Abstract
The global epidemic of metabolic diseases has led to the emergence of metabolic dysfunction-associated steatotic liver disease (MASLD) and metabolic dysfunction-associated steatohepatitis (MASH), which pose a significant threat to human health. Despite recent advances in research on the pathogenesis and treatment of MASLD/MASH, there is still a lack of more effective and targeted therapies. Extracellular vesicles (EVs) discovered in a wide range of tissues and body fluids encapsulate different activated biomolecules and mediate intercellular communication. Recent studies have shown that EVs derived from the liver and adipose tissue (AT) play vital roles in MASLD/MASH pathogenesis and therapeutics, depending on their sources and intervention types. Besides, adipose-derived stem cell (ADSC)-derived EVs appear to be more effective in mitigating MASLD/MASH. This review presents an overview of the definition, extraction strategies, and characterisation of EVs, with a particular focus on the biogenesis and release of exosomes. It also reviews the effects and potential molecular mechanisms of liver- and AT-derived EVs on MASLD/MASH, and emphasises the contribution and clinical therapeutic potential of ADSC-derived EVs. Furthermore, the future perspective of EV therapy in a clinical setting is discussed.
1. Background
Metabolic dysfunction-associated steatotic liver disease (MASLD) is a liver disease characterised by hepatic steatosis and at least one cardiometabolic risk factor, without any other identifiable cause [Citation1], emerging as a considerable menace to public health worldwide. According to recent studies, the global prevalence of MASLD was about 30% from 1990 to 2019, and this number continues to increase, particularly in Latin America, the Middle East and North Africa, South Asia, and Southeast Asia, where rates are higher [Citation2]. In China, the national prevalence of MASLD was 29.2% from 2008 to 2018 [Citation3]. A predictive model estimated that the MASLD prevalence in China could surge by 29.1% from 2016 to 2030 [Citation4]. If the liver exhibits abnormal changes, ranging from hepatocyte injury, ballooning, inflammation, to varying degrees of fibrosis induced by hepatic stellate cell (HSC) activation, it indicates that MASLD has progressed to metabolic dysfunction-associated steatohepatitis (MASH). MASH can eventually develop into cirrhosis, hepatic failure, and hepatocellular carcinoma [Citation5, Citation6]. The evolution of MASLD to MASH has been proven to be driven by the innate immune system and lipotoxicity due to dysregulated lipid metabolism, resulting in organellar dysfunction, inflammation, abnormal activation of intracellular signalling, hepatocellular injury, and cell death [Citation6, Citation7]. Aside from dyslipidemia, MASLD/MASH is often complicated by other metabolic comorbidities, including obesity, type 2 diabetes mellitus, and hypertension [Citation8, Citation9]. Therefore, MASLD/MASH is often regarded as the hepatic manifestation of the metabolic syndrome. Despite some advances in research, the pathogenesis of MASLD/MASH has not been fully understood. Thanks to the in-depth research on extracellular vesicles (EVs), new concepts on the pathogenesis and treatment of MASLD/MASH have gradually emerged.
Accumulating evidence has shown that EVs secreted by various cells play a role in physiological or pathological processes relying on the paracrine pathway [Citation10]. EVs are nanoscale and lipid-membrane vesicles shed by almost all cell types and widely distributed in the human body, transporting various molecular cargoes (such as lipids, proteins, and nucleic acids), mediating intercellular communication, and deeply getting into the pathophysiological processes of recipient cells. EVs are heterogeneous in terms of content, size, quantity, and surface markers, depending on the origin of cells and the protein complex in membrane trafficking. These heterogeneities determine the fate and function of EVs, showing diagnostic and therapeutic potential for pathophysiological conditions [Citation11, Citation12]. Moreover, EVs have been shown to remarkably take part in angiogenesis, regulation of proliferation and apoptosis, cell migration, cellular differentiation, and immunomodulation [Citation13, Citation14]. Importantly, EVs could exert the same therapeutic capacity as their source cells [Citation10, Citation15–17]. Without the drawbacks of cell therapy, they exhibit good biocompatibility, low toxicity, stability in biological fluids, and convenient manufacturing, storage, and delivery [Citation10, Citation14, Citation15]. And their excellent prospects for industrialisation and commercialisation could not be overlooked. These findings make EVs an ideal delivery vector for drug therapy and a promising alternative for cell treatment. With their phospholipid bilayer membrane structure, EVs are classified into different subtypes such as microvesicles, apoptotic bodies, and exosomes according to various characterisations [Citation10, Citation18–21]. Exosomes are the primary focus in this review due to the thorough research on their functions and underlying mechanisms. In MASLD/MASH, an increasing number of studies have shown that liver- and adipose tissue (AT)-derived exosomes, a type of small EV, may play an important role [Citation6, Citation22, Citation23]. MicroRNAs (miRNAs) are non-coding RNAs that are evolutionarily conserved across vertebrates [Citation24–26]. And they could regulate gene expression at the transcriptional level. EV miRNAs could also be considered as indicators of pathophysiological conditions and are deeply involved in the biological functions of resident cells [Citation27–29].
AT is widely recognised as an endocrine organ in the human body, involved in the regulation of various pathophysiological processes. It could also release abundant EVs into the circulation to act on distant cells or tissues. The EV crosstalk between the liver and AT is believed to be involved in the pathophysiological process of the liver in MASLD/MASH [Citation30–32]. Although adipocytes dominate AT, adipose-derived stem cells (ADSCs) are of great interest due to their unlimited proliferation and differentiation ability. Studies have found that ADSC-assisted cell therapy has great efficacy and broad application prospects in MASLD/MASH [Citation33, Citation34]. The corresponding EV treatment could achieve similar effects or even better than the former [Citation10, Citation15], suggesting the possibility of ADSC-derived EV therapy for MASLD/MASH.
This review provides an outline of how EVs are defined, extracted, and characterised, with a particular focus on exosome biogenesis and release. The roles and underlying mechanisms of liver- and AT-derived EVs on MASLD/MASH are highlighted. Moreover, the review discusses the contribution and potential clinical application of ADSC-derived EVs, as well as the future perspective of EV therapy.
2. EVs
2.1. Subtypes of EVs
The EV subpopulations have similarities and differences in biophysical properties, biochemical properties, biogenesis, and cellular release form (). Microvesicles (100–1000 nm) enriched in phosphatidylserine and cholesterol outward bud directly from the plasma membrane [Citation20, Citation21, Citation35]. Apoptotic bodies, ranging from 50 nm to 5000 nm, are formed when the cell undergoes apoptosis [Citation18, Citation20, Citation21]. The exosomes are the best described. The definition of exosomes and the dramatic expansion of related research began in the 1980s [Citation36]. Exosomes, with a diameter of 50–150 nm [Citation17, Citation18, Citation35] and a density of 1.13–1.19 g/mL in the sucrose gradient [Citation37], have a typical cup-shaped morphology and encapsulate nucleic acids, proteins, lipids, and metabolites. They are widely distributed in various organs and biofluids of the human body, such as liver, AT, circulating blood, breast milk, and urine. Besides, some research has referred to exosomes as EVs, and both have been discussed in this article.
Table 1. Subtypes of EVs.
2.2. Biogenesis and release of exosomes
Different subpopulations of EVs have different methods of biogenesis and secretion, which is a complex multistep process with the help of organelles, such as Golgi, endoplasmic reticulum (ER), and lysosome [Citation13, Citation19, Citation38]. Exosome formation begins with early-sorting endosomes, which are formed by the invagination of the cell membrane. The endosomes gradually mature into late-sorting endosomes. The latter undergo inward invagination of the endosomal limiting membrane to generate intraluminal vesicles (ILVs). Subsequently, the multivesicular bodies (MVBs) form until late-sorting endosomes envelop multiple ILVs. Finally, some MVBs are sent to lysosomes for cargo degradation, while others fuse with the plasma membrane and release ILVs as exosomes into the extracellular environment (). The entire process is inseparable from the participation of molecules (such as lipids and proteins) on the EV membrane, suggesting a strong link between EVs and lipids.
Figure 1. Biogenesis and secretion of exosomes.
In exosome biogenesis, the Golgi apparatus and ER participate in the formation of early-sorting endosomes and the sorting of the protein cargo. In the secretory pathway, MVBs fuse with the cell membrane to release the exosomes. Alternatively, certain MVBs undergo fusion with lysosomes to release ILVs into the lysosomal lumen for cargo degradation. This figure was created by the authors of this article.
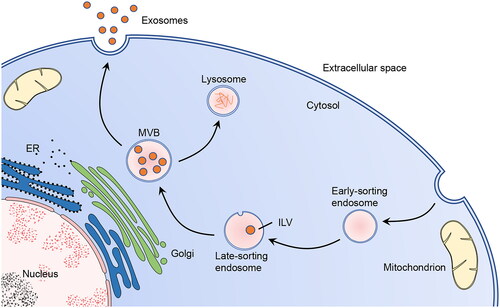
2.3. Isolation of EVs
The quality of isolated EVs profoundly influences the subsequent analysis and application. However, obtaining EVs with excellent yield, morphological integrity, and absolute purity is challenging. First, EV subpopulations are heterogeneous in several ways and have considerable overlap in size and other phenotypes [Citation35, Citation39]. Second, many non-vesicular macromolecules, similar to EVs, are difficult to ignore [Citation35, Citation39]. Therefore, the most appropriate method for EV extraction should be carefully selected following the experimental purpose, sample property, total amount, cost, equipment condition, and downstream application.
Several isolation methods could be used for EVs based on different mechanisms, such as ultracentrifugation and size-exclusion chromatography (). Previous studies have shown that ultracentrifugation is the most widely used technique [Citation40–42]. It is considered a recommendable choice for reserving high-quality and high-concentration EVs in the enrichment process [Citation43]. However, it may disrupt EV integrity and induce aggregation [Citation44, Citation45], and the extracted EVs have low specificity, which poses a great challenge for follow-up experiments. Size-exclusion chromatography, as the second most used technique, collects uniform particles in segmental separation by size, shape, and molecular weight [Citation21, Citation42]. It has a great balance in the purity and yield of EV isolation [Citation46]. And it could increase the purity of EVs better than ultracentrifugation, especially for samples with complex components, such as serum, plasma, or urine [Citation21, Citation46–49].
Table 2. Characterisation of EV isolation methods.
There is no “golden standard” technique to obtain EVs with high recovery, integrity, and specificity. In a global survey conducted in 2015, nearly 60% of the respondents chose the combined extraction method [Citation40]. The combined approach is nothing more than a hope for researchers to obtain ideal EVs. Recently, diversified separation methods, such as tangential flow, microfluidics, and field-flow fractionation, have been gradually developed with favourable results [Citation32, Citation42, Citation50, Citation51]. However, further validation experiments are required before large-scale application.
2.4. Quantification and characterisation of EVs
Accurately controlling the input of EVs in functional experiments is crucial for obtaining reliable results, highlighting the importance of quantification and normalisation significant. However, precisely determining the amount of EVs is challenging. In addition to clarifying the mass or volume of the EVs’ source, quantifying the particle number and components of EVs could be an alternative option for EV quantification.
Particle number can be measured using various technologies, such as nanoparticle tracking analysis (NTA) and flow cytometry. NTA utilises light scattering and Brownian motion to detect particle concentration in liquid suspension and size distribution with diameters ranging from tens to thousands of nanometres [Citation52, Citation53]. However, NTA is sensitive to non-EV contamination and tends to overestimate vesicles [Citation10, Citation54, Citation55]. Besides, standard flow cytometry is limited by the optical diffraction limit (∼200 nm), making it difficult to detect and screen smaller EV markers at the single vesicle level [Citation56]. Therefore, it is better suited for lager EVs. For smaller EVs, high-resolution flow cytometry should be employed [Citation41]. Quantifying the cargo of EVs is desirable due to the presence of numerous biomolecules. Measuring the protein concentration is one of the preferred methods, such as using the BCA or Bradford method [Citation42]. These methods are relatively simple and do not require special detection equipment. However, this indirect measurement may lack accuracy.
There are likely several other types of impurities after EV extraction, as their subtypes share many physical properties. Therefore, it is preferable to perform various characterisation measurements before performing functional experiments.
Individual EV characterisation should include high-resolution images and other results reflecting biophysical properties, such as particle size. Electron microscopy, specially transmission electron microscopy (TEM), could directly observe the typical lipid bilayer and unique morphology of EVs at the subcellular level (). Particle size could be analysed using electron microscopy, NTA, and flow cytometry. While the electron microscopy can detect the smallest EVs, NTA provides a more comprehensive view and can detect relatively smaller vesicles [Citation57]. To characterise EVs, it is necessary to identify biomarkers. Although there is no consensus on specific markers for EV subtypes, five categories of biomarkers based on protein composition have been recommended, including transmembrane or GPI-anchored proteins, cytosolic proteins, and others [Citation41]. To ensure accurate identification of EV subtypes, it is important to comprehensively consider biomarker selection. Western blot and flow cytometry are commonly used to validate these biomarkers, but they may not be able to distinguish co-isolated non-EV-derived proteins. To address this issue, certain EV dyes and reagents may be useful [Citation41].
Figure 2. Representative result of exosomes detected by TEM.
Exosomes were obtained through the differential ultracentrifugation technique. The ADSCs were obtained from the adipose tissue of patients who underwent liposuction surgery at the Plastic Surgery Hospital, Chinese Academy of Medical Sciences and Peking Union Medical College. The related study was conducted in accordance with the Declaration of Helsinki, and the protocol was approved by the Medical Ethics Committee of Plastic Surgery Hospital, Chinese Academy of Medical Sciences and Peking Union Medical College (No. 2020 (129)). The culture medium from human ADSCs’ third to fifth passages was collected and then centrifuged at 300 g and 2000 g for 10 min and 10,000 g for 30 min at 4 °C to remove the deposit. The supernatant was ultracentrifuged (himac, Japan) at 100,000 g for 70 min at 4 °C. The sediment was washed again with PBS and resuspended to obtain exosomes. The exosomes were observed by TEM (JEOL, Japan) after negatively staining. They exhibited a typical cup-shaped morphology and were approximately 100 nm in size. Scale bar = 200 nm. This figure was taken by the author of this article.
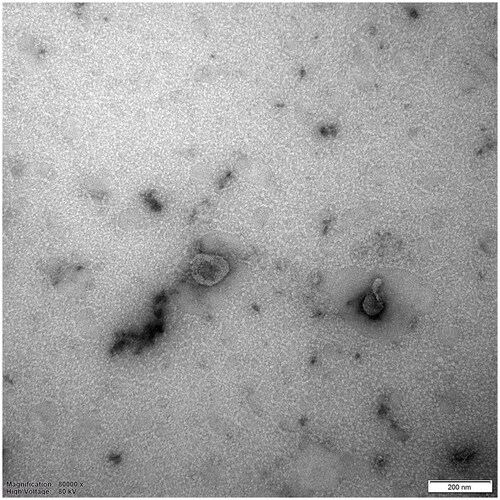
Despite the increasing number of measurement technologies available, precisely quantifying and characterising EVs using a single technique remains a challenge. Therefore, it is desirable to use a comprehensive approach for identifying them in related studies.
3. Liver-derived EVs in MASLD/MASH
3.1. Interaction between EVs and lipids
Lipids are a kind of critical cargoes and components in EVs, and these particles are rich in cholesterol, sphingomyelin, phosphatidylserine, phosphatidylcholine, and ceramide [Citation14, Citation58]. There is a strong correlation between lipids and EV concentration. Plasma levels of small EVs are positively correlated with EV sphingolipids in patients with MASH [Citation59]. And the similar correlation was found between EV concentrations and plasma cholesterol levels [Citation60].
Circulating EV levels are often influenced by lipid metabolism, as lipids are involved in EV membrane components, biogenesis, and cellular release [Citation61]. Several lipid components mediate EV biogenesis and release. The sphingolipid ceramide could decide the budding of EVs into MVBs, except for the endosomal sorting complex required for the transport machinery [Citation58]. Cholesterol could increase exosome release from hepatocytes by inducing lysosomal dysfunction [Citation62]. And cellular cholesterol could modulate the astrocyte-derived exosome release through the phosphoinositide 3-kinase/Akt pathway [Citation63]. Meanwhile, recent studies demonstrated that EVs might be engaged in lipid metabolism [Citation64, Citation65]. Exosomes was reported to induce lipid droplet formation in macrophage and non-macrophage cells [Citation66]. Therefore, EVs may carry some weight in diagnosing and treating lipid metabolic abnormalities and diseases.
3.2. Secretion of EVs in MASLD/MASH
In MASLD/MASH, the increase in circulating EVs could be observed consistently throughout the disease [Citation67–70]. And the liver appears to be an important source of circulating EVs [Citation67]. Levels of circulating total and hepatocyte-derived EVs are dynamic and reflect the clinical characterisation and severity of the disease [Citation59, Citation67, Citation71, Citation72]. In addition to hepatocytes, the source cells cover invariant natural killer T (iNKT) cells, macrophages/monocytes (CD14+ cells) and neutrophils that mediate the disease pathogenesis, as well as leukocytes [Citation69, Citation73, Citation74]. The levels of EVs secreted by iNKT and CD14+ cells may also be the indicators of MASH severity [Citation73]. Besides, the plasma concentration of leukocyte-derived CD16+ EVs was inversely associated with the severity of hepatic fibrosis [Citation74]. In a sense, the levels of circulating or specific cell-derived EVs may serve as an indicator of the severity of MASLD/MASH.
The biogenesis and release process of EV involves various mechanisms in MASLD/MASH. In MASLD, the release of hepatocyte-derived exosomes could be modulated by DNA damage-regulated autophagy modulator through affecting the lysosomal bioactivity [Citation70]. And lipids may trigger hepatocyte-derived EV release by activating death receptor 5 (DR5) in a ligand-independent manner, which could be suppressed by inactivating mediators of the DR5 pathway or by inhibiting rho-associated coiled-coil-containing protein kinase 1 inhibition [Citation75]. Since MASLD/MASH is characterised by abnormal lipid metabolism, EV secretion was also evaluated under this pathological condition. When lipid overload occurs, the liver responds immediately [Citation64]. Through the Rab GTPase prenylation-dependent manner (Rab27A geranylgeranylation), elevated geranylgeranyl diphosphate synthase (GGPPS) increases the hepatocyte-derived EV secretion [Citation64]. And Rab27A was uncovered to function in MVBs docking to the plasma membrane, which belongs to the Rab GTPases and is regulated by protein prenylation [Citation64, Citation76]. To date, more in-depth studies are needed to better understand the molecular mechanisms underlying EV biogenesis and release in MASLD/MASH.
3.3. Role of hepatocyte-derived EVs and underlying mechanism in MASLD/MASH
In MASLD/MASH, the mechanism linking lipotoxicity to hepatocellular injury and inflammation has not been fully understood. However, with the deepening of related studies, it has been confirmed that EVs are incorporated in the regulation of the liver microenvironment and participate in the progression of the disease. Hepatocyte-derived EVs have been shown to play an imperative role in causing lipotoxicity, especially leading to macrophage activation and inflammation.
Many pathways exist for EVs to participate in regulatory activities, and the ceramide-dependent pathway has been the most widely reported. Lipotoxic EVs secreted by palmitate-treated hepatocytes were enriched in ceramide and sphingosine-1-phosphate (S1P), which could activate macrophage chemotaxis and recruit them to the liver [Citation68, Citation77]. Under lipotoxic conditions, the increase of ceramide-enriched EV released from hepatocytes probably rely on the endoplasmic reticulum to nucleus signalling 1 (namely IRE1α)/X-box binding protein 1 (XBP1)/serine palmitoyltransferase (SPT) pathway [Citation68, Citation78] (). Furthermore, ceramide transporter 1 could regulate ceramide distribution in exosomes and cells, and mediate the palmitate-stimulated EV release [Citation79]. Known as a pro-inflammatory lipid and ceramide metabolite, S1P is enriched in lipotoxic EVs via sphingosine kinases 1 and 2 and probably induces macrophage infiltration via S1P1 receptor [Citation77]. Using S1P antagonist could improve the symptoms of animal models of MASH [Citation80].
Figure 3. Elevated secretion of hepatocyte-origin ceramide-enriched EVs in lipotoxic circumstances through IRE1α/XBP1/SPT pathway.
In the lipotoxic environment, hepatocytes experience ER stress and activation of IRE1α. The activated IRE1α cleaves XBP1u mRNA, converting it to XBP1s mRNA, which then translates into an active transcription factor. Subsequently, XBP1s binds to SPT, its target gene, in the nucleus and facilitates the downstream transcription and translation. Since SPT is a crucial rate-limiting enzyme of de novo ceramide biosynthesis, and ceramide can impact EV release, the concentration of ceramide-rich EVs increased. These EVs are then released into the circulation, leading to macrophage activation and recruitment to the liver. This figure was created using the results from Kakazu et al. (2016) and Dasgupta et al. (2020).
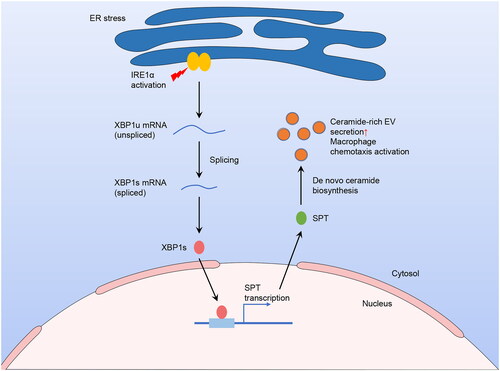
C-X-C motif chemokine ligand (CXCL) and its receptors are likewise related to hepatocyte-derived EVs in MASLD/MASH. Increased hepatic expression and serum levels of CXCL10 are observed in MASH patients, associated with disease severity [Citation81, Citation82]. CXCL10-bearing EVs secreted by lipotoxic hepatocytes could induce macrophage chemotaxis and macrophage-driven inflammation [Citation82–84]. The biogenesis of these EVs was likely regulated by the mixed lineage kinase 3 (MLK3)/mitogen-activated protein kinase kinase (MAP2K) 3 or MAP2K6/p38 mitogen-activated protein kinase/signal transducer and activator of transcription 1 pathway [Citation82, Citation84]. Mlk3-deficient mice were found to be protected from high-fat and high-carbohydrate diet-induced MASH by inhibiting c-Jun N-terminal kinase activation in a weight-independent manner [Citation85]. Hence, EV-encapsulated CXCL10 could be considered as a pro-disease factor. C-X-C motif chemokine receptor (CXCR) 1 and CXCR2 could determine the release of hepatocyte-derived exosomes [Citation86]. The CXCR1-deficient hepatocytes produced fewer exosomes than the control group, whereas the CXCR2-deficient ones enhanced exosome production by increasing the activities of neutral sphingomyelinase and intracellular ceramide [Citation86]. CXCR1 and CXCR2 are mainly activated by interleukin (IL)-8 (namely CXCL8) to activate various mediators in the recipient cells to induce inflammatory responses [Citation87]. Besides, IL-8 could be released from the inflammatory cells in liver and AT, and its levels were found to be significantly increased in obesity [Citation88, Citation89]. These findings indicate that in patients with MASLD/MASH complicated by obesity, the IL-8/CXCR1/2 pathway may participate in the secretion of lipid-rich exosomes and the pathological process of the liver. In addition, CXCR3 has been discovered to regulate immune responses by recruiting and activating leukocytes through binding to CXCL10 [Citation90].
Other mechanisms behind the hepatocyte-derived EVs and MASLD/MASH have been elucidated. Under lipotoxic conditions, hepatocyte-derived EVs have the tumour necrosis factor-related apoptosis-inducing ligand, which activates DR5 on macrophages and induces inflammation [Citation75]. And microvesicles secreted by fat-laden hepatocyte-origin cells could be taken up by cells of the same origin or macrophages, followed by activation of nucleotide-binding oligomerisation domain-like receptor family pyrin domain-containing 3 inflammasomes, and ultimately lead to an increase in IL-1β release [Citation91]. Additionally, active integrin subunit β1-bearing EVs secreted by lipotoxic hepatocytes facilitated monocyte adhesion to liver sinusoidal endothelial cells and monocyte-derived macrophage inflammation in mice with diet-induced MASH [Citation92]. Moreover, hepatocytes in mice and patients with MASH produced circulating microparticles carrying mitochondrial DNA to activate toll-like receptor 9, which mediates the development of high-fat-diet (HFD)-induced MASH [Citation93]. Consequently, in the lipotoxic environment of MASLD/MASH, various biomolecules encapsulated in hepatocyte-derived EVs promote disease progression by increasing the release of pro-inflammatory cytokines and activating and recruiting inflammatory cells.
Non-metabolic factors could influence hepatocyte-derived EVs and the disease progression. When hypoxia was incorporated, fat-laden hepatocyte-derived EVs stimulated Kupffer cells (KCs), which are liver-resident macrophages, to exhibit the pro-inflammatory phenotype [Citation94] and led to the expression of fibrotic markers in HSCs to promote fibrosis [Citation95]. This finding indicates that when patients with MASLD/MASH suffer from hypoxia, such as in obstructive sleep apnoea syndrome or cardiovascular disease, the liver disorder may be simultaneously exacerbated by the influenced EVs.
3.4. EV miRNAs in circulation and liver in MASLD/MASH
MiRNAs are critical agents carried by EVs that could regulate the target gene expression at the transcriptional level and head the cast in coordinating biological activities. The heterogeneity of EV miRNAs could reflect their source cells, microenvironment, and other conditions. Thus, these miRNAs might provide clues for diagnosis, assessment, and monitoring of disease severity and progression. MiRNAs present in circulating EVs are considered one of the most promising candidates for disease biomarkers due to their stability and specificity. In addition, the easy accessibility of circulating blood is also one of its advantages. Several circulating EV miRNAs have been identified as potential specific biomarkers for MASLD/MASH [Citation12, Citation28, Citation96–100]. These circulating EV miRNAs may be of great convenience in clinical practice, but they are in need of further clinical evidence. It is important to note that the current methods of EV isolation and characterisation pose a serious challenge to their clinical application.
To date, the effect of certain EV miRNAs on the pathogenesis of MASLD/MASH has been well established. MiR-122 is highly expressed in the liver, particularly in hepatocytes, and plays a pivotal role in cholesterol metabolism. It is closely associated with lipid levels in MASLD [Citation101]. The expression of miR-122 increases in circulating exosomes during MASLD/MASH [Citation28, Citation100–103], and its serum level is positively associated with disease severity [Citation101, Citation102]. Conversely, hepatic miR-122 is reduced and inversely regulates the modulators of tissue remodelling in MASH, thus playing a role in fibrosis [Citation28, Citation103]. Notably, macrophages activation is a hallmark of MASLD/MASH, and the significance of EV miRNAs in this process could not be overlooked. Hepatocyte-derived miR-122-5p-enriched exosomes enhanced M1 macrophage polarisation and macrophage-induced inflammation, and exosome release was increased by cholesterol via induction of lysosomal dysfunction to suppress MVB degradation [Citation62]. In addition to miR-122, miR-192 has been reported to play a role in macrophage activation. Similar to the former, plasma exosomal and hepatic miR-192 expression exhibited divergent alternations in CCl4-treated animals [Citation28]. Hepatocyte-derived exosomes were enriched in miR-192-5p in MASLD, and miR-192-5p could activate pro-inflammatory macrophages by regulating the rapamycin-insensitive companion of mammalian target of rapamycin (RICTOR)/Akt/forkhead box transcription factor O1 pathway [Citation104]. Besides, lipotoxic hepatocyte-derived exosomal miR-9-5p could activate macrophages in MASLD [Citation105]. After being taken up by macrophages, it may inhibit the expression of transglutaminase 2 (TGM2) and drive M1 macrophage polarisation, thus promoting disease progression [Citation105]. EVs and EV miRNAs could also induce fibrosis ulteriorly. Steatotic hepatocyte-derived EVs could influence the phenotypes and remodel the expression levels of HSC markers [Citation106]. In lipotoxic circumstances, HSCs are activated by hepatocyte-derived EV miR-128-3p through the suppression of peroxisome proliferator-activated receptor (PPAR)-γ in recipient cells [Citation107]. This suppression increased pro-fibrogenic genes, proliferation, and wound healing responses [Citation107, Citation108]. Since they contribute to hepatic inflammation and fibrosis in MASLD/MASH, targeting these liver-origin EV miRNAs or the downstream pathways to inhibit the molecular activity or block the pathways may be one of the ideal strategies to alleviate and treat MASLD/MASH.
Few studies have demonstrated that EV miRNAs have the potential to alleviate the disease. EV miR-690 derived from KCs has potential as a therapeutic strategy because it could attenuate de novo lipogenesis in hepatocytes, inflammation of recruited hepatic macrophages, and fibrogenesis in HSCs by targeting NAD kinase (NADK) [Citation109]. And during the progression of MASH, the level of miR-690 decreased in the liver with KCs becoming miR-690 deficient [Citation109]. Another miRNA known for its anti-fibrotic properties, miR-223, is abundant in neutrophils and macrophages [Citation110]. It has been shown to reduce the hepatic inflammatory response and fibrosis in MASH [Citation111]. In hepatocytes, CXCL10, WW domain-containing transcription regulator 1 (WWTR1), NLR family pyrin domain-containing 3 (NLRP3), and insulin-like growth factor 1 receptor (IGF1R) were found to be as miR-223 target genes that play a pivotal role in the induction of inflammation and fibrosis [Citation110, Citation111]. The selective uptake of miR-223-enriched EVs by hepatocytes may rely on the apolipoprotein E on EVs and the low-density lipoprotein receptor on hepatocytes, which could be enhanced by free fatty acids (FFAs) [Citation112]. In addition to hepatocytes, macrophages could also be recipients of miR-233. Neutrophil-derived EV miR-223 could reverse the pro-inflammatory phenotype of hepatic macrophages by inhibiting NLRP3 [Citation113]. Moreover, the level of miR-223-enriched exosomes released from macrophages is increased under the induction of IL-6 [Citation110]. And this miRNA could form a negative feedback loop to limit neutrophil over-activation [Citation114] and prevent the progression of simple steatosis to MASH [Citation111]. Although both detrimental and beneficial EV miRNAs have been discovered in MASLD/MASH, the aggravating factors outweigh the ameliorating factors in the disease process, leading to the progression of MASLD/MASH. The miRNAs of liver-derived EVs driven by lipid accumulation are shown in .
Table 3. MiRNAs from liver-derived EVs acting on the liver in MASLD/MASH, driven by lipid accumulation.
4. Crosstalk between AT and liver via EVs in MASLD/MASH
In addition to resident cells in the liver, EVs secreted from other tissues or organs could be released into the circulation to act on the liver. Among these, AT is difficult to ignore because of its multiple roles in pathophysiological regulation. In addition to being a storage depot for lipids, AT has been widely recognised as an endocrine organ in the human body. Animal experiments have demonstrated that AT dysfunction, characterised by increased macrophage infiltration and inflammation, has a close relationship with MASH pathogenesis, accompanied by increased expression of numerous cytokines and chemokines [Citation115]. In addition, AT could induce phenotypic changes in macrophages. In contrast to typical lipolysis, adipocytes were found to deliver lipids to local macrophages through lipid-laden exosome-sized vesicles, which significantly augmented the lipid accumulation in macrophages [Citation116]. Such vesicles could also induce the differentiation of bone marrow progenitor cells into AT macrophage-like cells, which is another mechanism for modulating macrophages differentiation and function [Citation116]. AT is a major source of circulating EV miRNAs, which may regulate whole-body metabolism and hepatic gene expression [Citation22]. And obesity and MASLD/MASH may interact via EVs. For example, the lean mice showed hepatic steatosis and obesity after being injected with the exosomes transfected with recognised miRNA mimics (miR-122, miR-192, miR-27a-3p, and miR-27b-3p) [Citation117]. Therefore, the crosstalk between the liver and AT via EVs is significant in maintaining lipid homeostasis and MASLD/MASH ().
Figure 4. Crosstalk between AT and liver via EVs in MASLD/MASH.
The crosstalk between AT and the liver via EVs is significant in MASLD/MASH. Several AT-derived EVs enveloping miRNAs, Akr1b7, CD36, miR-99b, or miR-132-3p are released into circulation to regulate hepatic pathological processes. Meanwhile, hepatocyte-derived exosomal let-7b-5p could modulate energy expenditure and WAT browning in AT, thereby accelerating HFD-induced steatosis and obesity. The exosome content may have either a beneficial or detrimental effect on the target cells or tissues, as indicated by the green and red arrows respectively. The target genes of EV miRNAs that interact between the two organs are annotated. This figure was created by the authors of this article.
Abbreviations: ADRB3, adrenoceptor beta 3.
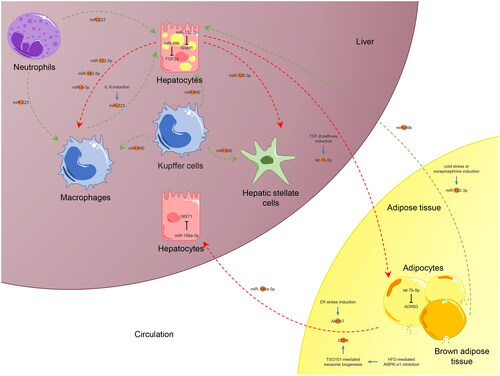
AT is thought to influence the pathophysiological process in the liver of MASLD/MASH through paracrine pathways, particularly EVs. ER stress-induced adipocyte-derived exosomes could transport aldo-keto reductase family 1 member B7 (Akr1b7) to the liver in mice, thus coordinating lipid metabolism [Citation118]. Another study demonstrated that HFD-mediated AMP-activated protein kinase (AMPK)-α1 inhibition contributed to MASLD by promoting tumour susceptibility 101 (TSG101)-mediated AT-derived CD36-rich exosome biogenesis [Citation119]. And CD36 has been thought to promote steatosis and steatohepatitis, which is regulated by PPAR-γ [Citation120]. Furthermore, miR-199a-5p was observed to be significantly increased in an HFD-induced steatosis mouse model [Citation121]. Known as a pro-fibrotic miRNA, it is abundantly expressed in visceral adipose tissue (VAT) and could be regulated by FFAs, cytokines, and adipokines [Citation28, Citation122]. Exosomal miR-199a-5p could aggravate hepatic lipid accumulation in hepatocytes via macrophage stimulating 1 (MST1)-related lipid metabolism pathways [Citation121].
AT usually refers to white adipose tissue (WAT), although brown adipose tissue (BAT) also exists in the human body. WAT is mainly participating in energy storage, whereas BAT is responsible for energy dissipation through fuel oxidation and thermogenesis [Citation123]. BAT-derived exosomes are more efficient in targeting the liver than WAT-derived ones [Citation22]. Some BAT-origin EVs are beneficial for MASLD/MASH. Under cold stress or norepinephrine induction, miR-132-3p was elevated in BAT-derived exosomes and could affect the expression levels of hepatic sterol regulatory element binding transcription factor 1 (Srebf1) and some lipogenic genes in mice, thereby lessening hepatic lipogenesis [Citation31]. Exosomal miR-99b secreted by BAT, but not WAT, could significantly decrease the hepatic fibroblast growth factor (FGF)-21 mRNA, thereby alleviating liver fibrosis [Citation22]. Another type of adipocytes, called beige adipocytes, is found in specific fat depots or under certain conditions in WAT [Citation123]. Beige adipocytes are partially similar in characterisation to BAT. Human ADSC-derived EVs, extracted during beige adipogenic differentiation, had a solid ability to prevent lipid accumulation in the liver of HFD-fed mice and to increase glucose sensitivity and reduce hepatic triglyceride levels [Citation32].
By location, AT is classified as VAT and subcutaneous adipose tissue (SAT). Visceral adiposity is one of the risk factors for MASLD/MASH [Citation124]. It has been observed that the expression of inflammatory genes is increased in VAT of patients with MASLD [Citation125]. VAT regulates the inflammatory and fibrotic signalling pathways in other organs, possibly through EVs [Citation126, Citation127]. An in vitro study found that VAT-derived exosomes could induce dysregulation of transforming growth factor (TGF)-β pathway members in hepatocytes [Citation126], and the signalling pathway was associated with MASLD. Withal, SAT, together with VAT, contributes to the progression of MASLD to MASH [Citation125]. In conclusion, AT-derived EVs could be released into the circulation to interfere with the pathophysiological processes in the liver of patients with MASLD/MASH, and the roles of different types of AT vary.
In MASLD/MASH, EVs produced by liver-origin cells could reversely affect AT. Several EV-derived miRNAs in the serum of patients with MASLD benefit adipocyte lipid accumulation, and their expression levels have been proven to positively correlate with BMI [Citation64]. When a liver-specific gene thought to regulate EV release, such as GGPPS, is deficient, fat deposition may be reduced [Citation64]. Hepatocyte-derived EVs could also modulate energy expenditure in BAT or browning of WAT [Citation128]. When cells were stimulated by FFAs and TGF-β signalling, hepatocyte-derived exosomal let-7b-5p significantly increased and aggravated steatosis and obesity by decreasing mitochondrial oxidative phosphorylation, thus promoting adipocyte whitening and inhibiting their browning [Citation128]. Therefore, the liver and AT may interact via EVs to jointly induce the metabolic syndrome in MASLD/MASH patients.
5. Therapeutic potential of ADSC-derived EVs in MASLD/MASH
ADSCs are characterised by infinite proliferation and differentiation capacities, and acquiring vast ADSCs from AT is relatively straightforward. They have also been confirmed to be vital in controlling obesity-related inflammation and metabolic diseases [Citation129]. In brief, ADSCs may have a favourable therapeutic effect on the disorder. Moreover, EVs have similar pathophysiological effects to their source cells [Citation10, Citation15–17], and avoid many disadvantages of cell therapy [Citation10, Citation14, Citation15]. Previous studies demonstrated that mesenchymal stem cell-derived EVs could improve hepatic glucose and lipid metabolism and modulate the anti-inflammatory phenotype of regulatory macrophages [Citation130, Citation131]. The EVs derived from various cell sources, such as human liver stem cells [Citation132], human umbilical cord-derived mesenchymal stem cells (UCMSCs) [Citation133, Citation134], and bone marrow mesenchymal stem cells (BMSCs) [Citation135], have shown to slow down or reverse the disease progression of MASLD/MASH. For example, UCMSC-derived miR-627-5p-enriched exosomes could promote cell viability and inhibit apoptosis of palmitate-treated hepatocytes [Citation133]. They could alleviate liver damage, glucose and lipid metabolism, and reduce lipid deposition by inhibiting the obesity-associated gene, thereby reducing the progression of MASLD [Citation133]. Furthermore, UCMSC-derived exosomes enriched in Beclin-1 could accelerate ferroptosis in HSCs and ameliorate liver fibrosis via the xCT/glutathione peroxidase 4 pathway [Citation134]. Consequently, ADSC-derived EVs are a kind of up-and-coming non-invasive therapeutic method for liver injury.
Several studies have demonstrated the potential therapeutic role of ADSC-derived EVs in MASLD/MASH and its associated pathophysiological process. The ADSC-conditioned medium (containing abundant EVs) could alleviate the hepatocyte steatosis and regulate the levels of several hepatic lipids in the animal model of liver injury [Citation136]. And ADSC-derived exosomes remit hepatic steatosis and improve insulin sensitivity, thereby contributing to metabolic homeostasis in HFD-fed mice [Citation23]. In animal models of MASH with fibrosis, ADSC or EV injection could reduce hepatic inflammation and fibrosis, possibly in a dose-dependent manner [Citation33].
ADSC-derived EVs have tremendous advantages as a therapeutic strategy. First, AT is a reservoir of ADSCs, possessing abundant stem cells. ADSCs could be harvested with limited surgical damage, and the acquisition technique is relatively simple and convenient. These characteristics make ADSCs as a safer and more suitable source of EVs for clinical use compared to other mesenchymal stem cells. They have unlimited proliferation and differentiation potential, and the in vitro culture method is mature, which theoretically allows the collection of numerous source cells. Second, ADSCs and their EVs have similar therapeutic results in various abnormalities and diseases, including MASLD/MASH. Third, EVs eliminate various disadvantages of cell therapy, such as low biocompatibility and cell survival rate. In addition, EVs could be edited with relative flexibility, showing infinite possibilities for delivering miRNAs or drugs of interest to target organs. These characteristics of ADSC-derived EVs make them easy to industrialise, commercialise, and attract the full interest of researchers.
6. Clinical applications of EVs in MASLD/MASH
The possibilities for EVs in diagnosis and therapy are endless, with multiple potential directions for research and development. Due to their heterogeneity in different pathophysiological states, EVs and their specific miRNAs in body fluids have been proposed as biomarkers for disease diagnosis and monitoring and prognostic assessment in the clinic [Citation137–139]. Several clinical trials have been completed, but enormous obstacles lie ahead. For example, the search for EV biomarkers with both sensitivity and specificity is ongoing. These markers are currently far from being ready for clinical use.
There are several important considerations before translating them to the clinic. First, reliable isolation and precise characterisation of EVs are urgently needed prior to large-scale application, as most present extraction methods and characterisation approaches lack rapidity, practicality, and cost-effectiveness. Second, off-target effects and safety of EVs must be addressed. Currently, most of the studies on the potential therapeutic role of EVs are mainly conducted in the laboratory, while clinical trials are few. At least hundreds of EV-related clinical trials are registered in www.clinicaltrials.gov (until June 10, 2023). And there are no registered clinical trials or corresponding reports of EV treatment in MASLD/MASH. Among the completed and reported trials, allogeneic BMSC-derived EVs were used in severe COVID-19 [Citation140] (NCT04493242), indicating the excellent therapeutic safety and efficacy of stem cell-derived EVs. Although the laboratory study demonstrated that ADSC-derived EVs show the same inspiring treatment results as their source cells for MASLD/MASH [Citation33], their application to the clinic is still a long way off.
7. Future perspectives of EV therapy in MASLD/MASH
Due to the extensive and deep involvement of EVs derived from liver and AT in MASLD/MASH, targeting EVs with negative effects and its active biocomponents would be the most direct form of treatment. For example, sphingolipid ceramide is involved in exosome biogenesis [Citation58], so sphingomyelinase inhibition could be an effective measure. And targeted knockdown or inhibition of GGPPS and Rab27A genes may reduce exosome secretion [Citation64, Citation76]. Approaches that target specific miRNAs for therapeutic benefits must be based on a thorough understanding of the mechanisms of miRNA action. However, although progress has been made in the understanding of liver- and AT-derived EVs and MASLD/MASH, most of the underlying molecular mechanisms remain unexplored and none of these approaches has reached to the clinical stage.
The most intriguing ability of EVs to deliver therapeutic drugs or molecules for selective therapy. Thanks to the development of engineered EVs, they have several unique advantages as ideal drug carriers. First, they have good biocompatibility, low toxicity, and relative stability in circulation, which could reduce the clearance and improve the efficiency of the delivered drug. Besides, EVs could ensure the stability of the cargo, especially for miRNAs, to enable their delivery to a distant target in an intact form. Second, EVs are capable of targeted delivery depending on their surface and membrane protein composition [Citation141]. For instance, in engineered EVs, modifying the ligand through transfection or chemical means can enhance its targeting and therapeutic efficacy. In addition, the drug could be encapsulated in EVs either endogenously or exogenously [Citation141], with a wide choice. There are two main methods for loading engineered EVs with therapeutic cargoes. The first involves the integration of the drug into the producing cells, such as by transfection, and obtaining the drug-loaded EVs through natural EV production. The second approach involves collecting EVs from various sources, such as cultured cells and milk, and introducing cargoes into them using different technologies, including electroporation and surfactant treatment. Fourth, EVs could be used in various forms to facilitate clinical drug delivery. Topical or intravenous administration could be used as a means of targeted drug delivery.
8. Conclusions
As one of the leading causes of chronic liver disease, MASLD/MASH places an enormous clinical and economic burden on individuals and society. Understanding the critical factors in the pathophysiological development of MASLD/MASH is beneficial for disease diagnosis and therapy. Among them, EVs have attracted growing interest from researchers. In MASLD/MASH, EVs and certain internal miRNAs, especially those derived from liver and AT resident cells, play a role in both the development and progression of the disease, either in a harmful or beneficial capacity. An in-depth study of the disease’s underlying mechanism may help identify targeted measures for its alleviation or cure. Due to the benefits of ADSC-derived and engineered EVs, they have potential therapeutic efficacy and promising clinical applications for MASLD/MASH. Therefore, more in-depth mechanistic studies and clinical trials for MASLD/MASH, as well as further development of ADSC-derived and engineered EVs, are needed to shed light on future therapies.
Author contributions
Wandi Li collected the literature, was a major contributor to the writing of the manuscript, provided or drew the figures, and obtained funding. Lili Yu revised the manuscript and obtained funding. All authors read and approved the final manuscript.
Disclosure statement
No potential conflict of interest was reported by the author(s).
Data availability statement
Data sharing is not applicable to this article as no new data were created or analysed in this study.
Additional information
Funding
References
- Rinella ME, Lazarus JV, Ratziu V, et al. A multisociety Delphi consensus statement on new fatty liver disease nomenclature. Hepatology. 2023;78(6):1966–1986. doi: 10.1097/hep.0000000000000520.
- Younossi ZM, Golabi P, Paik JM, et al. The global epidemiology of nonalcoholic fatty liver disease (NAFLD) and nonalcoholic steatohepatitis (Nash): a systematic review. Hepatology. 2023;77(4):1335–1347. doi: 10.1097/hep.0000000000000004.
- Zhou F, Zhou J, Wang W, et al. Unexpected rapid increase in the burden of NAFLD in China from 2008 to 2018: a systematic review and meta-analysis. Hepatology. 2019;70(4):1119–1133. doi: 10.1002/hep.30702.
- Estes C, Anstee QM, Arias-Loste MT, et al. Modeling NAFLD disease burden in China, France, Germany, Italy, Japan, Spain, United Kingdom, and United States for the period 2016-2030. J Hepatol. 2018;69(4):896–904. doi: 10.1016/j.jhep.2018.05.036.
- Farrell GC, Haczeyni F, Chitturi S. Pathogenesis of nash: how metabolic complications of overnutrition favour lipotoxicity and pro-inflammatory fatty liver disease. Adv Exp Med Biol. 2018;1061:19–44. doi: 10.1007/978-981-10-8684-7_3.
- Parthasarathy G, Revelo X, Malhi H. Pathogenesis of nonalcoholic steatohepatitis: an overview. Hepatol Commun. 2020;4(4):478–492. doi: 10.1002/hep4.1479.
- Geng Y, Faber KN, De Meijer VE, et al. How does hepatic lipid accumulation lead to lipotoxicity in non-Alcoholic fatty liver disease? Hepatol Int. 2021;15(1):21–35. doi: 10.1007/s12072-020-10121-2.
- Younossi ZM, Koenig AB, Abdelatif D, et al. Global epidemiology of nonalcoholic fatty liver disease-meta-analytic assessment of prevalence, incidence, and outcomes. Hepatology. 2016;64(1):73–84. doi: 10.1002/hep.28431.
- Henry L, Paik J, Younossi ZM. Review article: the epidemiologic burden of non-alcoholic fatty liver disease across the world. Aliment Pharmacol Ther. 2022;56(6):942–956. doi: 10.1111/apt.17158.
- Varderidou-Minasian S, Lorenowicz MJ. Mesenchymal stromal/stem cell-derived extracellular vesicles in tissue repair: challenges and opportunities. Theranostics. 2020;10(13):5979–5997. doi: 10.7150/thno.40122.
- Han C, Yang J, Sun J, et al. Extracellular vesicles in cardiovascular disease: biological functions and therapeutic implications. Pharmacol Ther. 2022;233:108025. doi: 10.1016/j.pharmthera.2021.108025.
- Newman LA, Muller K, Rowland A. Circulating cell-specific extracellular vesicles as biomarkers for the diagnosis and monitoring of chronic liver diseases. Cell Mol Life Sci. 2022;79(5):232. doi: 10.1007/s00018-022-04256-8.
- Kalluri R, Lebleu VS. The biology, function, and biomedical applications of exosomes. Science. 2020;367(6478):eaau6977. doi: 10.1126/science.aau6977.
- Dilsiz N. Hallmarks of exosomes. Future Sci OA. 2021;8(1):FSO764. doi: 10.2144/fsoa-2021-0102.
- Vizoso FJ, Eiro N, Cid S, et al. Mesenchymal stem cell secretome: toward cell-free therapeutic strategies in regenerative medicine. Int J Mol Sci. 2017;18(9):1852. doi: 10.3390/ijms18091852.
- Chen B, Cai J, Wei Y, et al. Exosomes are comparable to source adipose stem cells in fat graft retention with up-regulating early inflammation and angiogenesis. Plast Reconstr Surg. 2019;144(5):816e–827e. doi: 10.1097/PRS.0000000000006175.
- Ghiasloo M, De Wilde L, Singh K, et al. A systematic review on extracellular vesicles-enriched fat grafting: a shifting paradigm. Aesthet Surg J. 2021;41(11):NP1695–NP1705. doi: 10.1093/asj/sjaa362.
- Cocozza F, Grisard E, Martin-Jaular L, et al. Snapshot: extracellular vesicles. Cell. 2020;182(1):262–262.e261. doi: 10.1016/j.cell.2020.04.054.
- Eitan E, Suire C, Zhang S, et al. Impact of lysosome status on extracellular vesicle content and release. Ageing Res Rev. 2016;32:65–74. doi: 10.1016/j.arr.2016.05.001.
- Szatanek R, Baj-Krzyworzeka M, Zimoch J, et al. The methods of choice for extracellular vesicles (evs) characterization. Int J Mol Sci. 2017;18(6):1153. doi: 10.3390/ijms18061153.
- Sidhom K, Obi PO, Saleem A. A review of exosomal isolation methods: is size exclusion chromatography the best option? Int J Mol Sci. 2020;21(18):6466. doi: 10.3390/ijms21186466.
- Thomou T, Mori MA, Dreyfuss JM, et al. Adipose-derived circulating miRNAs regulate gene expression in other tissues. Nature. 2017;542(7642):450–455. doi: 10.1038/nature21365.
- Zhao H, Shang Q, Pan Z, et al. Exosomes from adipose-derived stem cells attenuate adipose inflammation and obesity through polarizing M2 macrophages and beiging in white adipose tissue. Diabetes. 2018;67(2):235–247. doi: 10.2337/db17-0356.
- Lagos-Quintana M, Rauhut R, Lendeckel W, et al. Identification of novel genes coding for small expressed RNAs. Science. 2001;294(5543):853–858. doi: 10.1126/science.1064921.
- Lau NC, Lim LP, Weinstein EG, et al. An abundant class of tiny RNAs with probable regulatory roles in caenorhabditis elegans. Science. 2001;294(5543):858–862. doi: 10.1126/science.1065062.
- Berezikov E, Guryev V, Van De Belt J, et al. Phylogenetic shadowing and computational identification of human microrna genes. Cell. 2005;120(1):21–24. doi: 10.1016/j.cell.2004.12.031.
- Mahmoudi A, Butler AE, Jamialahmadi T, et al. The role of exosomal miRNA in nonalcoholic fatty liver disease. J Cellular Physiol. 2022;237(4):2078–2094. doi: 10.1002/jcp.30699.
- Broermann A, Schmid R, Gabrielyan O, et al. Exosomal miRNAs as potential biomarkers to monitor phosphodiesterase 5 inhibitor induced anti-fibrotic effects on Ccl4 treated rats. Int J Mol Sci. 2020;22(1):382. doi: 10.3390/ijms22010382.
- Hu J, Jiang Y, Wu X, et al. Exosomal mir-17-5p from adipose-derived mesenchymal stem cells inhibits abdominal aortic aneurysm by suppressing Txnip-Nlrp3 inflammasome. Stem Cell Res Ther. 2022;13(1):349. doi: 10.1186/s13287-022-03037-1.
- Martínez MC, Andriantsitohaina R. Extracellular vesicles in metabolic syndrome. Circ Res. 2017;120(10):1674–1686. doi: 10.1161/circresaha.117.309419.
- Kariba Y, Yoshizawa T, Sato Y, et al. Brown adipocyte-derived exosomal mir-132-3p suppress hepatic Srebf1 expression and thereby attenuate expression of lipogenic genes. Biochem Biophys Res Commun. 2020;530(3):500–507. doi: 10.1016/j.bbrc.2020.05.090.
- Jung YJ, Kim HK, Cho Y, et al. Cell reprogramming using extracellular vesicles from differentiating stem cells into white/beige adipocytes. Sci Adv. 2020;6(13):eaay6721. doi: 10.1126/sciadv.aay6721.
- Watanabe T, Tsuchiya A, Takeuchi S, et al. Development of a non-alcoholic steatohepatitis model with rapid accumulation of fibrosis, and its treatment using mesenchymal stem cells and their small extracellular vesicles. Regen Ther. 2020;14:252–261. doi: 10.1016/j.reth.2020.03.012.
- Mahmood A, Seetharaman R, Kshatriya P, et al. Stem cell transplant for advanced stage liver disorders: current scenario and future prospects. CMC. 2020;27(37):6276–6293. doi: 10.2174/0929867326666191004161802.
- Mathieu M, Martin-Jaular L, Lavieu G, et al. Specificities of secretion and uptake of exosomes and other extracellular vesicles for cell-to-cell communication. Nat Cell Biol. 2019;21(1):9–17. doi: 10.1038/s41556-018-0250-9.
- Couch Y, Buzas EI, Di Vizio D, et al. A brief history of nearly Ev-Erything - the rise and rise of extracellular vesicles. J Extracell Vesicles. 2021;10(14):e12144. doi: 10.1002/jev2.12144.
- Théry C, Zitvogel L, Amigorena S. Exosomes: composition, biogenesis and function. Nat Rev Immunol. 2002;2(8):569–579. doi: 10.1038/nri855.
- Chen Y, Zhao Y, Yin Y, et al. Mechanism of cargo sorting into small extracellular vesicles. Bioengineered. 2021;12(1):8186–8201. doi: 10.1080/21655979.2021.1977767.
- Brennan K, Martin K, Fitzgerald SP, et al. A comparison of methods for the isolation and separation of extracellular vesicles from protein and lipid particles in human serum. Sci Rep. 2020;10(1):1039. doi: 10.1038/s41598-020-57497-7.
- Gardiner C, Di Vizio D, Sahoo S, et al. Techniques used for the isolation and characterization of extracellular vesicles: results of a worldwide survey. J Extracell Vesicles. 2016;5(1):32945. doi: 10.3402/jev.v5.32945.
- Théry C, Witwer KW, Aikawa E, et al. Minimal information for studies of extracellular vesicles 2018 (Misev2018): a position statement of the international society for extracellular vesicles and update of the Misev2014 guidelines. J Extracell Vesicles. 2018;7(1):1535750. doi: 10.1080/20013078.2018.1535750.
- Royo F, Théry C, Falcón-Pérez JM, et al. Methods for separation and characterization of extracellular vesicles: results of a worldwide survey performed by the isev rigor and standardization subcommittee. Cells. 2020;9(9):1955. doi: 10.3390/cells9091955.
- Wang JM, Li YJ, Wu JY, et al. Comparative evaluation of methods for isolating small extracellular vesicles derived from pancreatic cancer cells. Cell Biosci. 2021;11(1):37. doi: 10.1186/s13578-021-00550-3.
- Linares R, Tan S, Gounou C, et al. High-speed centrifugation induces aggregation of extracellular vesicles. J Extracell Vesicles. 2015;4(1):29509. doi: 10.3402/jev.v4.29509.
- Lobb RJ, Becker M, Wen SW, et al. Optimized exosome isolation protocol for cell culture supernatant and human plasma. J Extracell Vesicles. 2015;4(1):27031. doi: 10.3402/jev.v4.27031.
- Yang J, Gao X, Xing X, et al. An isolation system to collect high quality and purity extracellular vesicles from serum. Int J Nanomedicine. 2021;16:6681–6692. doi: 10.2147/IJN.S328325.
- Baranyai T, Herczeg K, Onódi Z, et al. Isolation of exosomes from blood plasma: qualitative and quantitative comparison of ultracentrifugation and size exclusion chromatography methods. PLoS One. 2015;10(12):e0145686. doi: 10.1371/journal.pone.0145686.
- Wei R, Zhao L, Kong G, et al. Combination of size-exclusion chromatography and ultracentrifugation improves the proteomic profiling of plasma-derived small extracellular vesicles. Biol Proced Online. 2020;22(1):12. doi: 10.1186/s12575-020-00125-5.
- Huang K, Garimella S, Clay-Gilmour A, et al. Comparison of human urinary exosomes isolated via ultracentrifugation alone versus ultracentrifugation followed by sec column-purification. J Pers Med. 2022;12(3):340. doi: 10.3390/jpm12030340.
- Mousavi SM, Amin Mahdian SM, Ebrahimi MS, et al. Microfluidics for detection of exosomes and microRNAs in cancer: state of the art. Mol Ther Nucleic Acids. 2022;28:758–791. doi: 10.1016/j.omtn.2022.04.011.
- Zhang H, Freitas D, Kim HS, et al. Identification of distinct nanoparticles and subsets of extracellular vesicles by asymmetric flow field-flow fractionation. Nat Cell Biol. 2018;20(3):332–343. doi: 10.1038/s41556-018-0040-4.
- Filipe V, Hawe A, Jiskoot W. Critical evaluation of nanoparticle tracking analysis (NTA) by nanosight for the measurement of nanoparticles and protein aggregates. Pharm Res. 2010;27(5):796–810. doi: 10.1007/s11095-010-0073-2.
- Dragovic RA, Gardiner C, Brooks AS, et al. Sizing and phenotyping of cellular vesicles using nanoparticle tracking analysis. Nanomedicine. 2011;7(6):780–788. doi: 10.1016/j.nano.2011.04.003.
- Akers JC, Ramakrishnan V, Nolan JP, et al. Comparative analysis of technologies for quantifying extracellular vesicles (EVS) in clinical cerebrospinal fluids (CSF). PLoS One. 2016;11(2):e0149866. doi: 10.1371/journal.pone.0149866.
- Zhang Y, Bi J, Huang J, et al. Exosome: a review of its classification, isolation techniques, storage, diagnostic and targeted therapy applications. Int J Nanomedicine. 2020;15:6917–6934. doi: 10.2147/IJN.S264498.
- Liu X, Zong Z, Xing M, et al. pH-mediated clustering of exosomes: breaking through the size limit of exosome analysis in conventional flow cytometry. Nano Lett. 2021;21(20):8817–8823. doi: 10.1021/acs.nanolett.1c03211.
- Van Der Pol E, Coumans FA, Grootemaat AE, et al. Particle size distribution of exosomes and microvesicles determined by transmission electron microscopy, flow cytometry, nanoparticle tracking analysis, and resistive pulse sensing. J Thromb Haemost. 2014;12(7):1182–1192. doi: 10.1111/jth.12602.
- Trajkovic K, Hsu C, Chiantia S, et al. Ceramide triggers budding of exosome vesicles into multivesicular endosomes. Science. 2008;319(5867):1244–1247. doi: 10.1126/science.1153124.
- Nakao Y, Amrollahi P, Parthasarathy G, et al. Circulating extracellular vesicles are a biomarker for NAFLD resolution and response to weight loss surgery. Nanomedicine. 2021;36:102430. doi: 10.1016/j.nano.2021.102430.
- Landa S, Verlov N, Fedorova N, et al. Extracellular particles as carriers of cholesterol not associated with lipoproteins. Membranes (Basel). 2022;12(6):618. doi: 10.3390/membranes12060618.
- Wang W, Zhu N, Yan T, et al. The crosstalk: exosomes and lipid metabolism. Cell Commun Signal. 2020;18(1):119. doi: 10.1186/s12964-020-00581-2.
- Zhao Z, Zhong L, Li P, et al. Cholesterol impairs hepatocyte lysosomal function causing M1 polarization of macrophages via exosomal mir-122-5p. Exp Cell Res. 2020;387(1):111738. doi: 10.1016/j.yexcr.2019.111738.
- Abdullah M, Nakamura T, Ferdous T, et al. Cholesterol regulates exosome release in cultured astrocytes. Front Immunol. 2021;12:722581. doi: 10.3389/fimmu.2021.722581.
- Zhao Y, Zhao MF, Jiang S, et al. Liver governs adipose remodelling via extracellular vesicles in response to lipid overload. Nat Commun. 2020;11(1):719. doi: 10.1038/s41467-020-14450-6.
- Yang L, Wang T, Zhang X, et al. Exosomes derived from human placental mesenchymal stem cells ameliorate myocardial infarction via anti-inflammation and restoring gut dysbiosis. BMC Cardiovasc Disord. 2022;22(1):61. doi: 10.1186/s12872-022-02508-w.
- Fujita K, Somiya M, Kuroda S, et al. Induction of lipid droplets in non-macrophage cells as well as macrophages by liposomes and exosomes. Biochem Biophys Res Commun. 2019;510(1):184–190. doi: 10.1016/j.bbrc.2019.01.078.
- Povero D, Eguchi A, Li H, et al. Circulating extracellular vesicles with specific proteome and liver microRNAs are potential biomarkers for liver injury in experimental fatty liver disease. PLoS One. 2014;9(12):e113651. doi: 10.1371/journal.pone.0113651.
- Kakazu E, Mauer AS, Yin M, et al. Hepatocytes release ceramide-enriched pro-inflammatory extracellular vesicles in an Ire1alpha-dependent manner. J Lipid Res. 2016;57(2):233–245. doi: 10.1194/jlr.M063412.
- Li J, Liu H, Mauer AS, et al. Characterization of cellular sources and circulating levels of extracellular vesicles in a dietary murine model of nonalcoholic steatohepatitis. Hepatol Commun. 2019;3(9):1235–1249. doi: 10.1002/hep4.1404.
- Zhang J, Tan J, Wang M, et al. Lipid-induced dram recruits stom to lysosomes and induces LMP to promote exosome release from hepatocytes in NAFLD. Sci Adv. 2021;7(45):eabh1541. doi: 10.1126/sciadv.abh1541.
- Povero D, Eguchi A, Niesman IR, et al. Lipid-induced toxicity stimulates hepatocytes to release angiogenic microparticles that require vanin-1 for uptake by endothelial cells. Sci Signal. 2013;6(296):ra88. doi: 10.1126/scisignal.2004512.
- Povero D, Yamashita H, Ren W, et al. Characterization and proteome of circulating extracellular vesicles as potential biomarkers for nash. Hepatol Commun. 2020;4(9):1263–1278. doi: 10.1002/hep4.1556.
- Kornek M, Lynch M, Mehta SH, et al. Circulating microparticles as disease-specific biomarkers of severity of inflammation in patients with hepatitis C or nonalcoholic steatohepatitis. Gastroenterology. 2012;143(2):448–458. doi: 10.1053/j.gastro.2012.04.031.
- Welsh JA, Scorletti E, Clough GF, et al. Leukocyte extracellular vesicle concentration is inversely associated with liver fibrosis severity in NAFLD. J Leukoc Biol. 2018;104(3):631–639. doi: 10.1002/JLB.5A1217-501R.
- Hirsova P, Ibrahim SH, Krishnan A, et al. Lipid-induced signaling causes release of inflammatory extracellular vesicles from hepatocytes. Gastroenterology. 2016;150(4):956–967. doi: 10.1053/j.gastro.2015.12.037.
- Ostrowski M, Carmo NB, Krumeich S, et al. Rab27a and Rab27b control different steps of the exosome secretion pathway. Nat Cell Biol. 2010;12(1):19–30. doi: 10.1038/ncb2000.
- Liao CY, Song MJ, Gao Y, et al. Hepatocyte-derived lipotoxic extracellular vesicle sphingosine 1-phosphate induces macrophage chemotaxis. Front Immunol. 2018;9:2980. doi: 10.3389/fimmu.2018.02980.
- Dasgupta D, Nakao Y, Mauer AS, et al. Ire1a stimulates hepatocyte-derived extracellular vesicles that promote inflammation in mice with steatohepatitis. Gastroenterology. 2020;159(4):1487–1503.e1417. doi: 10.1053/j.gastro.2020.06.031.
- Fukushima M, Dasgupta D, Mauer AS, et al. Star-related lipid transfer domain 11 (Stard11)-mediated ceramide transport mediates extracellular vesicle biogenesis. J Biol Chem. 2018;293(39):15277–15289. doi: 10.1074/jbc.RA118.002587.
- Mauer AS, Hirsova P, Maiers JL, et al. Inhibition of sphingosine 1-phosphate signaling ameliorates murine nonalcoholic steatohepatitis. Am J Physiol Gastrointest Liver Physiol. 2017;312(3):G300–G313. doi: 10.1152/ajpgi.00222.2016.
- Zhang X, Shen J, Man K, et al. Cxcl10 plays a key role as an inflammatory mediator and a non-Invasive biomarker of non-alcoholic steatohepatitis. J Hepatol. 2014;61(6):1365–1375. doi: 10.1016/j.jhep.2014.07.006.
- Ibrahim SH, Hirsova P, Tomita K, et al. Mixed lineage kinase 3 mediates release of C-X-C motif ligand 10-bearing chemotactic extracellular vesicles from lipotoxic hepatocytes. Hepatology. 2016;63(3):731–744. doi: 10.1002/hep.28252.
- Tomita K, Freeman BL, Bronk SF, et al. Cxcl10-mediates macrophage, but not other innate immune cells-associated inflammation in murine nonalcoholic steatohepatitis. Sci Rep. 2016;6(1):28786. doi: 10.1038/srep28786.
- Tomita K, Kabashima A, Freeman BL, et al. Mixed lineage kinase 3 mediates the induction of Cxcl10 by a Stat1-dependent mechanism during hepatocyte lipotoxicity. J Cellular Biochem. 2017;118(10):3249–3259. doi: 10.1002/jcb.25973.
- Ibrahim SH, Gores GJ, Hirsova P, et al. Mixed lineage kinase 3 deficient mice are protected against the high fat high carbohydrate diet-induced steatohepatitis. Liver Int. 2014;34(3):427–437. doi: 10.1111/liv.12353.
- Nojima H, Konishi T, Freeman CM, et al. Chemokine receptors, Cxcr1 and Cxcr2, differentially regulate exosome release in hepatocytes. PLoS One. 2016;11(8):e0161443. doi: 10.1371/journal.pone.0161443.
- Dhayni K, Zibara K, Issa H, et al. Targeting Cxcr1 and Cxcr2 receptors in cardiovascular diseases. Pharmacol Ther. 2022;237:108257. doi: 10.1016/j.pharmthera.2022.108257.
- Qureshi K, Abrams GA. Metabolic liver disease of obesity and role of adipose tissue in the pathogenesis of nonalcoholic fatty liver disease. World J Gastroenterol. 2007;13(26):3540–3553. doi: 10.3748/wjg.v13.i26.3540.
- Duan Y, Pan X, Luo J, et al. Association of inflammatory cytokines with non-alcoholic fatty liver disease. Front Immunol. 2022;13:880298. doi: 10.3389/fimmu.2022.880298.
- Lee EY, Lee ZH, Song YW. Cxcl10 and autoimmune diseases. Autoimmun Rev. 2009;8(5):379–383. doi: 10.1016/j.autrev.2008.12.002.
- Cannito S, Morello E, Bocca C, et al. Microvesicles released from fat-laden cells promote activation of hepatocellular Nlrp3 inflammasome: a pro-inflammatory link between lipotoxicity and non-alcoholic steatohepatitis. PLoS One. 2017;12(3):e0172575. doi: 10.1371/journal.pone.0172575.
- Guo Q, Furuta K, Lucien F, et al. Integrin beta1-enriched extracellular vesicles mediate monocyte adhesion and promote liver inflammation in murine nash. J Hepatol. 2019;71(6):1193–1205. doi: 10.1016/j.jhep.2019.07.019.
- Garcia-Martinez I, Santoro N, Chen Y, et al. Hepatocyte mitochondrial DNA drives nonalcoholic steatohepatitis by activation of Tlr9. J Clin Invest. 2016;126(3):859–864. doi: 10.1172/JCI83885.
- Hernández A, Geng Y, Sepúlveda R, et al. Chemical hypoxia induces pro-inflammatory signals in fat-laden hepatocytes and contributes to cellular crosstalk with kupffer cells through extracellular vesicles. Biochim Biophys Acta Mol Basis Dis. 2020;1866(6):165753. doi: 10.1016/j.bbadis.2020.165753.
- Hernández A, Reyes D, Geng Y, et al. Extracellular vesicles derived from fat-laden hepatocytes undergoing chemical hypoxia promote a pro-fibrotic phenotype in hepatic stellate cells. Biochim Biophys Acta Mol Basis Dis. 2020;1866(10):165857. doi: 10.1016/j.bbadis.2020.165857.
- Gim JA, Bang SM, Lee YS, et al. Evaluation of the severity of nonalcoholic fatty liver disease through analysis of serum exosomal mirna expression. PLoS One. 2021;16(8):e0255822. doi: 10.1371/journal.pone.0255822.
- López-Pastor AR, Infante-Menéndez J, González-Illanes T, et al. Concerted regulation of non-alcoholic fatty liver disease progression by microRNAs in apolipoprotein E-deficient mice. Dis Model Mech. 2021;14(12):dmm049173. doi: 10.1242/dmm.049173.
- Newman LA, Useckaite Z, Johnson J, et al. Selective isolation of liver-derived extracellular vesicles redefines performance of mirna biomarkers for non-alcoholic fatty liver disease. Biomedicines. 2022;10(1):195. doi: 10.3390/biomedicines10010195.
- Zhang JW, Pan HT. Microrna profiles of serum exosomes derived from children with nonalcoholic fatty liver. Genes Genomics. 2022;44(7):879–888. doi: 10.1007/s13258-021-01150-8.
- Jiang H, Qian Y, Shen Z, et al. Circulating microRNA135a3p in serum extracellular vesicles as a potential biological marker of nonalcoholic fatty liver disease. Mol Med Rep. 2021;24(1):498. doi: 10.3892/mmr.2021.12137.
- Cermelli S, Ruggieri A, Marrero JA, et al. Circulating microRNAs in patients with chronic hepatitis C and non-alcoholic fatty liver disease. PLoS One. 2011;6(8):e23937. doi: 10.1371/journal.pone.0023937.
- Pirola CJ, Gianotti TF, Castaño GO, et al. Circulating microRNA-122 signature in nonalcoholic fatty liver disease and cardiovascular disease: a new endocrine system in metabolic syndrome. Hepatology. 2013;57(6):2545–2547. doi: 10.1002/hep.26116.
- Csak T, Bala S, Lippai D, et al. MicroRNA-122 regulates hypoxia-inducible factor-1 and vimentin in hepatocytes and correlates with fibrosis in diet-induced steatohepatitis. Liver Int. 2015;35(2):532–541. doi: 10.1111/liv.12633.
- Liu XL, Pan Q, Cao HX, et al. Lipotoxic hepatocyte-derived exosomal microRNA 192-5p activates macrophages through rictor/AKT/forkhead box transcription factor O1 signaling in nonalcoholic fatty liver disease. Hepatology. 2020;72(2):454–469. doi: 10.1002/hep.31050.
- Liu H, Niu Q, Wang T, et al. Lipotoxic hepatocytes promote nonalcoholic fatty liver disease progression by delivering microRNA-9-5p and activating macrophages. Int J Biol Sci. 2021;17(14):3745–3759. doi: 10.7150/ijbs.57610.
- Koenen MT, Brandt EF, Kaczor DM, et al. Extracellular vesicles from steatotic hepatocytes provoke pro-fibrotic responses in cultured stellate cells. Biomolecules. 2022;12(5):698. doi: 10.3390/biom12050698.
- Povero D, Panera N, Eguchi A, et al. Lipid-induced hepatocyte-derived extracellular vesicles regulate hepatic stellate cell via microRNAs targeting PPAR-gamma. Cell Mol Gastroenterol Hepatol. 2015;1(6):646–663.e644. doi: 10.1016/j.jcmgh.2015.07.007.
- Lee YS, Kim SY, Ko E, et al. Exosomes derived from palmitic acid-treated hepatocytes induce fibrotic activation of hepatic stellate cells. Sci Rep. 2017;7(1):3710. doi: 10.1038/s41598-017-03389-2.
- Gao H, Jin Z, Bandyopadhyay G, et al. Mir-690 treatment causes decreased fibrosis and steatosis and restores specific kupffer cell functions in nash. Cell Metab. 2022;34(7):978–990.e974. doi: 10.1016/j.cmet.2022.05.008.
- Hou X, Yin S, Ren R, et al. Myeloid-cell-specific Il-6 signaling promotes microRNA-223-enriched exosome production to attenuate NAFLD-associated fibrosis. Hepatology. 2021;74(1):116–132. doi: 10.1002/hep.31658.
- He Y, Hwang S, Cai Y, et al. MicroRNA-223 ameliorates nonalcoholic steatohepatitis and cancer by targeting multiple inflammatory and oncogenic genes in hepatocytes. Hepatology. 2019;70(4):1150–1167. doi: 10.1002/hep.30645.
- He Y, Rodrigues RM, Wang X, et al. Neutrophil-to-hepatocyte communication via LDLR-dependent mir-223-enriched extracellular vesicle transfer ameliorates nonalcoholic steatohepatitis. J Clin Invest. 2021;131(3):e141513. doi: 10.1172/JCI141513.
- Calvente CJ, Tameda M, Johnson CD, et al. Neutrophils contribute to spontaneous resolution of liver inflammation and fibrosis via microRNA-223. J Clin Invest. 2019;129(10):4091–4109. doi: 10.1172/JCI122258.
- He Y, Feng D, Li M, et al. Hepatic mitochondrial DNA/toll-like receptor 9/microRNA-223 forms a negative feedback loop to limit neutrophil overactivation and acetaminophen hepatotoxicity in mice. Hepatology. 2017;66(1):220–234. doi: 10.1002/hep.29153.
- Duval C, Thissen U, Keshtkar S, et al. Adipose tissue dysfunction signals progression of hepatic steatosis towards nonalcoholic steatohepatitis in C57bl/6 mice. Diabetes. 2010;59(12):3181–3191. doi: 10.2337/db10-0224.
- Flaherty SE, 3rd, Grijalva A, Xu X, et al. A lipase-independent pathway of lipid release and immune modulation by adipocytes. Science. 2019;363(6430):989–993. doi: 10.1126/science.aaw2586.
- Castaño C, Kalko S, Novials A, et al. Obesity-associated exosomal miRNAs modulate glucose and lipid metabolism in mice. Proc Natl Acad Sci U S A. 2018;115(48):12158–12163. doi: 10.1073/pnas.1808855115.
- Gu H, Yang K, Shen Z, et al. Er stress-induced adipocytes secrete-aldo-keto reductase 1b7-containing exosomes that cause nonalcoholic steatohepatitis in mice. Free Radic Biol Med. 2021;163:220–233. doi: 10.1016/j.freeradbiomed.2020.12.011.
- Yan C, Tian X, Li J, et al. A high-fat diet attenuates AMPK Alpha1 in adipocytes to induce exosome shedding and nonalcoholic fatty liver development in vivo. Diabetes. 2021;70(2):577–588. doi: 10.2337/db20-0146.
- Larter CZ, Yeh MM, Van Rooyen DM, et al. Roles of adipose restriction and metabolic factors in progression of steatosis to steatohepatitis in obese, diabetic mice. J Gastroenterol Hepatol. 2009;24(10):1658–1668. doi: 10.1111/j.1440-1746.2009.05996.x.
- Li Y, Luan Y, Li J, et al. Exosomal mir-199a-5p promotes hepatic lipid accumulation by modulating Mst1 expression and fatty acid metabolism. Hepatol Int. 2020;14(6):1057–1074. doi: 10.1007/s12072-020-10096-0.
- Gu N, You L, Shi C, et al. Expression of mir-199a-3p in human adipocytes is regulated by free fatty acids and adipokines. Mol Med Rep. 2016;14(2):1180–1186. doi: 10.3892/mmr.2016.5379.
- Czech MP. Mechanisms of insulin resistance related to white, beige, and brown adipocytes. Mol Metab. 2020;34:27–42. doi: 10.1016/j.molmet.2019.12.014.
- Zhang X, Ji X, Wang Q, et al. New insight into inter-organ crosstalk contributing to the pathogenesis of non-alcoholic fatty liver disease (NAFLD). Protein Cell. 2018;9(2):164–177. doi: 10.1007/s13238-017-0436-0.
- Du Plessis J, Van Pelt J, Korf H, et al. Association of adipose tissue inflammation with histologic severity of nonalcoholic fatty liver disease. Gastroenterology. 2015;149(3):635–648.e614. doi: 10.1053/j.gastro.2015.05.044.
- Koeck ES, Iordanskaia T, Sevilla S, et al. Adipocyte exosomes induce transforming growth factor beta pathway dysregulation in hepatocytes: a novel paradigm for obesity-related liver disease. J Surg Res. 2014;192(2):268–275. doi: 10.1016/j.jss.2014.06.050.
- Ferrante SC, Nadler EP, Pillai DK, et al. Adipocyte-derived exosomal miRNAs: a novel mechanism for obesity-related disease. Pediatr Res. 2015;77(3):447–454. doi: 10.1038/pr.2014.202.
- Zhao J, Hu L, Gui W, et al. Hepatocyte TGF-beta signaling inhibiting wat browning to promote NAFLD and obesity is associated with let-7b-5p. Hepatol Commun. 2022;6(6):1301–1321. doi: 10.1002/hep4.1892.
- Shang Q, Bai Y, Wang G, et al. Delivery of adipose-derived stem cells attenuates adipose tissue inflammation and insulin resistance in obese mice through remodeling macrophage phenotypes. Stem Cells Dev. 2015;24(17):2052–2064. doi: 10.1089/scd.2014.0557.
- He Q, Wang L, Zhao R, et al. Mesenchymal stem cell-derived exosomes exert ameliorative effects in type 2 diabetes by improving hepatic glucose and lipid metabolism via enhancing autophagy. Stem Cell Res Ther. 2020;11(1):223. doi: 10.1186/s13287-020-01731-6.
- Hyvärinen K, Holopainen M, Skirdenko V, et al. Mesenchymal stromal cells and their extracellular vesicles enhance the anti-inflammatory phenotype of regulatory macrophages by downregulating the production of interleukin (Il)-23 and Il-22. Front Immunol. 2018;9:771. doi: 10.3389/fimmu.2018.00771.
- Bruno S, Pasquino C, Herrera Sanchez MB, et al. HLSC-derived extracellular vesicles attenuate liver fibrosis and inflammation in a murine model of non-alcoholic steatohepatitis. Mol Ther. 2020;28(2):479–489. doi: 10.1016/j.ymthe.2019.10.016.
- Cheng L, Yu P, Li F, et al. Human umbilical cord-derived mesenchymal stem cell-exosomal mir-627-5p ameliorates non-alcoholic fatty liver disease by repressing FTO expression. Hum Cell. 2021;34(6):1697–1708. doi: 10.1007/s13577-021-00593-1.
- Tan Y, Huang Y, Mei R, et al. Hucmsc-derived exosomes delivered Becn1 induces ferroptosis of hepatic stellate cells via regulating the Xct/Gpx4 axis. Cell Death Dis. 2022;13(4):319. doi: 10.1038/s41419-022-04764-2.
- El-Derany MO, Abdelhamid SG. Upregulation of mir-96-5p by bone marrow mesenchymal stem cells and their exosomes alleviate non-alcoholic steatohepatitis: emphasis on caspase-2 signaling inhibition. Biochem Pharmacol. 2021;190:114624. doi: 10.1016/j.bcp.2021.114624.
- Zhang Q, Liu X, Piao C, et al. Effect of conditioned medium from adipose derived mesenchymal stem cells on endoplasmic reticulum stress and lipid metabolism after hepatic ischemia reperfusion injury and hepatectomy in swine. Life Sci. 2022;289:120212. doi: 10.1016/j.lfs.2021.120212.
- Shuen TWH, Alunni-Fabbroni M, Öcal E, et al. Extracellular vesicles may predict response to radioembolization and sorafenib treatment in advanced hepatocellular carcinoma: an exploratory analysis from the soramic trial. Clin Cancer Res. 2022;28(17):3890–3901. doi: 10.1158/1078-0432.Ccr-22-0569.
- Zuo R, Ye LF, Huang Y, et al. Hepatic small extracellular vesicles promote microvascular endothelial hyperpermeability during NAFLD via novel-miRNA-7. J Nanobiotechnol. 2021;19(1):396. doi: 10.1186/s12951-021-01137-3.
- Jiang F, Chen Q, Wang W, et al. Hepatocyte-derived extracellular vesicles promote endothelial inflammation and atherogenesis via microRNA-1. J Hepatol. 2020;72(1):156–166. doi: 10.1016/j.jhep.2019.09.014.
- Sengupta V, Sengupta S, Lazo A, et al. Exosomes derived from bone marrow mesenchymal stem cells as treatment for severe covid-19. Stem Cells Dev. 2020;29(12):747–754. doi: 10.1089/scd.2020.0080.
- Fuhrmann G, Herrmann IK, Stevens MM. Cell-derived vesicles for drug therapy and diagnostics: opportunities and challenges. Nano Today. 2015;10(3):397–409. doi: 10.1016/j.nantod.2015.04.004.