 10.1007/s10854-015-2881-y.
- Pradeepa M, Harini K, Ruckmani K, et al. Extracellular bio-inspired synthesis of silver nanoparticles using raspberry leaf extract against human pathogens. Int. J. Pharm. Sci. Rev. Res. 2014;25(2):160–165.
- Ajitha B, Reddy YAK, Reddy PS, et al. Instant biosynthesis of silver nanoparticles using Lawsonia inermis leaf extract: innate catalytic, antimicrobial and antioxidant activities. J Mol Liq. 2016;219:474–481. doi: 10.1016/j.molliq.2016.03.041.
- Pabisch S, Feichtenschlager B, Kickelbick G, et al. Effect of interparticle interactions on size determination of zirconia and silica based systems – a comparison of SAXS, DLS, BET, XRD and TEM. Chem Phys Lett. 2012;521(C):91–97. doi: 10.1016/j.cplett.2011.11.049.
- Serrano-Díaz P, Williams DW, Vega-Arreguin J, et al. Geranium leaf-mediated synthesis of silver nanoparticles and their transcriptomic effects on Candida albicans. Green Process. Syn. 2023;12(1):20228105.
- Sizochenko N, Mikolajczyk A, Syzochenko M, et al. Zeta potentials (ζ) of metal oxide nanoparticles: a meta-analysis of experimental data and a predictive neural networks modeling. NanoImpact. 2021;22:100317. doi: 10.1016/j.impact.2021.100317.
- El Badawy AM, Silva RG, Morris B, et al. Surface charge-dependent toxicity of silver nanoparticles. Environ Sci Technol. 2011;45(1):283–287. doi: 10.1021/es1034188.
- Kojom Foko LP, et al. A systematic review on anti-malarial drug discovery and antiplasmodial potential of green synthesis mediated metal nanoparticles: overview. Challen Fut Perspect. 2019;18:1–14.
- Saini P, Saha SK, Roy P, et al. Evidence of reactive oxygen species (ROS) mediated apoptosis in Setaria cervi induced by green silver nanoparticles from Acacia auriculiformis at a very low dose. Exp Parasitol. 2016;160:39–48. doi: 10.1016/j.exppara.2015.11.004.
- Alkholief M, Kalam MA, Alshememry AK, et al. Topical application of linezolid-loaded chitosan nanoparticles for the treatment of eye infections. Nanomaterials. 2023;13(4); 681. doi: 10.3390/nano13040681.
- Arya G, Kumari R, Pundir R, et al. Versatile biomedical potential of biosynthesized silver nanoparticles from Acacia nilotica bark. J Appl Biomed. 2019;17(2):115–124. doi: 10.32725/jab.2019.010.
- Macovei I, Luca SV, Skalicka-Woźniak K, et al. Phyto-functionalized silver nanoparticles derived from conifer bark extracts and evaluation of their antimicrobial and cytogenotoxic effects. Molecules. 2021;27(1):217. doi: 10.3390/molecules27010217.
- Benedec D, Oniga I, Cuibus F, et al. Origanum vulgare mediated green synthesis of biocompatible gold nanoparticles simultaneously possessing plasmonic, antioxidant and antimicrobial properties. Int J Nanomedicine. 2018;13:1041–1058. doi: 10.2147/ijn.S149819.
- Rahman H, Rauf A, Khan SA, et al. Green synthesis of silver nanoparticles using Rhazya stricta Decne extracts and their anti-microbial and anti-oxidant activities. Crystals. 2023;13(3):398. doi: 10.3390/cryst13030398.
- Jeeva K, Thiyagarajan M, Elangovan V, and Products, et al. Caesalpinia coriaria leaf extracts mediated biosynthesis of metallic silver nanoparticles and their antibacterial activity against clinically isolated pathogens. Ind Crops Prod. 2014;52:714–720. doi: 10.1016/j.indcrop.2013.11.037.
- Gupta SC, Prasad S, Tyagi AK, et al. Neem (Azadirachta indica): an Indian traditional panacea with modern molecular basis. Phytomedicine. 2017;34:14–20. doi: 10.1016/j.phymed.2017.07.001.
- Bekono BD, Ntie-Kang F, Onguéné PA, et al. The potential of anti-malarial compounds derived from African medicinal plants: a review of pharmacological evaluations from 2013 to 2019. Malar J. 2020;19(1):183. doi: 10.1186/s12936-020-03231-7.
- Othman L, Sleiman A, Abdel-Massih RM. Antimicrobial activity of polyphenols and alkaloids in Middle Eastern plants. Front Microbiol. 2019;10:911. doi: 10.3389/fmicb.2019.00911.
- Phillipson JD, Wright CW. Antiprotozoal agents from plant sources. Planta Med. 1991;57(7 Suppl):S53–S59. doi: 10.1055/s-2006-960230.
- Meena PR, Singh AP, Tejavath KK, et al. Biosynthesis of silver nanoparticles using Cucumis prophetarum aqueous leaf extract and their antibacterial and antiproliferative activity against cancer cell lines. ACS Omega. 2020;5(10):5520–5528, doi: 10.1021/acsomega.0c00155.
- Shankar SS, Rai A, Ahmad A, et al. Rapid synthesis of Au, Ag, and bimetallic Au core-Ag shell nanoparticles using neem (Azadirachta indica) leaf broth. J Colloid Interface Sci. 2004;275(2):496–502. doi: 10.1016/j.jcis.2004.03.003.
- Gomathi M, Rajkumar P, Prakasam A, et al. Green synthesis of silver nanoparticles using Datura stramonium leaf extract and assessment of their antibacterial activity. Resour-Effic Technol. 2017;3(3):280–284. doi: 10.1016/j.reffit.2016.12.005.
- Alyousef AA, Arshad M, AlAkeel R, et al. Biogenic silver nanoparticles by Myrtus communis plant extract: biosynthesis, characterization and antibacterial activity. Biotechnol Biotechnol Equip. 2019;33(1):931–936. doi: 10.1080/13102818.2019.1629840.
- Muthukumaran U, Govindarajan M, Rajeswary M, et al. Synthesis and characterization of silver nanoparticles using Gmelina asiatica leaf extract against filariasis, dengue, and malaria vector mosquitoes. Parasitol Res. 2015;114(5):1817–1827. doi: 10.1007/s00436-015-4368-4.
- Zhao Y, Wang Y, Ran F, et al. A comparison between sphere and rod nanoparticles regarding their in vivo biological behavior and pharmacokinetics. Sci Rep. 2017;7(1):4131. doi: 10.1038/s41598-017-03834-2.