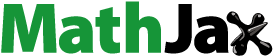
Abstract
Haemorrhagic shock is a leading cause of death worldwide. Blood transfusions can be used to treat patients suffering severe blood loss but donated red blood cells (RBCs) have several limitations that limit their availability and use. To solve the problems associated with donated RBCs, several acellular haemoglobin-based oxygen carriers (HBOCs) have been developed to restore the most important function of blood: oxygen transport. One promising HBOC is the naturally extracellular haemoglobin (i.e. erythrocruorin) of Lumbricus terrestris (LtEc). The goal of this study was to maximise the portability of LtEc by lyophilising it and then testing its stability at elevated temperatures. To prevent oxidation, several cryoprotectants were screened to determine the optimum formulation for lyophilisation that could minimise oxidation of the haem iron and maximise recovery. Furthermore, samples were also deoxygenated prior to storage to decrease auto-oxidation, while resuspension in a solution containing ascorbic acid was shown to partially reduce LtEc that had oxidised during storage (e.g. from 42% Fe3+ to 11% Fe3+). Analysis of the oxygen equilibria and size of the resuspended LtEc showed that the lyophilisation, storage, and resuspension processes did not affect the oxygen transport properties or the structure of the LtEc, even after 6 months of storage at 40 °C. Altogether, these efforts have yielded a shelf-stable LtEc powder that can be stored for long periods at high temperatures, but future animal studies will be necessary to prove that the resuspended product is a safe and effective oxygen transporter in vivo.
Introduction
Haemorrhagic shock caused 1.9 million deaths per year between 2000 and 2015 (1.5 million of which were related to physical trauma), making it a leading cause of death worldwide [Citation1,Citation2]. Blood transfusions are often the preferred treatment option for patients suffering severe blood loss [Citation3], but donor red blood cells (RBCs) must be constantly refrigerated and have a finite shelf life of ∼42 days that limits their availability [Citation4]. In addition to these storage issues, the logistical challenges associated with cold chain transport of refrigerated RBCs over vast distances are formidable, especially in austere environments [Citation5–7]. Consequently, while it is possible to deploy donor RBCs for some military operations, most of those units are ultimately discarded before they can be utilised (e.g. ∼95% of units were discarded during the first Persian Gulf War) [Citation8].
To solve the problems associated with the storage and transport of donated RBCs, several acellular haemoglobin-based oxygen carriers (HBOCs) with prolonged shelf lives and other favourable characteristics have been developed, including human and bovine hemoglobins [Citation9]. Another promising HBOC is the naturally extracellular haemoglobin (also known as erythrocruorin, Ec) of Lumbricus terrestris (LtEc). LtEc offers several advantages over adult human haemoglobin (HbA), including a lower auto-oxidation rate, intramolecular disulphide bonds that prevent subunit dissociation, and a relatively high molecular weight (3.6 MDa) that prevents extravasation and prolongs its circulation half-life. [Citation10] LtEc also has a high thermal stability (Tm = 55 °C), suggesting that it may not need to be refrigerated like donor RBCs [Citation11]. Finally, the oxygen affinity of LtEc (P50 = 28 mm Hg) is similar to human RBCs (P50 = 26 mm Hg), indicating that O2 delivery by LtEc in vivo may closely mimic RBC O2 transport [Citation10,Citation12]. Indeed, LtEc has successfully been transfused into hamsters experiencing haemorrhagic shock without any adverse side effects [Citation13,Citation14].
The goal of this project was to further improve the portability of LtEc by lyophilising it into a lightweight powder that can be stored easily without refrigeration and then resuspended quickly using saline or similar rehydration fluids that are carried by first responders [Citation15–18]. Lyophilisation is an excellent way to store proteins, since the removal of water from the protein helps to avoid hydrolysis, oxidation, aggregation, and disulphide rearrangements [Citation15,Citation19]. Multiple blood products have previously been lyophilised. For example, lyophilised plasma was developed during World War II [Citation15] to save the lives of thousands of Allied troops. Production of lyophilised plasma was discontinued in the latter half of the twentieth century due to the inability to remove pathogens (e.g. hepatitis and HIV) from donated plasma, [Citation7,Citation20] but recent advances in pathogen removal have resulted in the reintroduction of lyophilised plasma in France, South Africa, and Germany [Citation7,Citation20].
In addition to plasma, several studies have demonstrated the lyophilisation of RBCs and HBOCs [Citation15]. These studies have shown that RBCs are prone to haemolysis and other damaging effects that occur during freeze/thaw processes [Citation15,Citation21–25], but the addition of cryoprotectants can solve these problems [Citation15,Citation21,Citation22,Citation26]. For example, RBCs have been preserved using glycerol, while haemoglobin and other proteins have been preserved using sucrose and trehalose [Citation15,Citation21,Citation22,Citation26–28]. Although their exact mechanisms are currently unknown, it is possible that these additives limit physical changes in the RBCs and HBOCs during the freezing process [Citation7,Citation15,Citation18,Citation29,Citation30]. For example, non-reducing sugars (such as sucrose and trehalose) create an amorphous boundary around proteins, which helps to preserve the tertiary structure of the protein [Citation15,Citation18,Citation29]. Sugars, along with polyols like glycerol and mannitol, also form a glassy matrix around proteins which limits mobility during the freezing and lyophilisation process [Citation15,Citation18,Citation29]. Polyols may assist by making this matrix more dense [Citation18].
Indeed, Zal et al. previously patented a method to lyophilise the erythrocruorin of Arenicola marina (AmEc) for use as an RBC substitute using additives such trehalose, sucrose, and mannitol [Citation31]. Their work showed that lyophilised AmEc retained its structural integrity and was able to transport oxygen after being resuspended [Citation26]. However, this patent only monitored sample storage over 5 days at 37 °C [Citation26]. Palmer et al. demonstrated successful lyophilisation of LtEc using trehalose, but that study only investigated room temperature storage [Citation32]. In this work, we sought to optimise an improved lyophilisation method for LtEc that provides a lyophilised LtEc product that can be stored for up to 6 months at 40 °C without affecting its structure or activity. Specifically, lyophilisation, storage, and resuspension protocols were developed which minimised the amount of haem oxidation (<20% Fe3+) during storage while maximising the recovery of soluble LtEc (>90%) in its fully assembled and reduced (Fe2+) form.
Materials and methods
Materials
Cryoprotectants were purchased from Sigma-Aldrich (St. Louis, MO), including sucrose (catalog #S2395), trehalose (#T0167), mannitol (#M4125), 1,4-dithiothreitol (#10197777001), and ascorbic acid (#A92902). Earthworms were purchased from Wholesale Bait Supply (Hamilton, OH) and LtEc was purified to homogeneity with minimal oxidation (%Fe3+ = 5–10%) via tangential flow filtration as previously described [Citation11,Citation33,Citation34].
Freezing and lyophilization
Aqueous solutions of LtEc (0.8 mM haem) were prepared in 20 mM Tris buffer (pH 7.4) by either combining the LtEc with one of the cryoprotectants or diluting control samples with ultrapure water. Samples were then flash-frozen in liquid nitrogen for 5 min, as described for other proteins [Citation35,Citation36], and lyophilised using a VirTis BenchTop Pro (SP Scientific, Warminster, PA).
Deoxygenation and long-term storage
After lyophilisation, samples were either resuspended immediately to perform preliminary quality checks on the lyophilisation process or stored for extended periods of time. Deoxygenated samples were prepared by transferring LtEc powder into flasks that were connected to a Schlenk line. The samples were then alternately flushed with grade 5.0 N2 gas (Keen Gas, Wilmington, DE) for 2 min at low pressure and exposed to vacuum for 2 min. This process was repeated at least 20 times to remove as much oxygen from the samples as possible. Deoxygenation of the samples was monitored by adding aqueous LtEc to one of the flasks connected to the Schlenk line. Specifically, after the alternating N2 and vacuum steps were completed, the aqueous LtEc sample was anaerobically transferred via cannula to a quartz cuvette that was prefilled with N2. That sample was then analysed with a Biotek Synergy UV-Vis spectrophotometer (Agilent, Santa Clara, CA) to measure its absorbance spectrum and confirm that the q-bands present at 540 and 576 nm in oxygenated LtEc had been completely replaced with a broad peak at 560 nm that is representative of deoxygenated LtEc (as shown in ). If deoxygenation was incomplete, the samples were exposed to more cycles of N2 and vacuum. Otherwise, the deoxygenated samples were subsequently sealed under nitrogen, detached from the Schlenk line, and stored at room temperature or 40 °C.
Resuspension
Lyophilised samples were resuspended in ultrapure water, Ringer’s lactate solution with 12.25 mM N-acetyl-L-cysteine (pH 7.4), phosphate buffered saline (PBS, 1X concentration, pH 7.4), or Tris buffer (20 mM, pH 7.4) to test the effects of buffer composition on the recovery of soluble LtEc. However, no difference in recovery or oxidation level was observed between those buffers in preliminary tests (data not shown, see Supplemental Information Section 3: ), so Tris buffer was used for subsequent resuspension steps as it was the simplest formulation. Specifically, samples were exposed to room air and then resuspended with 785 µL of 20 mM Tris (pH 7.4) at room temperature, briefly vortexed for 5 s, and then centrifuged at 10,000 g for 5 min at 4 °C to remove any aggregates. When samples were resuspended with a buffer containing ascorbic acid, the buffer strength and pH were increased (50 mM Tris, pH 7.6) to stabilise the pH of the solution against acidification by the ascorbic acid. The supernatants were then analysed using UV-Vis spectrophotometry to measure the oxidation level of the sample (%Fe3+) and the recovery of soluble LtEc. Finally, 5 mM ascorbic acid (AA) was also added to the samples to reduce LtEc that oxidised during storage [Citation11].
Oxidation assay and recovery
The oxidation level (%Fe3+) and yield of the resuspended LtEc samples were determined spectroscopically using a Biotek Synergy HT microplate reader (Agilent, Santa Clara, CA). Approximately 100 µL of each sample was transferred to a 96 well plate, then the absorbance spectrum of the sample was immediately recorded from 450 to 700 nm. The oxidation levels of each sample were then calculated using EquationEquation 1(1)
(1) , while the concentration of soluble LtEc was calculated using the Beer-Lambert Law (A = εCL). It is important to note that different extinction coefficients had to be used for each sample, since the cryoprotectants and ascorbic acid significantly affected the pH and absorbance spectra of the samples. Please refer to Section 1 of the Supplementary Information for the specific isosbestic points and extinction coefficients used for each type of sample (, ).
(1)
(1)
Figure 2. Effects of additives (sucrose, mannitol, and a combination thereof) on oxidation during lyophilisation and immediate resuspension. Control samples contained only Tris buffer with no additives. Bars represent the mean (n = 3), while error bars represent one standard deviation. Letters indicate significantly different groups as determined by ANOVA (n = 45, F11,33 = 113.9, p < 0.05).
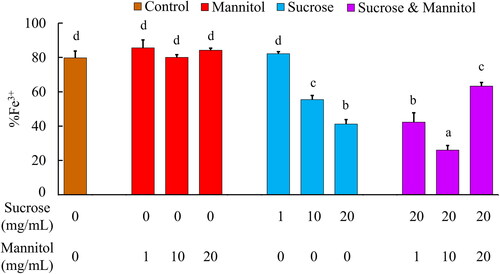
Figure 3. Effects of deoxygenation and resuspension with ascorbic acid on the oxidation levels (A) and absorbance spectra (B) of lyophilised samples that were stored at 40 °C for 2 months. Error bars indicate standard deviation. Letters indicate significantly different groups as determined by ANOVA (n = 12, p < 0.05).
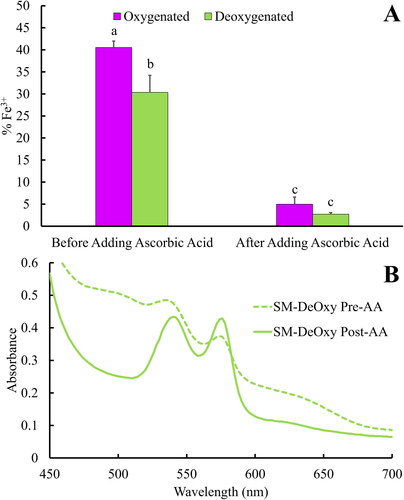
Table 1. Summary of oxygen affinity (P50) and cooperativity (n) for lyophilised LtEc. Mean ± one standard deviation. Letters indicate significantly different groups as determined by ANOVA (P50) or K-W test (n) using p < 0.05.
Subunit dissociation
A Shimadzu high-pressure liquid chromatography system was used to detect dissociation of the LtEc hexagonal bilayer structure (180 subunits, 3.6 MDa, 30 nm diameter) into smaller oligomers and subunits during the lyophilisation, storage, or resuspension steps. Samples were passed through a size-exclusion column (Tosoh Bioscience USA, San Francisco, CA, #0022856) using a mobile phase of 20 mM Tris-HCl (pH 7.4) at a flow rate of 0.9 ml/min, while the absorbance of the column effluent at 280 nm was monitored to detect eluting proteins. Under these conditions, fully assembled hexagonal bilayer (HBL) LtEc eluted at 7.5 min, while dissociation products (dodecamers, trimers, and monomers) eluted at later times.
Hemox analysis
Oxygen equilibrium curves for the LtEc samples were measured using a Hemox Analyser (TCS Scientific, New Hope, PA). Prior to analysis, samples were diluted with Hemox buffer and sparged with air to obtain an O2 partial pressure (pO2) of 150 mm Hg. The samples were then sparged with N2 to progressively decrease the pO2 to 2 mm Hg while the percent O2 saturation (Y) in the sample was monitored spectrophotometrically. The pO2 at which the LtEc was 50% saturated with O2 (Y = 0.5) was used to estimate the oxygen affinities (P50) of the samples. The Hill coefficient (n, a measure of subunit cooperativity) [Citation37] was estimated using previously described methods [Citation11,Citation33,Citation38–40].
Statistical analysis
Statistical analysis of the oxidation level (%Fe3+), oxygen affinity (P50), Hill coefficient (n), and recovery datasets was performed in R Studio using either an ANOVA test or the Kruskal-Wallis (K-W) test if the data were found to be non-parametric. Significant differences were defined as any result with a p-value < 0.05. Tukey’s Honestly Significant Different (HSD) test was used to identify significantly different groups. All figures were created using Microsoft Excel. in the Supplemental Information was created with R Studio.
Figure 4. Effects of long-term (6 month) storage at 20 °C or 40 °C on the oxidation (top) and recovery (bottom) of lyophilised LtEc samples. ‘AA’ indicates treatment with ‘Ascorbic Acid’. Representative images of each type of sample following resuspension and centrifugation are also shown between the plots and the corresponding bars. All samples were prepared in triplicate (n = 3). Letters indicate groups that are significantly different as determined via ANOVA or K-W test (p < 0.05).
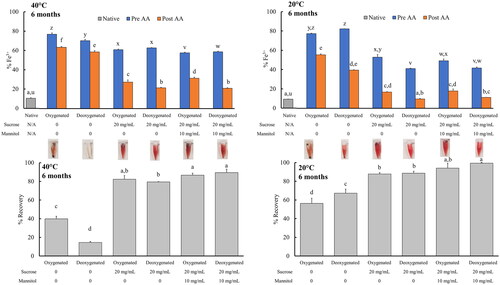
Results
Effects of cryoprotectants
Several cryoprotectants were screened in preliminary experiments to determine their effects on the oxidation and recovery of LtEc samples that were immediately resuspended after lyophilisation. These experiments showed that glycine, DTT, and ascorbic acid did not prevent the oxidation of LtEc during lyophilisation (data not shown), while sucrose significantly limited the oxidation of the lyophilised LtEc (). Specifically, while control samples of LtEc that lacked cryoprotectants were extensively oxidised during lyophilisation (79.7 ± 4.0% Fe3+), the addition of 10 or 20 mg/mL of sucrose significantly decreased the amount of oxidation in those samples (55.4 ± 2.5% Fe3+ and 58.9 ± 2.7%, respectively).
also shows that the addition of mannitol by itself had no effect on the oxidation level of the lyophilised LtEc relative to the control. However, since previous studies have shown that mixtures of sucrose and mannitol may synergistically protect lyophilised proteins [Citation27,Citation28], combinations of 20 mg/mL sucrose with 1, 10, and 20 mg/mL mannitol were also tested. shows that a combination of 20 mg/mL sucrose and 10 mg/mL mannitol provided the most protection against oxidation during lyophilisation, yielding samples with an oxidation level of 26.0 ± 2.6% Fe3+.
Deoxygenation and resuspension with ascorbic acid
Since none of the cryoprotectants were able to completely prevent oxidation of the lyophilised LtEc, a Schlenk line was used to deoxygenate the lyophilised samples and further decrease auto-oxidation. shows that the Schlenk apparatus effectively deoxygenated liquid LtEc samples (as indicated by a shift in the absorbance spectrum from two distinct q-bands at 540 and 576 nm to a broad plateau centred around 560 nm). A fresh sample of liquid LtEc (with no additives) was used to monitor the deoxygenation of the lyophilised LtEc in the Schlenk line, since it was not possible to directly measure the oxygenation of LtEc as a powder (see Supplemental Information Section 4: ). In contrast to the cryoprotectant screening experiments, these deoxygenated LtEc samples were subsequently stored for 2 months at 40 °C and then resuspended to determine the effects of deoxygenation on LtEc oxidation during longer term storage, as shown in .
Figure 5. Analytical size exclusion chromatography (SEC) analysis showing representative chromatograms for LtEc samples before and after lyophilisation, storage for 6 months with sucrose and mannitol, and resuspension with ascorbic acid.
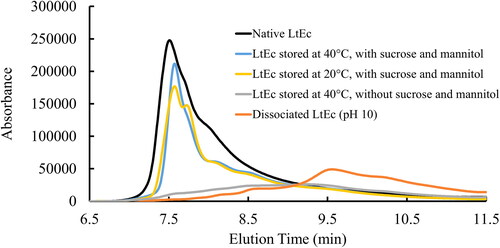
Figure 6. Representative oxygen equilibrium curves measured for native and lyophilised LtEc samples. Oxygen affinity (P50) and cooperativity (n) values estimated from these curves are shown in .
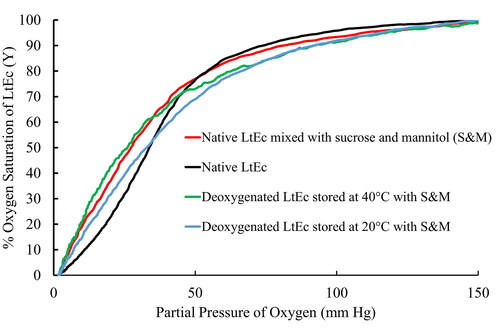
shows that the oxygenated control samples (LtEc samples stored with sucrose and mannitol at 40 °C for 2 months) had a higher oxidation level than the LtEc samples that were immediately resuspended following lyophilisation (%Fe3+ = 40.5 ± 1.5% vs. 26.0 ± 2.6% as shown in ), indicating that more oxidation of the LtEc occurred during the prolonged storage period. However, deoxygenation of the samples prevented some of the auto-oxidation during storage, as shown by the significant decrease in %Fe3+ of the deoxygenated samples following resuspension (30.3 ± 3.9%).
The observation that the deoxygenated samples still oxidised to some extent suggests that the Schlenk line was unable to completely remove all the O2 from the samples. We chose to address this problem of residual oxygen by adding 5.68 mM (1 mg/mL) ascorbic acid to the samples to reduce any LtEc that had oxidised during storage. shows that adding ascorbic acid led to the reduction of a majority of the oxidised LtEc in both the oxygenated (5.0 ± 1.5% Fe3+) and deoxygenated samples (2.7 ± 0.4% Fe3+), with no significant difference in oxidation level between oxygenated and deoxygenated samples.
Long-term storage (6 months) of lyophilized LtEc
Since sucrose, mannitol, and deoxygenation appeared to prevent the oxidation of LtEc to some extent, these measures were used to store lyophilised LtEc samples for up to 6 months at 20 °C or 40 °C to determine if lyophilised LtEc could be stored under conditions that may be encountered in austere environments. The effects of long-term storage on the oxidation and recovery of soluble LtEc are shown in , along with pictures of the resuspended samples (exact values for %Fe3+ and recovery are shown in Supplemental Information Section 5 – Tables S.3–4).
In agreement with previous short-term experiments, deoxygenation of the samples, storage with sucrose, and resuspension with ascorbic acid significantly decreased oxidation of samples stored at 20 °C and 40 °C (9.8 ± 0.4% Fe3+ and 21.5% ± 0.5% Fe3+, respectively) relative to the control samples that were stored without any additives and in the presence of oxygen. Furthermore, the samples that were deoxygenated and stored with sucrose at room temperature showed no significant increase in oxidation relative to freshly purified LtEc samples, while the rest of the samples showed some increase in oxidation during lyophilisation and storage. The addition of mannitol did not have a significant effect on oxidation, but the combination of sucrose and mannitol significantly increased the recovery of soluble LtEc during resuspension (e.g. from 67.2 ± 4.4% in the deoxygenated control sample to 99.5 ± 0.9% in the deoxygenated sucrose and mannitol sample at 20 °C). Deoxygenation did not significantly increase the recovery of any type of sample, but it is interesting to note that deoxygenation of the control samples lacking sucrose or mannitol led to a significant decrease in the recovery of LtEc samples stored at 40 °C. Altogether, these results show that lyophilised LtEc should be deoxygenated, stored with sucrose and mannitol, and resuspended with a buffer containing ascorbic acid to minimise oxidation (%Fe3+ = 21.0 ± 0.5% at 40 °C; %Fe3+ = 10.5 ± 0.2% at 20 °C) and maximise recovery (89.5 ± 3.7% recovery at 40 °C; 99.5 ± 0.9% recovery at 20 °C).
Subunit dissociation
In addition to oxidation and the recovery of soluble LtEc, it is also important to monitor the dissociation of LtEc during storage, since dissociation of the HBL into smaller subunits could lead to extravasation of globins into tissues and accumulation of toxic levels of iron in vivo. To determine if lyophilisation of LtEc induces its dissociation, the samples were analysed with analytical size exclusion chromatography (SEC).
As shown in , freshly purified LtEc samples at pH 7.4 eluted from the SEC column as a single peak at 7.5 min. In contrast, the alkaline dissociation of LtEc at a pH of 10 led to the elution of several smaller peaks/oligomers at later elution times. Control samples stored at 40 °C with no sucrose or mannitol showed a similar elution profile, suggesting that a high degree of HBL dissociation occurred in those samples during the lyophilisation or resuspension steps. Samples that were lyophilised using our optimised formulation and methods exhibited no peaks after an elution time of ∼8 min, suggesting that these LtEc samples were still fully assembled. SEC chromatograms for the other types of samples are shown in Section 6 of the Supplemental Information (Figures S.7–12).
Oxygen affinity and cooperativity
The oxygen affinity (P50) and cooperativity of the resuspended samples were measured using a Hemox Analyser (). First, it is important to note that the oxygen affinity and cooperativity of freshly purified LtEc (P50 = 33.8 ± 1.2 mm Hg, n = 1.9 ± 0.2) decrease significantly when it is mixed with 20 mg/mL sucrose and 10 mg/mL mannitol (P50 = 27.2 ± 0.4 mm Hg, n = 1.6 ± 0.1). Therefore, fresh LtEc mixed with sucrose and mannitol was used as the basis for comparison in these experiments. The oxygen equilibrium curves in show that our optimised lyophilisation method provided samples that were able to bind and release oxygen after long-term storage and resuspension, but some notable differences in cooperativity were observed. Specifically, the deoxygenated LtEc samples showed no significant differences in P50 or the Hill coefficient, n, relative to the freshly purified LtEc samples with sucrose and mannitol added. However, the oxygen affinity and cooperativity of the samples stored with oxygen were significantly lower than the controls and other samples, perhaps because of oxidation or dissociation of the LtEc HBL [Citation41,Citation42].
Discussion
Optimising the formulation of lyophilized LtEc
The combination of sucrose and mannitol protected LtEc from the effects of the lyophilisation process. As previously mentioned, both sugars (e.g. sucrose) and polyols (e.g. mannitol) are thought to create an environment which limits protein mobility [Citation15,Citation18]. Sugars, such as trehalose or sucrose, also create an amorphous boundary that could help to preserve the structural integrity of LtEc [Citation15,Citation18]. It is also thought that polyols protect lyophilised proteins via the water replacement hypothesis [Citation18]. Specifically, polyols increase hydrogen bonding between the hydroxyl groups of the cryoprotectants and the polar groups of the proteins during the drying process, which increases protein stability [Citation15,Citation18,Citation30]. The recovery data support these theories as all the sucrose-mannitol samples had significantly higher recoveries compared to the other conditions that were used, regardless of storage temperature (). These samples had the highest recoveries overall compared to all the other samples.
Overall, the results in and show that deoxygenation of the sample prior to storage, along with resuspension with the antioxidant ascorbic acid, helps to minimise or reverse oxidation of the haem iron, while also helping LtEc maintain its structure. These findings are in line with previous studies and patents that show deoxygenation prevents damage and changes to the oxygen binding properties of haemoglobin proteins during storage since removing oxygen should decrease oxidation [Citation11,Citation32,Citation43,Citation44]. shows that most of the oxygen was removed from our samples, but it might be possible to further prevent oxidation with an improved deoxygenation process.
Sample oxidation
Despite all our efforts, even our best samples were still oxidised to some degree, either during the lyophilisation, storage, or resuspension steps. However, it is important to mention that the freshly purified LtEc samples were already oxidised to some extent prior to lyophilisation (10 ± 0.0% Fe3+) (Supplemental Information Section 2: Table S.2). Therefore, the samples stored at 20 °C for 6 months (%Fe3+ = 11.3 ± 0.2%) were not oxidised any more than the original LtEc samples prior to lyophilisation and there was only a moderate increase in oxidation for the samples stored at 40 °C (%Fe3+ = 21.0 ± 0.5%) relative to the freshly purified LtEc.
In humans, %Fe3+ levels below 90% can induce a condition known as methemoglobinemia [Citation45–49]. This is dangerous condition where the HbA cannot carry enough oxygen and the ferric haem can participate in dangerous oxidative side reactions [Citation45–49]. However, healthy patients are generally not treated so long as the %Fe3+ does not increase above 20% [Citation45,Citation50]. Since the lyophilised LtEc samples discussed here would only be used supplement the existing haemoglobin in the bloodstream, any oxidised LtEc would be diluted by a much larger amount of reduced human haemoglobin, such that transfusion of the LtEc samples stored at 40 °C (%Fe3+ = 21%) would not increase the total %Fe3+ in the patient above the critical threshold of 20% Fe3+.
Biocompatibility of ascorbic acid, sucrose, and mannitol
This study and other previous reports show that ascorbic acid can effectively reduce oxidised LtEc [Citation11,Citation32]. Ascorbic acid, also known as vitamin C, is a water-soluble antioxidant that is essential for human health [Citation51,Citation52]. Oxidised ascorbic acid is known as dehydroascorbic acid (DHA) and can cause some health issues in humans [Citation52]. These issues include an increase in kidney stones and diarrhea [Citation51,Citation52]. Additionally, there is a possibility that DHA in concentrations greater than 2 mM could be disruptive to cell membranes [Citation53]. To put this toxicity into perspective, the LD50 for DHA is 250 mg/kg of body mass, while the LD50 for ascorbic acid is 11,900 mg/kg of body mass [Citation54,Citation55]. In a hypothetical 517 ml bag (approximate volume of 1 unit of whole blood [WB]) of the lyophilised LtEc, there would be approximately 2,585 mg of ascorbic acid [Citation56]. However, the current formulation is 10% of the amount of haemoglobin that would be present in 1 unit of WB (calculated to ∼7.4 mM haem). This value was calculated by averaging the amount of HbA in WB (10–14 g/dL), and then using molecular weight of haemoglobin ∼64,600 Da [Citation57–59]. Correcting for this, the amount of DHA would be approximately 249.5 mg. Therefore, if the ascorbic acid is converted 1:1 with DHA, there could be medical complications from a lyophilised LtEc transfusion, but full animal testing would need to be performed to determine if the DHA generated during reduction of LtEc causes any side effects in vivo. It is also worth mentioning that the aforementioned LD50 values are for healthy individuals and may vary in patients experiencing haemorrhagic shock or other traumas, so it is currently unclear if the additives in the resuspended LtEc will cause side effects in vivo that may limit the maximum safe dose that may be transfused. Therefore, future work should focus on developing additional or alternative antioxidant strategies or minimising the initial oxidation level of the LtEc prior to lyophilisation so that the amount of additives needed to prevent or reverse LtEc oxidation can be decreased.
Similar calculations and precedents for sucrose and mannitol can also be considered to show that our current formulation may be safe to transfuse in vivo [Citation56]. Mannitol is regularly prescribed in concentrations of 250 mg/mL, or 1.5 − 2 g/kg of patient [Citation60]. Additionally, the median lethal dose (LD50) for mannitol injections is 14 g/kg of mass [Citation61]. This means that if the lyophilised LtEc formulation was optimised to match 1 unit of whole blood, the transfusion would be safe in adult patients (assuming ≥100 kg in weight). As a result, mannitol should not negatively affect an adult patient. Similarly, sucrose also has an LD50 of 14 g/kg of mass [Citation62].
Structure and function of lyophilized LtEc
and show that the lyophilised samples are still able to bind and release oxygen after resuspension in vitro, with only minor changes in P50 and cooperativity relative to the freshly purified controls. Therefore, it appears that the lyophilised LtEc should be able to transport oxygen in vivo as well. It is interesting to note that sucrose and mannitol significantly decreased the P50 (i.e. increased the oxygen affinity) of both the freshly purified LtEc and the lyophilised LtEc stored at 40 °C for 6 months. The nature of this phenomenon is unknown, but it is important to note that the decreased P50 is still much higher than several other HBOCs that have been tested in vivo [Citation14,Citation40,Citation63]. It is also likely that the effects of sucrose and mannitol on the LtEc will be inconsequential in vivo, since those smaller molecules will be rapidly metabolised or cleared from the bloodstream.
Conclusion
Our lyophilisation method represents an improvement over the techniques previously reported by Zal and Palmer [Citation26,Citation31,Citation32], since we demonstrated that lyophilised LtEc can be stored at up to 40 °C for up to 6 months with minimal loss in structure or function. However, further work will be required to determine the safety and efficacy of lyophilised LtEc for use in transfusion.
Authors’ contributions
Sean Dowd (analysis and interpretation of the data, drafting of the paper), Catherine Sharo (analysis and interpretation of the data), Osheiza Abdulmalik (analysis and interpretation of the data, revised critically for intellectual content, final approval of the version to be published), and Jacob Elmer (conception and design, analysis and interpretation of the data, drafting of the paper, revising critically for intellectual content, final approval of the version to be published). All authors agree to be accountable for all aspects of the work.
Acknowledgements
The authors wish to acknowledge the contributions of Dr. Charles Coe for help with the Schlenk line studies, Dr. Noelle Comolli for use of the lyophilizer and HPLC, and Dr. Nathaniel Myers for help with the lyophilizer and HPLC studies. This work also received funding for open access publication costs from the Villanova University’s Falvey Memorial Library Scholarship Open Access Reserve (SOAR) Fund.
Disclosure statement
In accordance with Taylor & Francis policy and my ethical obligation as a researcher, I, Jacob Elmer, am reporting that I am a consultant to a company (Bexorg, Inc. in New Haven, CT, U.S.A.) that may be affected by the research reported in the enclosed paper. I have disclosed those interests fully to Taylor & Francis, and I have in place an approved plan filed with Villanova University for managing any potential conflicts arising from my paid consulting activities.
Data availability statement
The data that support the findings of this study are available from the corresponding author, JE, upon reasonable request.
Additional information
Funding
References
- Cannon JW. Hemorrhagic shock. N Engl J Med. 2018;378(4):370–379. doi:10.1056/NEJMra1705649.
- Burgess FW, Sborov MJ, Calcagni DR. Hemorrhage, shock, and fluid resuscitation. In: Zajtchuk R, Grande CM, editors. Anesthesia and perioperative care of the combat casualty. 1st ed. Washington, D.C.: Office of the Surgen General at TMM Publications Borden Institute; 1995. p. 81–100.
- Gurney JM, Le TD, Becker TE, et al. Epidemiology if hemorrhagic shock. In: Pascual JL, Cannon JW, editors. Hemorrhagic shock. 1st ed. New York, NY: Nova Science Publishers, Inc.; 2017. p. 1–17.
- Kim-Shapiro DB, Lee J, Gladwin MT. Storage lesion: role of red blood cell breakdown. Transfusion (Paris). 2011;51(4):844–851. doi:10.1111/j.1537-2995.2011.03100.x.
- Malsby RF, Quesada J, Powell-Dunford N, et al. Prehospital blood product transfusion by U.S. Army MEDEVAC during combat operations in Afghanistan: a process improvement initiative. Mil Med. 2013;178(7):785–791. doi:10.7205/MILMED-D-13-00047.
- Spinella PC, Dunne J, Beilman GJ, et al. Constant challenges and evolution of US military transfusion medicine and blood operations in combat. Transfusion (Paris). 2012;52(5):1146–1153. doi:10.1111/j.1537-2995.2012.03594.x.
- Pusateri AE, Given MB, Schreiber MA, et al. Dried plasma: state of the science and recent developments. Transfusion (Paris). 2016;56(Suppl 2):S128–S139. doi:10.1111/trf.13580.
- Bowersox JC, Hess JR. Trauma and military applications of blood substitutes. Artif Cells Blood Substit Immobil Biotechnol. 1994;22(2):145–157. doi:10.3109/10731199409117410.
- Matsuhira T, Sakai H. Artificial oxygen carriers, from nanometer- to micrometer-sized particles, made of hemoglobin composites substituting for red blood cells. Particuology. 2021;64:43–55. doi:10.1016/j.partic.2021.08.010.
- Elmer J, Palmer AF. Biophysical properties of Lumbricus terrestris erythrocruorin and its potential use as a red blood cell substitute. J Funct Biomater. 2012;3(1):49–60. doi:10.3390/jfb3010049.
- Muzzelo C, Neely C, Shah P, et al. Prolonging the shelf life of Lumbricus terrestris erythrocruorin for use as a novel blood substitute. Artif Cells Nanomed Biotechnol. 2018;46(1):39–46. doi:10.1080/21691401.2017.1290645.
- Elmer J, Harris D, Palmer AF. Purification of hemoglobin from red blood cells using tangential flow filtration and immobilized metal ion affinity chromatography. J Chromatogr B Analyt Technol Biomed Life Sci. 2011;879(2):131–138. doi:10.1016/j.jchromb.2010.11.021.
- Elmer J, Palmer AF, Cabrales P. Oxygen delivery during extreme anemia with ultra-pure earthworm hemoglobin. Life Sci. 2012;91(17–18):852–859. doi:10.1016/j.lfs.2012.08.036.
- Elmer J, Zorc K, Rameez S, et al. Hypervolemic infusion of Lumbricus terrestris erythrocruorin purified by tangential-flow filtration. Transfusion (Paris). 2012;52(8):1729–1740. doi:10.1111/j.1537-2995.2011.03523.x.
- Fernandez-Moure J, Maisha N, Lavik EB, et al. The chemistry of lyophilized blood products. Bioconjug Chem. 2018;29(7):2150–2160. doi:10.1021/acs.bioconjchem.8b00271.
- Maher P, Utarnachitt R, Louzon MJ, et al. Logistical concerns for prehospital blood product use by air medical services. Air Med J. 2017;36(5):263–267. doi:10.1016/j.amj.2017.05.003.
- Goodrich RP, Sowemimo-Coker SO, Zerez CR, et al. Preservation of metabolic activity in lyophilized human erythrocytes. Proc Natl Acad Sci U S A. 1992;89(3):967–971. doi:10.1073/pnas.89.3.967.
- Butreddy A, Janga KY, Ajjarapu S, et al. Instability of therapeutic proteins—an overview of stresses, stabilization mechanisms and analytical techniques involved in lyophilized proteins. Int J Biol Macromol. 2021;167:309–325. doi:10.1016/j.ijbiomac.2020.11.188.
- Franks F. Freeze-drying of bioproducts: putting principles into practice. Eur J Pharm Biopharm. 1998;45(3):221–229. doi:10.1016/s0939-6411(98)00004-6.
- Sailliol A, Martinaud C, Cap AP, et al. The evolving role of lyophilized plasma in remote damage control resuscitation in the French armed forces health service. Transfusion (Paris). 2013;53(Suppl 1):65S–71S. doi:10.1111/trf.12038.
- Iijima T. Thermal analysis of cryoprotective solutions for red blood cells. Cryobiology. 1998;36(3):165–173. doi:10.1006/cryo.1998.2075.
- Chao H, Davies PL, Carpenter JF. Effects of antifreeze proteins on red blood cell survival during cryopreservation. J Exp Biol. 1996;199(Pt 9):2071–2076. doi:10.1242/jeb.199.9.2071.
- Mazur P. Freezing of living cells: mechanisms and implications. Am J Physiol. 1984;247(3 Pt 1):C125–C142. doi:10.1152/ajpcell.1984.247.3.C125.
- Meryman HT. Freezing injury and its prevention in living cells. Annu Rev Biophys Bioeng. 1974;3(0):341–363. doi:10.1146/annurev.bb.03.060174.002013.
- Lovelock JE. The mechanism of the protective action of glycerol against haemolysis by freezing and thawing. Biochim Biophys Acta. 1953;11(1):28–36. doi:10.1016/0006-3002(53)90005-5.
- Zal F, Rousselot M. Annelid haemoglobin lyophilisation process. USA: US Patent & Trademark Office; 2020.
- Chintala R, Bhambhani A. HPV vaccine formulations comprising aluminum adjuvant and methods of producing same [internet]. US Patent App. 14/126,992. USA: US Patent & Trademark Office; 2020. Available from: http://www.google.com/patents/US20140127260.
- McAleer WJ, Markus HZ. Lyophilization process for live viral compositions [internet]. USA: US Patent & Trademark Office; 1981. Available from: https://patents.google.com/patent/US4273762A/en.
- Akers MJ, Vasudevan V, Stickelmeyer M. Formulation development of protein dosage forms. Pharm Biotechnol. 2002;14:47–127. doi:10.1007/978-1-4615-0549-5_2.
- Patapoff TW, Overcashier DE. The importance of freezing on lyophilization cycle development. BioPharm. 2002;15:16–21 + 72.
- Zal F, Rousselot M. Annelid haemoglobin lyophilisation process. European Union: European Patent Office; 2018.
- Savla C, Palmer AF. Lyophilized annelid mega-hemoglobin retains its’ quaternary structure and oxygen equilibrium properties after room temperature storage for over 6 months. PLoS One. 2022;17(2):e0263996. doi:10.1371/journal.pone.0263996.
- Zimmerman D, Dienes J, Abdulmalik O, et al. Purification of diverse hemoglobins by metal salt precipitation. Protein Expr Purif. 2015;125:74–82. doi:10.1016/j.pep.2015.09.006.
- Timm B, Elmer J. Enhancing the stability of Lumbricus terrestris erythrocruorin (LtEc) for use as a blood substitute. International Symposium for Blood Substitutes 2017 Review; 2019. p. 3093.
- Schwegman JJ, Hardwick LM, Akers MJ. Practical formulation and process development of Freeze-Dried products. Pharm Dev Technol. 2005;10(2):151–173. doi:10.1081/pdt-56308.
- Heller MC, Carpenter JF, Randolph TW. Protein formulation and lyophilization cycle design: prevention of damage due to freeze-concentration induced phase separation. Biotechnol Bioeng. 1999;63(2):166–174. doi:10.1002/(SICI)1097-0290(19990420)63:2<166::AID-BIT5>3.0.CO;2-H.
- Koike Y, Tomono Y, Tanaka H, et al. The possible effects of the aggregation of the molecules of hemoglobin on its dissociation curves. J Physiol. 1910;40:iv–vii. Available from: https://cir.nii.ac.jp/crid/1571980074887256960.
- Rajesh A, Zimmerman D, Spivack K, et al. Glutaraldehyde cross-linking increases the stability of Lumbricus terrestris erythrocruorin. Biotechnol Prog. 2018;34(2):521–528. doi:10.1002/btpr.2593.
- Spivack K, Tucker M, Zimmerman D, et al. Increasing the stability of lumbricus terrestris erythrocruorin via poly(acrylic acid) conjugation. Artif Cells Nanomed Biotechnol. 2018;46(sup2):1137–1144. doi:10.1080/21691401.2018.1480491.
- Zimmerman D, DiIusto M, Dienes J, et al. Direct comparison of oligochaete erythrocruorins as potential blood substitutes. Bioeng Transl Med. 2017;2(2):212–221. doi:10.1002/btm2.10067.
- Edelstein SJ. Cooperative interactions of hemoglobin. Annu Rev Biochem. 1975;44(1):209–232. doi:10.1146/annurev.bi.44.070175.001233.
- Carver TE, Brantley RE, Singleton EW, et al. A novel site-directed mutant of myoglobin with an unusually high O2 affinity and low autooxidation rate. J Biol Chem. 1992;267(20):14443–14450. doi:10.1016/S0021-9258(19)49732-9.
- Bitensky MW, Yoshida T. Blood storage device and method for oxygen removal. USA: USPTO; 2000.
- Zolla L, D’Alessandro A. An efficient apparatus for rapid deoxygenation of erythrocyte concentrates for alternative banking strategies. J Blood Transfus. 2013;2013:896537–896538. doi:10.1155/2013/896537.
- Panjwani D, Li MLJ. Methemoglobinemi. In: Mattu A, Chanmugam AS, Swadron SP, et al., editors. Avoiding common errors in the emergency department. 2nd ed. Waltham, MA: Wolters Kluwer; 2017. p. 827–829.
- Wright RO, Lewander WJ, Woolf AD. Methemoglobinemia: etiology, pharmacology, and clinical management. Ann Emerg Med. 1999;34(5):646–656. doi:10.1016/s0196-0644(99)70167-8.
- Costello JF, Plass C. Methylation matters. J Med Genet. 2001;38(5):285–303. Available from: www.jmedgenet.com. doi:10.1136/jmg.38.5.285.
- Tomoda A, Takeshita M, Yoneyama Y. Characterization of intermediate hemoglobin produced during methemoglobin reduction by ascorbic acid; 1978.
- Dela Cruz M, Glick J, Merker SH, et al. Survival after severe methemoglobinemia secondary to sodium nitrate ingestion. Toxicol Commun. 2018;2(1):21–23. doi:10.1080/24734306.2018.1467532.
- Ludlow JT, Wilkerson RG, Nappe TM. Methemoglobinemia. StatPearls. Treasure Island, FL: StatPearls Publishing LLC; 2023.
- Institute of Medicine F and NB. Vitamin C. In: Institute of Medicine F and NB. editor. Dietary reference intakes for vitamin C, vitamin E, selenium, and carotenoids [internet]. Washington, DC: National Academy Press; 2000. p. 95–186. Available from: https://www.ebsco.com/terms-of-use.
- Sulaiman S, Heis Z, Jahangir A. Drug-Nutrient Interactions in the Elderly. In: Malavolta M, Mocchegiani E, eds. Molecular Basis of Nutrition and Aging. 1st ed. San Diego, CA: Academic Press; 2016:73–107.
- Bianchi J, Rose RC. Dehydroascorbic acid and cell membranes: possible disruptive effects. Toxicology. 1986;40(1):75–82. doi:10.1016/0300-483x(86)90047-8.
- ThermoFisher Scientific. Ascorbic Acid Safety Data Sheet. 2021.
- Cayman Chemicals. Dehydroascorbic Acid Safety Data Sheet. 2019.
- Woodson LC, Sherwood ER, Aarsland A, et al. Anesthesia for burned patients. In: Herndon DN, editor. Total burn care: expert consult. 4th ed. Philadelphia, PA: Elsevier, Inc.; 2012. p. 194.
- Manjula BN, Acharya SA. Hemoglobin disorders molecular methods and protocols. In: Nagel RL, editor. Hemoglobin disorders: molecular methods and protocols. Totowa, NJ: Humana Press; 2003. p. 31–47.
- Nguyen TQ. Interactions of human hemoglobin with high‐molecular‐weight dextran sulfate and diethylaminoethyl dextran. Makromol Chem. 1986;187(11):2567–2578. doi:10.1002/macp.1986.021871106.
- Ardin S, Störmer M, Radojska S, et al. Comparison of three noninvasive methods for hemoglobin screening of blood donors. Transfusion (Paris). 2015;55(2):379–387. doi:10.1111/trf.12819.
- Hospira. Mannitol injection, for intravenous use [Internet]. 2020. Available from: www.fda.gov/medwatch.
- Cayman Chemical. Mannitol Safety Data Sheet. 2022.
- VWR Canada. Sucrose Safety Data Sheet. 2009.
- Song BK, Nugent WH, Moon-Massat PF, et al. Effects of a hemoglobin-based oxygen carrier (HBOC-201) and derivatives with altered oxygen affinity and viscosity on systemic and microcirculatory variables in a top-load rat model. Microvasc Res. 2014;95:124–130. doi:10.1016/j.mvr.2014.07.005.