ABSTRACT
The perovskite-type cubic oxides have found useful applications in catalysis, solid oxide fuel cells, gas sensors, and membrane technology. XPS and Raman studies of a recently developed (Ba0.5Sr0.5)(Al0.2-xMgxFe0.8)O3-ξ (x = 0–0.2) oxygen-permeable system are reported here. The effect of magnesium is shown to alter the relative amounts of Fe3+, Fe4+, and oxygen species via Fe (2p3/2 and 2p1/2) and O 1s signals. Besides, it causes a surge in oxygen vacancies, an increase in Fe3+ ions, a decline in B – O bond strength (B = Al/Mg/Fe), and a rise in anion flow. The O1s signals at ~ 528.5 and ~531.0 eV correspond to surface oxide and adsorbed (O22−, O2−, or O−) species, respectively. Raman spectra offer evidence for symmetric A1g stretching, oxygen vacancies, distorted BO6 octahedra, A-O stretching vibration modes (A = Ba, Sr), and bending B-O linkages. A good correlation is advanced between the XPS and Raman results, in conformity with lattice expansion, Mossbauer data, improved oxygen permeability, enhanced electrical conductivity, and high structural stability realized by means of partial replacement of Al3+ with Mg2+ in (Ba0.5Sr0.5)(Al0.2Fe0.8)O3-ξ earlier.
1. Introduction
The perovskite-type cubic oxide (ABO3; A = La, Ba, Sr; B = Co, Fe, Al, Mg; O-oxygen) based systems have generated immense interest due to their potential applications as gas separation membranes, solid electrolyte materials, and catalysts besides in fuel cells, sensors, etc [Citation1–10]. Their membranes possess a unique capacity of oxygen separation from the air and so play a vital role in industry and power generation [Citation10–13]. The flow of oxygen occurs due to the driving force brought by the pressure gradient across the membrane. The main advantage of the process lies in the continuous production of ~ 100% pure oxygen and cost-effectiveness. In contrast, cryogenic distillation and pressure swing adsorption methods yield oxygen of only 90–95% purity and involve large-scale plants with high operation cost [Citation10,Citation12,Citation13]. The practical issues in the effective utilization as ceramic membranes are however pertain to their structural stability, mechanical strength, and oxygen permeability at operating temperatures. Intensive efforts have been made in the past to develop multi-component solid-state membranes exhibiting high oxygen permeability with adequate structural stability at elevated temperatures. Some known oxygen-permeable system based on iron and cobalt are La1−xBxCo1−yFeyO3-ξ (B = Sr and/or Ba, Ca; x = 0–1; y = 0–1), Sr0.9Ca0.1Co1−yFeyO2.5+ξ (y = 0, 0.1), Gd0.2Ba0.8Co0.7Cu0.2Fe0.1O3-ξ, and Ba0.95Yb0.05Co0.7Fe0.3O3-ξ [Citation14–17]. But the cobalt-based membranes, though exhibit reasonable oxygen permeability, are expensive and show poor stability (thermal and mechanical) and high thermal expansion [Citation1,Citation10]. So, the attention was shifted to the iron-based compounds, viz., SrFeO3-ξ, Ba1−xSrxFeO3-ξ, La1−xSrxFeO3-ξ, Sr1−xBixFeO3-ξ, Sr0.97Ti1−xFexO3-ξ, (Ba0.5Sr0.5)(Zn0.2Fe0.8)O3-ξ, SrTi0.7Fe0.2Mg0.1O3-ξ, La0.6Sr0.4Ga0.6Fe0.4O3-ξ, (La, Sr) (Ga, Fe) O3-ξ, etc [Citation18–29]. In this series, the present authors developed a multi-component (Ba0.5Sr0.5)(Al0.2-xMgxFe0.8)O3-ξ (x = 0–0.2) system by partial replacement of Sr2+ with Ba2+ (at A-site) and Al3+ with Mg2+ (at B-site) in SrAl0.2Fe0.8O3-ξ and focused on its phase stability, oxygen permeability, and electrical conductivity [Citation30]. The work described here is in continuation and present some results of X-ray photoelectron spectroscopy and Raman studies of (Ba0.5Sr0.5)(Al0.2-xMgxFe0.8)O3-ξ (x = 0–0.2) to understand the effect of magnesium and possible correlation with earlier findings [Citation30].
2. Experimental
(Ba0.5Sr0.5)(Al0.2-xMgxFe0.8)O3-ξ (x = 0–0.2) powder samples were synthesized via sol-gel route comprising of mixing of the metal nitrates and oxalic acid solutions in ethanol, gel formation and drying at 150°C for 24 h, decomposition at 950°C for 5 h in air, and sieving [Citation30]. The synthesis procedure is given in the supplementary information. A Thermo-electron X-ray diffractometer (model ARL X/TRA) was employed for phase analysis. The molecular vibrational spectra having sensitivity to local structure and chemistry were obtained using a Raman microscope (Renishaw model Invia) at the excitation wavelength of 1064 nm (YAG laser); resolution being 0.3 cm−1 (FWHM). To explore the electronic states of species, the X-ray photoelectron spectra (sample area 100 μm x 100 μm) were recorded with a PHI 5000 VersaProbe (Model Ulvac) using an Al Kα (hυ = 1486.6 eV) at the pressure of ~ 6.7 × 10−8 Pa. The signal was gathered at the take-off angle of 45° to get a contribution from the sub-surface region. The high resolution (step 125 meV) spectra of species were obtained inappropriate energy regimes. Carbon 1s peak at 284.6 eV (with the precision of 0.1–0.2 eV) was used as a reference for deducing the binding energy (BE) accurately. The data accumulation time interval was fixed at 3 – 60 min and an Origin 8.5 software was utilized for fitting of each peak assuming the Gaussian/Lorentzian distribution(s).
3. Results and discussion
(Ba0.5Sr0.5)(Al0.2-xMgxFe0.8)O3-ξ (0 ≤ x ≤ 0.2) powder samples exhibit a perovskite-type cubic structure with lattice parameter 3.953–3.978 Å ± 0.002 Å (Fig S1 supplementary information). XPS data contain information about the oxidation state(s) of species as well as local charge transfer related effects in the material [Citation31]. The electronic/bonding states and local chemical environment around oxygen are examined through XPS studies to understand the anion vacancy formation with magnesium insertion (x ≤ 0.2) at Al3+ sites in (Ba0.5Sr0.5)(Al0.2-xMgxFe0.8) O3-ξ. The carbon C 1s peak at 284.6 eV is used as a standard for calibration of XPS data. X-ray photoelectron (XP) spectra of (Ba0.5Sr0.5) (Al0.2-xMgxFe0.8) O3-ξ (0 ≤ x ≤ 0.2) having contributions from Ba 3d, Sr 3d, Al 2p, Mg 1s, Fe 2p, and oxygen (O 1s) levels shown in appear nearly similar for all the powder samples. For instance, the Ba 3d doublet is invariably observed in the energy range of 775–800 eV with FWHM of ~ 1.5–1.7 eV ()). But the peaks shift slightly toward higher energy and show progressive broadening with magnesium insertion, i.e. from ~794.79 and 779.54 eV for x = 0 to ~ 794.92 and 779.67 eV for x = 0.20, indicative thereby increasing distortion. The presence of two peak maxima at ~ 779 and ~795 eV also gives the signature of the perovskite structure [Citation31,Citation32]. ) depicts the XPS scans of Ba 3d5/2 and Ba 3d3/2 peaks at respective binding energies (marked) in different compositions. The peak is deconvoluted into two with centers around 779 eV and 777 eV in the case of Ba 3d5/2. The exact position, width, and percentage area of each fitting peak for both the cases are summarized in . Notice that the (%) peak area decreases at ~779.5 eV but increases at ~ 777.5 eV with a rise in magnesium content. These correspond to barium lying on the surface and in sub-surface regions, respectively. The figures suggest depletion of barium at the surface with increased magnesium content. Further, the presence of both the peaks points toward relaxed states for Ba-species [Citation31–35]. The Sr 3d signal appears as a doublet due to spin-orbit coupling of 3d5/2 and 3d3/2 levels; their binding energies (BE) being ~ 133 and ~ 135 eV, respectively (). These peaks shift marginally toward lower energy with magnesium insertion, i.e. from 133.42 and 134.92 eV for x = 0 to 133.04 and 134.79 eV for x = 0.20. On examining closely, a hump is observed at the lower energy side (~ 131.67 eV) in the spectrum of composition x = 0.20. The above binding energy values match well with the Sr2+ ion data observed before in the perovskite-type cubic oxides [Citation33,Citation34,Citation36]. The Sr 3d and Ba 3d XPS data support XRD findings, which conform to occupancy of barium and strontium at A-sites in ABO3-δ cubic structure [Citation30]. depicts the XP spectra of (Ba0.5Sr0.5)(Al0.2-xMgxFe0.8)O3-ξ samples for aluminum (Al 2p) and magnesium (Mg 1s).
Figure 1. XPS survey spectra of (Ba0.5Sr0.5)(Al0.2-xMgxFe0.8)O3-ξ (x = 0, 0.05, 0.10, 0.20) powder samples indicating peaks belonging to different elements. Carbon 1s peak, taken as standard, is also shown
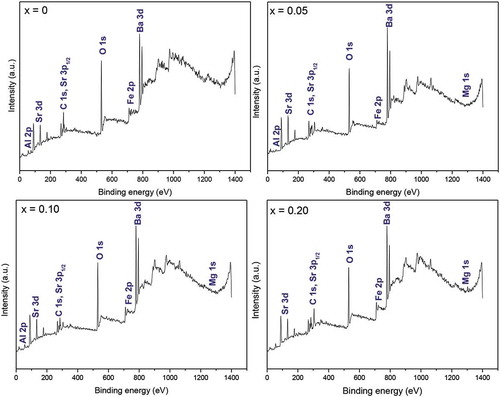
Figure 2. XPS spectra of (Ba0.5Sr0.5)(Al0.2-xMgxFe0.8)O3-ξ (x = 0, 0.05, 0.10, 0.15, 0.20) showing (a) Ba 3d3/2 and 3d5/2 levels and (b) their deconvoluted fitting curves for the composition (x) = 0.05, 0.10, 0.20
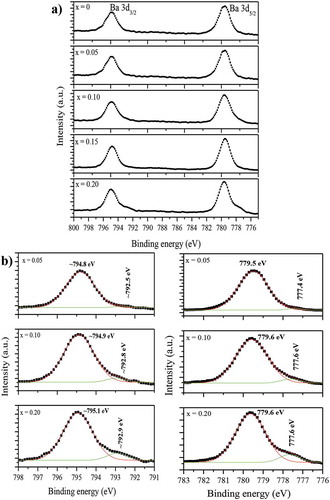
Figure 3. XPS spectra of (Ba0.5Sr0.5) (Al0.2-xMgxFe0.8) O3-ξ (x = 0–0.20) showing Sr 3d3/2 and Sr 3d5/2 levels
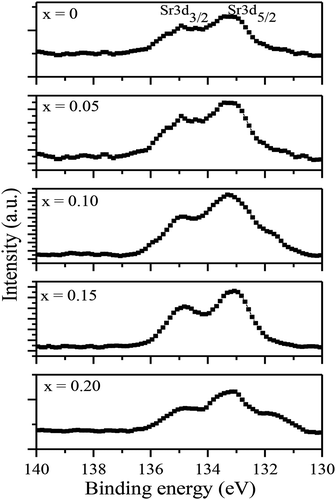
Figure 4. XPS Al 2p and Mg 1s spectra of (Ba0.5Sr0.5) (Al0.2-xMgxFe0.8) O3-ξ for the composition x = (0, 0.05, 0.10) and (0.05, 0.10, 0.20), respectively
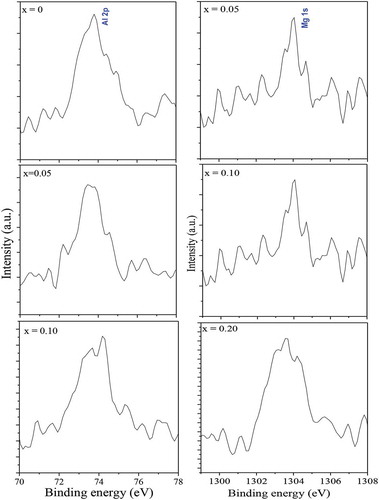
Table 1. XPS parameters of Ba 3d3/2 and Ba 3d5/2 levels in (Ba0.5Sr0.5) (Al0.2-xMgxFe0.8) O3-ξ (x = 0.05, 0.10, 0.20)
The XP spectra of Fe 2p level in (Ba0.5Sr0.5)(Al0.2-xMgxFe0.8)O3-ξ samples are shown in . The spin-orbit splitting gives rise to doublets for 2p3/2 and 2p1/2 levels. Their Gaussian fitting have four peaks. While the main ones at ~ 710.2 and ~ 712.8 eV correspond to Fe3+ (2p3/2) and Fe4+ (2p3/2), respectively, others at ~723.3 and ~725.6 eV resemble with Fe3+ (2p1/2) and Fe4+ (2p1/2), respectively. Thus, iron ions exist in two oxidation states (namely, 3+ and 4+) in (Ba0.5Sr0.5)(Al0.2-xMgxFe0.8)O3-ξ (x = 0–0.2) samples. Their origin lies with electrostatic interaction, spin-orbit coupling between 2p core hole and unpaired 3d electron of photoionized cation, and crystal field effect [Citation37–40]. The relative amounts of Fe3+ and Fe4+ ions are deduced from respective areas of the fitting curves and summarized in . Clearly, there is decrease of Fe4+ ions and increase of Fe3+ species with rise in magnesium content (x), both occupying B-sites in ABO3-δ structure. It essentially means that magnesium substitution induces some Fe4+ → Fe3+ conversion with added anion vacancies and consumption of electron released by oxygen desorption as molecule. These findings are consistent and in agreement with the Mössabuer data, revealing upsurge of Fe3+ ion concentration in (Ba0.5Sr0.5)(Al0.2-xMgxFe0.8)O3-ξ (0 ≤ x ≤ 0.2) [Citation30].
Figure 5. Typical XPS spectra (open circles) and fitted curves of (Ba0.5Sr0.5)(Al0.2-xMgxFe0.8)O3-ξ (x = 0.10, 0.15, 0.20) compounds showing individual contributions of Fe3+ and Fe4+ species
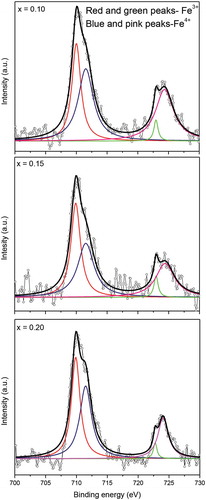
Table 2. XPS peak parameters of Fe 2p level in (Ba0.5Sr0.5)(Al0.2-xMgxFe0.8)O3-ξ (x = 0.05, 0.10, 0.15, 0.20)
The high-resolution XP spectrum of O 1s level presented in is fitted with two Gaussian peaks at ~ 528.5 and ~ 531.0 eV (say, for x = 0), ascribed to surface oxide, and adsorbed (O22−, O2−, or O−) species, respectively () [Citation41]. The lower binding energy (LBE) peak at 528.5 eV with FWHM of ~ 1.4 eV corresponds to B – O linkages (B = Fe/Mg/Al), whereas the higher binding energy (HBE) signal at ~ 531 eV is attributed to A – O bonds (A = Ba, Sr) associated with adsorbed oxygen [Citation31,Citation36,Citation41–45]. The growth of LBE peak area suggests increase in B – O linkages at the surface with rise in magnesium content. A weak B-O bond is expected to release extra oxygen, increasing thereby the anion vacancies. This scenario provides more open space in the structure and facilitates the anion movement – leading to higher ionic conductivity, as found earlier [Citation30,Citation31,Citation41–45].
Figure 6. XPS spectra of O1s for (Ba0.5Sr0.5)(Al0.2-xMgxFe0.8)O3-ξ (x = 0, 0.05, 0.10, 0.15, 0.20) with Gaussian fitting using two peaks in each case. The peaks with higher and lower binding energies are attributed to adsorbed oxygen species and surface oxide, respectively
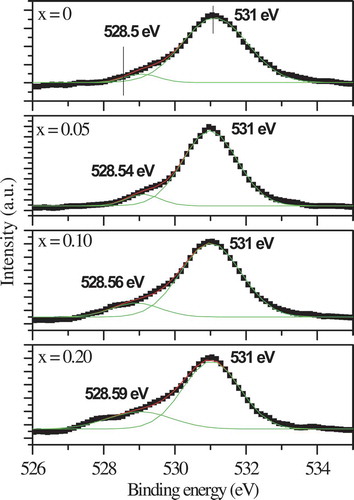
Table 3. XPS parameters of O1s in (Ba0.5Sr0.5)(Al0.2-xMgxFe0.8)O3-ξ (x = 0–0.20)
Structural symmetry of oxides can be evaluated by Raman spectroscopy because of its sensitivity to local chemistry and coordination [Citation46]. ) displays a few typical Raman spectra of (Ba0.5Sr0.5)(Al0.2-xMgxFe0.8)O3-ξ (x = 0–0.20) powder samples in the wavenumber range of 300–900 cm−1. Interestingly, the main peak having Raman shift (> 600 cm−1) moves toward higher wavenumber with increasing magnesium content (x); notice that the Mg2+ ion is lighter and has less positive charge than Al3+. Also, a shoulder in the Raman band observed in the sample of composition x = 0.20 indicates splitting of the signal into two peaks centered around 540 and 680 cm−1 ()). A band with Raman shift in the range of 600–700 cm−1 corresponds to a totally symmetric A1g stretching mode of BO6 self-distorted octahedra (or in-phase stretching breathing mode of oxygen in close vicinity of B-ion). The band near 680 cm−1 provides evidence for oxygen vacancies too [Citation46,Citation47]. These characteristics possibly arise due to local distortion of the crystal symmetry [Citation32,Citation48]. Generally, the anti-stretching (Eg) mode is two-fold (Eg1 and Eg2) whereas that of bending F2gi is three-fold (i = 1,2,3) degenerate and represent octahedra tilting as well as Ba2+/Sr2+ translation. Both Eg and F2g modes exhibit Raman shift in the range of 500–600 cm−1. Thus, the split peak at 540 cm−1 arises due to octahedral tilting and/or Sr/Ba-ion translation induced by the creation of extra anion vacancies in the vicinity. The orthorhombic distortion of BO6 octahedra displays a total of 24 Raman active modes involving rotation, anti-stretching, bending, and stretching effects [Citation49–51]. The oxygen vibrates along M-O-M’ bonds, where M and M’ stand for metal ions of different size and oxidation states. The relative size of M and M’ ions (Mg2+, Al3+, Fe3+, Fe4+) alters the stiffness of bonds and hence the frequency of vibration. Also, the occupation of Mg2+ ion at Al3+ site in (Ba0.5Sr0.5)(Al0.2-xMgxFe0.8)O3-ξ affects oxygen vacancies in the neighborhood and, in turn, modifies the local symmetry. As a result, some variation in the B-O stretching occurs, leading to different vibration modes. This change is revealed by peak shifting and splitting. It further suggests that Mg2+ addition brings in the distortion of octahedra as well as enhancement in the oxygen vacancies (or non-stoichiometry parameter, ξ). With increase occurring in lattice parameter and anion vacancy concentration in (Ba0.5Sr0.5)(Al0.2-xMgxFe0.8)O3-ξ with rise in magnesium content (x), the perovskite-type cubic structure becomes more open, facilitates charge carrier movement, and leads to a high ionic conductivity. The weak band observed with Raman shift of 405 cm−1 corresponds to internal F2g (or ν5) mode caused by bending of B – O linkages (B = Mg, Al, Fe) belonging to the distorted BO6 octahedra. The corresponding Raman shift usually lies in the range of 305–420 cm−1 depending on the nature of B – ions [Citation52,Citation53]. Generally, A-O (A = Ba, Sr) linkage modes fall in the low-frequency range (80–281 cm−1). But peaks which appear below 200 cm−1 only are attributed to stretching vibrations [Citation32,Citation47,Citation54]. Notice that the bands observed in the composition of x = 0.20 correspond to Raman shift of ~ 122, 165, 405, 540, and 680 cm− 1. These can be associated with A-O stretching vibrations (122, 165 cm−1), bending of B-O linkages (405 cm−1), and BO6 symmetric A1g stretching, octahedra tilting and/or A-O translation, and oxygen vacancies (540, 680 cm−1).
Figure 7. (a) Room temperature Raman spectra of (Ba0.5Sr0.5)(Al0.2-xMgxFe0.8)O3-ξ (x = 0, 0.10, 0.15, 0.20) in the wave number range of 110–900 cm−1 and (b) deconvoluted fitting curves covering span of 500–850 cm−1 (associated with oxygen vacancies) having peaks at 540 and 680 cm−1 for the composition x = 0.20
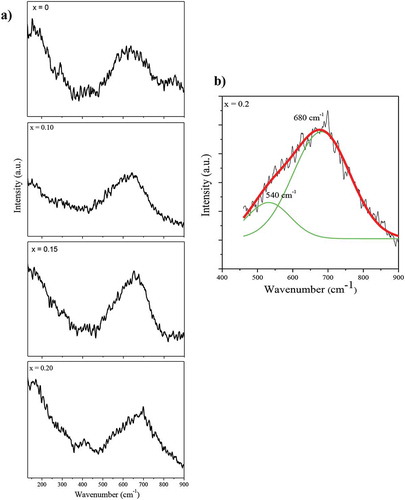
Magnesium in (Ba0.5Sr0.5)(Al0.2-xMgxFe0.8)O3-ξ (0 ≤ x ≤ 0.2) compounds (a) expands the ABO3 cubic cell (a = 3.953–3.978 Å) due to its size being larger than the substituted Al3+ (rMg2+ = 0.72 Å, rAl3+ = 0.585 Å), (b) creates additional anion vacancies with oxygen desorption as O2- → ½ O2 + e−, (c) induces Fe4+ → Fe3+ conversion following Fe4+ + e−→ Fe3+, (d) increases the electrical conductivity, and (e) weakens the metal-oxygen bond. These characteristics are consistent and well supported by XPS data and Raman spectra discussed above, evident in Mossbauer studies reported before [Citation30], and responsible for improving the oxygen permeability (JO2) of the membrane (thickness ~ 1 mm) to ~ 4.05–4.51 ml/cm2.min at 1000 °C (measurement details given briefly in the supplementary information). The process involves (i) a reaction O2 (Air) + 4e− → 2O2- at preferential sites on the front surface, (ii) migration of O2- ions via anion vacancies and due to pressure difference across the membrane, and (iii) liberation of oxygen as a molecule with release of associated electrons at the exit surface. The electrons then travel back to the front surface as such or by hopping via Fe3+- O – Fe4+ framework for continuation/recycle of the process. The enhancement in (JO2) with Mg2+ content results due to enlarged unit cell, increased oxygen vacancies, and improved electrical conductivity. Further, the (Ba0.5Sr0.5)(Fe0.8Mg0.20)O3-ξ membrane retains its original cubic phase and sustains high oxygen permeability at 900 °C for 120 h continuously. The stability (structural as well as mechanical) of membranes at elevated temperatures can therefore be attributed to magnesium in (Ba0.5Sr0.5)(Al0.2-xMgxFe0.8)O3-ξ.
4. Conclusions
Magnesium in (Ba0.5Sr0.5) (Al0.2-xMgxFe0.8) O3-ξ (x = 0–0.2) brings distortion in oxygen octahedra, creates additional oxygen vacancies, weakens the B-O (B = Al, Mg, Fe) bonds in perovskite ABO3-ξ cubic structure, and induces some Fe4+ → Fe 3+ conversion. These features accompanied by unit cell enlargement provide phase stability and significant improvement in the oxygen permeation in the system.
Supplemental Material
Download MS Word (448.5 KB)Disclosure statement
No potential conflict of interest was reported by the authors.
Supplementary material
Supplemental data for this article can be accessed here.
Additional information
Funding
References
- Sunarso J, Baumann S, Serra JM, et al. Mixed ionic–electronic conducting (MIEC) ceramic-based membranes for oxygen separation. J Membr Sci. 2008;320(1–2):13–41. .
- Hansen KK. Electrochemical reduction of oxygen and nitric oxide at low temperature on La1−xSrxCoO3−δ cathodes. J Electrochem Soc. 2010;157(9):P79–P82.
- Jin C, Liu J. Preparation of Ba1.2Sr0.8CoO4+δ K2NiF4-type structure oxide and cathodic behavioral of Ba1.2Sr0.8CoO4+δ–GDC composite cathode for intermediate temperature solid oxide fuel cells. J Alloys Compd. 2009;474(1–2):573–577.
- Wei X, Wei T, Li J, et al. Strontium cobaltite coated optical sensors for high temperature carbon dioxide detection. Sensors Actuat B. 2010;144:260–266.
- Kharton VV, Yaremchenko AA, Kovalevsky AV, et al. Perovskite-type oxides for high-temperature oxygen separation membranes. J Membr Sci. 1999;163(2):307–317. .
- Bouwmeester HJM, Burggraaf AJ. Dense ceramic membranes for oxygen separation. Amsterdam: Elsevier; 1996. Cot L, ed; p. 435–528.
- Fujishiro F, Kadota D, Hashimoto T. Relationship between the arrangement of oxide ion vacancies and oxide ion conduction in Ba(Fe0.9In0.1)2O5+d. J Am Ceram Soc. 2016;99:1866–1869.
- Lofberg A, Boujmini S, Capoen E, et al. Oxygen permeation versus catalytic properties of bismuth-based oxide ion conductors used for propene oxidation in a catalytic dense membrane reactor. Catal Today. 2004;91:79–83.
- Jaiswal SK, Kashyap VK, Kumar J. On the sol-gel synthesis and characterization of oxygen permeable strontium ferrite ceramic material. Mater Res Bull. 2012;47:692–699.
- Li K. Ceramic membrane for separation and reaction. England: John Wiley& Sons Ltd.; 2007.
- Hashim SS, Mohamed AR, Bhatia S. Oxygen separation from air using ceramic-based membrane technology for sustainable fuel production and power generation. Renewable Sustainable Energy Rev. 2011;15:1284–1293.
- Jasra RV, Choudary NV, Baht SGT. Separation of gases by pressure swin. Sep Sci Technol. 1991;16(7):885–930.
- Hashim SS, Mohamed AR, Bhatia S. Current status of ceramic-based membranes for oxygen separation from air. Adv Colloid Interface Sci. 2011;160:88–100.
- Vitoriano NO, Larramendi IR, Larramendi JR, et al. Synthesis and electrochemical performance of La0.6Ca0.4Fe1−xNixO3 (x = 0.1, 0.2, 0.3) material for solid oxide fuel cell cathode. J Power Sources. 2009;192:63–69.
- Nagai T, Itoh W, Sakon T. Relationship between cation substitution and stability of perovskite structure in SrCoO3−δ-based mixed conductors. Solid State Ion. 2007;177:3433–3444.
- Zeng P, Ran R, Chen Z, et al. Novel mixed conducting SrSc0.05Co0.95O3−δ ceramic membrane for oxygen separation. AIChE J. 2007;53:3116–3124.
- Miura N, Okamoto Y, Tamaki J, et al. Oxygen semipermeability of mixed-conductive oxide thick-film prepared by slip casting. Solid State Ion. 1995;79:195–200.
- Li D, Liu W, Zhang H, et al. Fabrication, microstructure, mechanical strength and oxygen permeation of Ba(Sr)Zr(CoFe)O3−δ particles-dispersed Ba0.5Sr0.5Co0.8Fe0.2O3−δ mixed-conducting composites. Mater Lett. 2004;58:1561–1564.
- Wang H, Tablet C, Feldhoff A, et al. A cobalt-free oxygen-permeable membrane based on the perovskite-type oxide Ba0.5Sr0.5Zn0.2Fe0.8O3−δ. Adv Mater. 2005;17:1785–1788.
- Park JH, Kim KY, Park SD. Oxygen permeation and stability of La0.6Sr0.4TixFe1-xO3-d (x = 0.2 and 0.3) membrane. Desalination. 2009;245:559–569.
- Kharton VV, Viskup AP, Kovalevsky AV, et al. Surface-limited ionic transport in perovskites Sr0.97(Ti,Fe,Mg)O3–δ. J Mater Chem. 2000;10:1161–1169.
- Lu Y, Zha H, Cheng X, et al. Investigation of In-doped BaFeO3-d perovskite type oxygen permeable membranes. J Mater Chem A. 2015;3:6202–6214.
- Teraoka Y, Shimokawa T, Kang CY, et al. Fe-based perovskite-type oxides as excellent oxygen-permeable and reduction-tolerant materials. Solid State Ion. 2006;177:2245–2248.
- Kashyap VK, Jaiswal SK, Kumar J. On the structural stability and oxygen permeation behavior of inorganic SrCo0.8Fe0.2O3−δ membranes. Ionics. 2016;22:2471–2485.
- Chen D, Chen C, Dong F, et al. Cobalt-free polycrystalline Ba0.95La0.05FeO3-d thin films as cathodes for intermediate-temperature solid oxide fuel cells. J Power Sources. 2014;250:188–195.
- Kharton VV, Viskup AP, Naumovich EN, et al. Oxygen ion transport in La2NiO4-based ceramics. J Mater Chem. 1999;9:2623–2629.
- Ishihara T, Nakashima K, Okada S, et al. Defect chemistry and oxygen permeation property of Pr2Ni0.75Cu0.25O4 oxide doped with Ga. Solid State Ion. 2008;179:1367.
- Czyperek M, Zapp P, Bouwmeester HJM, et al. Gas separation membranes for zero-emission fossil power plants: MEM-BRAIN. J Membr Sci. 2010;359:149–159.
- Jaiswal SK, Kumar J. Oxygen permeation characteristics of sol-gel derived barium substituted strontium ferrite membranes. J Am Ceram Soc. 2017;100:1306–1312.
- Jaiswal SK, Kumar J. A novel series of Ba0.5Sr0.5Al0.2−xMgxFe0.8O3−ξ (x ≤ 0.2) membranes for oxygen permeation application. J European Ceram Soc. 2014;34:381–390. .
- Amsif M, Marrero-Lopez D, Ruiz-Morales JC, et al. Influence of rare-earth doping on the microstructure and conductivity of BaCe0.9Ln0.1O3-d proton conductors. J Power Sources. 2011;196:3461–3469.
- Kosacki I, Schoonman J, Balkanski M. Raman scattering and ionic transport in SrCe1−xYbxO3. Solid State Ion. 1992;57:345–351.
- Liu B, Zhang Y, Tang L. X-ray photoelectron spectroscopic studies of Ba0.5Sr0.5Co0.8Fe0.2 O3-d cathode for solid oxide fuel cells. Int J Hydrogen Energy. 2009;34:435–439.
- Vander Heide PAW. Systematic X-ray photoelectron spectroscopic study of La1-xSrx-based perovskite-type oxides. Surf Interface Anal. 2002;33:414–425.
- Mukhopadhyay MS, Chen Tim CS. Surface chemical states of barium titanate: influence of sample processing. J Mater Res. 1995;10:1502–1507.
- Lee SO, Lee D, Jung I, et al. Ceria interlayer-free Ba0.5Sr0.5Co0.8Fe0.2O3-d-Sc0.1Zr0.9O1.95 composite cathode on zirconia based electrolyte for intermediate temperature solid oxide fuel cells. Int J Hydrogen Energy. 2013;38:9320–9329.
- Hwang HY, Cheong SW, Radaelli PG, et al. Lattice Effects on the Magnetoresistance in Doped LaMnO3. Phys Rev Lett. 1995;75:914.
- Atanelov J, Mohn P. Electronic and magnetic properties of GaFeO3: ab initio calculations for varying Fe/Ga ratio, inner cationic site disorder, and epitaxial strain. Phys Rev B. 2015;92:104408.
- Hatnean M, Robert J, Fernandez Diaz MT, et al. Neutron scattering study of the magnetoelectric compound GaFeO3. The European Physical J Special Topics. 2012;213:69–76.
- Sun ZH, Dai S, Zhou YL, et al. Elaboration and optical properties of GaFeO3 thin films. Thin Solid Films. 2008;516:7433–7436.
- Machocki A, Ioannides T, Stasinska B, et al. Manganese–lanthanum oxides modified with silver for the catalytic combustion of methane. J Catal. 2004;227:282–296.
- Jaiswal SK, Hong J, Yoon KJ, et al. Optical absorption and XPS studies of (Ba1-xSrx)(Ce0.75Zr0.10Y0.15)O3-d electrolytes for protonic ceramic fuel cells. Ceram Int. 2016;42:10366–10372.
- Beche E, Peraudeau G, Flaud V, et al. An XPS investigation of (La2O3)1-x(CeO2)2x(ZrO2)2 compounds. Surf Interface Anal. 2012;44:1045–1050.
- Anandan C, Bera P. XPS studies on the interaction of CeO2 with silicon in magnetron sputtered CeO2 thin films on Si and Si3N4 substrates. Appl Surf Sci. 2013;283:297–303.
- Jaiswal SK, Hong J, Yoon KJ, et al. Synthesis and conductivity behaviour of proton conducting (1-x)Ba0.6Sr0.4Ce0.75Zr0.10Y0.15O3-δ-xGDC (x = 0, 0.2, 0.5) composite electrolytes. J Am Ceramic Soc. 2017;100:4710–4718.
- Kosacki I, Tuller HL. Mixed conductivity in SrCe0.95Yb0.05O3 protonic conductors. Solid State Ion. 1995;80:223–229.
- Ricote S, Bonanos N, Lucas M, et al. Structural and conductivity study of the proton conductor BaCe(0.9−x)ZrxY0.1O(3−δ) at intermediate temperatures. J Power Sources. 2009;193:189–193.
- Ganguly M, Rout SK, Sinha TP, et al. Characterization and rietveld refinement of A-site deficient lanthanum doped barium titanate. J Alloys Compd. 2013;579:473–484.
- Bull CL, McMillan PF. Raman scattering study and electrical properties characterization of elpasolite perovskites Ln2(BB′)O6(Ln=La, Sm … Gd and B,B′=Ni, Co, Mn). J Solid State Chem. 2004;177:2323–2328.
- Iliev MN, Abrashev MV, Lee HG, et al. Raman spectroscopy of orthorhombic perovskite like YMnO3 and LaMnO3. Phys Rev B. 1998;57:2872–2882.
- Kreisel J, Glazer AM, Jones G, et al. An X-ray diffraction and Raman spectroscopy investigation of A-site substituted perovskite compounds: the (Na1-xKx)0.5Bi0.5TiO3 solid solution. J Phys Cond Matter. 2000;12:3267–3280.
- Gu YJ, Liu ZG, Ouyang JH, et al. Structure and electrical conductivity of BaCe0.85Ln0.15O3−δ (Ln=Gd, Y, Yb) ceramics. Elec Acta. 2013;105:547–553.
- Zhang C, Zhao H. Influence of In content on the electrical conduction behavior of Sm- and In-co-doped proton conductor BaCe0.80-xSm0.20InxO3-δ. Solid State Ion. 2012;206:17–21.
- Seeharaj P, Boonchom B, Charoonsuk P, et al. Barium zirconate titanate nanoparticles synthesized by the sonochemical method. Ceram Int. 2013;39:S559–S562.