Abstract
Although para-aminosalicylic acid (PAS) has been used to treat tuberculosis for decades, mechanisms of resistance to this drug in Mycobacterium tuberculosis (M. tuberculosis) clinical isolates have not been thoroughly investigated. Previously, we found that decreased methylenetetrahydrofolate reductase (MTHFR) activity of Rv2172c led to increased sensitivity to antifolates in M. tuberculosis. In this study, we collected the genome-sequencing data of 173 PAS-resistant and 803 PAS-sensitive clinical isolates and analyzed rv2172c mutations in those 976 isolates. The results showed that two mutations (T120P and M172 V) on rv2172c could be identified in a certain proportion (6.36%) of PAS-resistant isolates. The results of AlphaFold2 prediction indicated that the T120P or M172 V mutation might affect the enzymatic activity of Rv2172c by influencing nicotinamide adenine dinucleotide (NADH) binding, and this was verified by subsequent biochemical analysis, demonstrating the role of residues Thr120 and Met172 on NADH binding and enzymatic activity of Rv2172c. In addition, the effect of rv2172c T120P or M172 V mutation on methionine production and PAS resistance was determined in M. tuberculosis. The results showed that both T120P and M172 V mutations caused increased intracellular methionine concentrations and high level PAS resistance. In summary, we discovered new molecular markers and also a novel mechanism of PAS resistance in M. tuberculosis clinical isolates and broadened the understanding of the NADH-dependent MTHFR catalytic mechanism of Rv2172c in M. tuberculosis, which will facilitate the molecular diagnosis of PAS resistance and also the development of new drugs targeting Rv2172c.
Disclaimer
As a service to authors and researchers we are providing this version of an accepted manuscript (AM). Copyediting, typesetting, and review of the resulting proofs will be undertaken on this manuscript before final publication of the Version of Record (VoR). During production and pre-press, errors may be discovered which could affect the content, and all legal disclaimers that apply to the journal relate to these versions also.Introduction
Tuberculosis (TB), caused by Mycobacterium tuberculosis (M. tuberculosis), is still the leading infectious killer in the world [1], responsible for over 1 million deaths annually. Drug resistance casts a shadow over the treatment of TB. Namely, according to the latest global TB report [2], the treatment success rate for rifampicin-resistant/multidrug-resistant TB is only 63.82%, while the mortality rate is 13.13%. To fundamentally solve the dilemma of treatment, it is crucial to develop new antituberculosis drugs. Meanwhile, further examining the mechanisms of and identifying molecular markers for drug resistance remains necessary in order to promote the rational use of existing drugs to reduce the occurrence of adverse treatment outcomes.
One of the earliest antituberculosis drugs used in clinical practice [3], para-aminosalicylic acid (PAS) works as a prodrug, which is sequentially activated by the bacterial dihydropteroate synthase (DHPS) FolP1 and dihydrofolate synthase (DHFS) FolC, finally targeting the bacterial dihydrofolate reductase (DHFR) DfrA and blocking the bacterial tetrahydrofolate (THF) synthesis () [4]. At present, PAS is still used as a second-line antituberculosis drug for the treatment of drug-resistant TB. Previous studies have shown that mutations on thyA, folC, and ribD are the most commonly identified mutations in PAS-resistant (PAS-R) clinical isolates. [5-9]. Over two-thirds of PAS-R M. tuberculosis clinical isolates harbor mutations in these three genes, and the corresponding mechanisms have been revealed. FolC and ThyA are located in folate synthesis and metabolism pathways, respectively (). Binding-pocket variants of FolC fail to activate H2PtePAS to H2PtePAS-Glu, thereby hindering the activation of PAS [5]. Functional deficiency of ThyA increases the intracellular concentration of THF, which competes with H2PtePAS for FolC catalysis, thereby also hindering the activation of PAS and finally causing PAS resistance in M. tuberculosis [10]. Except for thyA and folC, a certain percentage (5%-9% in different studies) of PAS-R clinical isolates harbors the G-12A mutation upstream of the ribD gene [8,9], which causes overexpression of ribD [11]. M. tuberculosis RibD has been shown to have weak DHFR activity [12]. When overexpressed, RibD could be used as an alternative DHFR [11]. Very recently, mutations on the promoter region of thyX and MetM were also shown to cause PAS resistance in M. tuberculosis [13,14]. Overexpression of the methionine transporter MetM promotes uptake of exogenous methionine, which in turn increases the intracellular methionine level and finally causes PAS resistance [14].
Figure 1 The folate synthesis and metabolic pathways in M. tuberculosis and the target of para-aminosalicylic acid (PAS). MTHFR, 5,10-methylenetetrahydrofolate reductase; DHPS, dihydropteroate synthase; DHFS, dihydrofolate synthase; DHFR, dihydrofolate reductase; TS, thymidylate synthase; MetE, cobalamin-independent homocysteine transmethylase; MetH, cobalamin-dependent methionine synthase; HSMT, serine hydroxymethyltransferase.
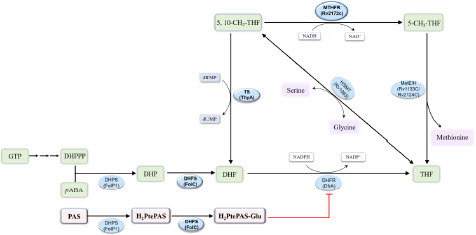
Methylenetetrahydrofolate reductase (MTHFR) catalyzes the reduction from 5, 10-methylenetetrahydrofolate (5, 10-CH2-THF) to 5-methyltetrahydrofolate (5-CH3-THF) [15], and this reaction is the sole source of bacterial 5-CH3-THF [16], which is required for the synthesis of methionine and the homeostasis of THF () [17-19]. In the early studies, MTHFRs found in prokaryotes (such as Escherichia coli and Thermus thermophilus) and eukaryotes (such as Saccharomyces cerevisiae and Homo sapiens) were both NAD(P)H-dependent flavoenzymes, which bound flavin adenine dinucleotide (FAD) as a source of reducing equivalents [20-22]. Until the last few years, MSMEG_6596/MSMEG_6649 and Rv2172c were characterized to be MTHFR in Mycobacterium smegmatis (M. smegmatis) and M. tuberculosis, respectively, and their enzymatic functions were experimentally proven to be FAD-independent [19,23]. It has been gradually recognized that mycobacterium encodes a special class of MTHFR solely depending on nicotinamide adenine dinucleotide (NADH). Very recently, Lin et al. solved the crystal structures of apo MSMEG_6649 and its complex with NADH [24], providing the foundation for understanding the potential catalytic mechanism of MTHFR in M. smegmatis. There are two MTHFRs in M. smegmatis, and even the major one (MSMEG_6596) is dispensable for the in vitro growth of the bacterium [23]. However, Rv2172c is the sole MTHFR in M. tuberculosis, which is essential for the in vitro growth of the bacterium [19]. Thus, Rv2172c is an ideal target for the development of antituberculosis drugs, and it is important to reveal the catalytic mechanism of Rv2172c.
Previously, we found that the L214A or R159N mutation on rv2172c resulted in decreased MTHFR activity of Rv2172c and then led to increased sensitivity to PAS in M. tuberculosis [19]. However, two rv2172c mutations (T120P and M172 V) seemed to be enriched in PAS-R clinical isolates. To virtually establish the association between the rv2172c T120P and M172 V mutations and PAS resistance, we collected the genome-sequencing data of 173 PAS-R and 803 PAS-sensitive (PAS-S) clinical isolates of M. tuberculosis from public databases [19,25-34]. We analyzed rv2172c mutations in these 976 genomes of the PAS-R/S isolates and also in 7061 genomes downloaded from the National Center for Biotechnology Information (NCBI) without drug susceptibility test (DST) profile. Then, we used AlphaFold2 to model the structure of Rv2172c, and the effect of T120P or M172 V mutation on NADH binding and enzymatic activity of Rv2172c was predicted. Subsequently, mutant proteins were purified, and the effect of T120P or M172 V mutation on the enzymatic activity of Rv2172c was analyzed in vitro. Furthermore, the effect of rv2172c T120P or M172 V mutation on methionine production and PAS resistance was determined in M. tuberculosis.
Materials and methods
Genome-sequencing data analysis
A library consisting of 7061 M. tuberculosis genomes was downloaded from the NCBI (https://www.ncbi.nlm.nih.gov/genome/browse#!/prokaryotes/mycobacterium%20tuberculosis, accessed on December 7, 2022). The acquired reads were subjected to quality assessment using FastQC v.0.11.9. Low-quality sequences were removed and trimmed using fastp as previously reported [35]. Reads shorter than 50 bp were discarded, the last 10 bp were trimmed, and bases with an average quality below 25 were removed using a sliding window of 20 bp. Then, analysis of single-nucleotide polymorphisms (SNPs) calling against the genome of M. tuberculosis H37Rv (NC_000962.3) was performed using the Snippy pipeline (Snippy. Available online: https://github.com/tseemann/snippy). Finally, mutations on the thyA, folC, or rv2172c genes were output, as well as the mutations on the 200 bp upstream ribD gene (Table S1). Thr202Ala in thyA as a marker for the Latin American Mediterranean lineage of the M. tuberculosis complex was excluded [36].
As shown in Table S2, a total of 976 genomes of M. tuberculosis clinical isolates with DST for PAS were obtained from previous studies [19,25-34]. Among these isolates, 173 strains were resistant to PAS, while 803 isolates were PAS-sensitive. Using the same method, we analyzed the ribD G-12A mutation or SNPs on thyA, folC, and rv2172c genes in these 976 isolates. Fragment insertions/deletions (ins/del) on the coding region of thyA, folC, or rv2172c were identified by Integrative genomics viewer (IGV v2.4.13). The results are shown in Table S2.
Bacterial strains, plasmids, and culture conditions
1. M. tuberculosis H37Ra and derivative strains were cultured at 37°C in 7H9 broth (Difco) supplemented with 10% (v/v) oleic acid–albumin–dextrose–catalase (OADC, Difco), 0.5% (v/v) glycerol, and 0.05% (v/v) Tween 80 (Sigma-Aldrich), or on 7H10 agar medium (Difco) supplemented with 10% (v/v) OADC (Difco) and 0.5% (v/v) glycerol. E. coli ArcticExpress (DE3) and derivative strains were cultured in Luria-Bertani (LB) medium (Difco), or on LB agar plates at 37°C. A total of 25 μg/mL and 100 μg/mL kanamycin (MedChemExpress, Inc.), 100 μg/mL ampicillin (MedChemExpress, Inc.), and 40 μg/mL gentamicin (MedChemExpress, Inc.) were used, unless otherwise indicated. We obtained 5, 10-CH2-THF from Schircks Laboratories. NADH was purchased from Coolaber. PAS (MedChemExpress, Inc.) was used at indicated concentrations. All bacteria strains, plasmids, and primers used in this study are described in detail in Table S3.
Genetic manipulation of mycobacterial strains
rv2172c was amplified from wild-type M. tuberculosis H37Ra genomic DNA using polymerase chain reaction (PCR) with the primers Rv2172c-261F and Rv2172c-261R (Table S3). The purified amplicon was cloned into pMV261, yielding pMV261::rv2172c. pMV261::rv2172c T120P and pMV261::rv2172c M172V plasmids were generated using the Mut Express II Fast Mutagenesis Kit V2 (Vazyme, China). M. tuberculosis was transformed with sequence-confirmed pMV261 recombinant plasmid and plated on 7H10 plates containing 25 µg/mL kanamycin. After 3 weeks of incubation at 37°C, single colonies were purified, and liquid cultures were prepared for determination of PAS MICs. The presence of pMV261 recombinant plasmid was verified by PCR amplification using primers pMV261-IDF and pMV261-IDR (Table S3).
A modified strategy for phage-mediated allelic exchange [19,37] was used to obtain M. tuberculosis H37Ra derivatives containing the mutations T120P and M172 V on the rv2172c gene. Briefly, rv2172c (wild type) and rv2172c alleles were cloned into pMV261, and recombinant plasmids were transformed to M. tuberculosis H37Ra. The native copy of rv2172c was deleted by specialized transduction using phAE159 containing a hygromycin resistance cassette. All primers used are listed in Table S3.
Growth curves
1. M. tuberculosis H37Ra and its derivatives were cultured to mid-log phase (optical density, OD600: 0.5–1.0) and diluted to about 105 CFU/mL using 10-fold serial dilutions in fresh 7H9 (with OADC) medium. Then, bacteria were incubated at 37°C. The growth of bacterial cultures was measured by monitoring the OD600 using a spectrophotometer (Bio-Rad) at specified time points as the previously reported [19]. Measurements were conducted in three biological clones. The graphs for growth analysis were prepared using GraphPad Prism, and the mean values with standard deviations (SDs) were plotted against time.
DST
The susceptibility to PAS was tested as in our previous publication [19]. Mycobacterial cells were cultured to mid-log phase (OD600: 0.5–1.0) and diluted to about 105 CFU/mL using 10-fold serial dilutions in fresh 7H9 (with OADC) medium. Then, bacterial cells were plated on 7H10 (with OADC) agar solid plates containing various concentrations of PAS (0, 0.005, 0.01, 0.02, 0.04, 0.08, 0.16, 0.32, 0.64, 1.28, 2.56, 5.12, 10.24, 20.48, 40.96, and 81.92 μg/mL). PAS was purchased from MedChemExpress and solubilized in accordance with the manufacturer’s recommendations. Cultures were then incubated at 37°C for 21 days. The MIC was defined as the lowest concentration of antibiotics required to inhibit 99% of CFUs after this culture period. The MICs were performed through two technical repetitions using three biological replicates. All of the bacteria strains used are listed in Table S3.
Structural and homology analysis
We gathered six crystal structures of MTHFR containing either NADH or FAD, which include T. thermophilus TTHA_0327 (PDB ID: 3APY), H. influenzae MetF (PDB ID: 5UME), N. meningitidis MetF (PDB ID: 7RML), E. coli MetF (PDB ID: 1ZPT), S. cerevisiae MET12 (PDB ID: 6FNU), and M. smegmatis MSMEG_6649 (PDB ID: 7WMZ). These structures were visualized from a consistent perspective using PyMOL (http://pymol.sourceforge.net/). Subsequently, we conducted multiple sequence alignments of these six proteins with Rv2172c and MSMEG_6596 using MEGA11 (https://www.megasoftware.net/), and identified and annotated residues known to directly interact with NADH or FAD as reported [24,38-40]. Predicted models of Rv2172c were generated using AlphaFold2 [41]. Following the acquisition of the model structure of Rv2172c, we employed PyMOL to overlay it with the complex structure of MSMEG_6649. Subsequently, utilizing PyMOL, we depicted the noncovalent interactions between NADH and Rv2172c based on prior findings [24]. We then proceeded to illustrate the potential noncovalent interactions between Met172, Arg119, Thr120, and Met121 with NADH, inferred from the complex structure of MSMEG_6649 and NADH.
Protein expression and purification
The codon-optimized full-length cDNA of rv2172c, rv2172c T120P, and rv2172c M172 V was subcloned into pET21b (+) vector with a C-terminal HRV3C-6× His tag. The plasmids were transformed into E. coli ArcticExpress (DE3) and cultured in LB medium supplemented with 100 μg/mL ampicillin and 40 μg/mL gentamicin at 37°C. Protein expression was induced with 0.5 mM isopropyl-β-D-1-thiogalactopyranoside when the cells were grown at 37°C in LB broth to an OD600 of ∼0.6, and then, the cells were cultured further for 24 hours at 10°C before harvesting. The collected bacteria were resuspended and lysed by French Press in buffer containing 20 mM Hepes (pH 8.0) and 300 mM NaCl. After centrifugation, the protein was purified from the supernatant using nickelnitrilotriacetic acid (Ni-NTA) affinity chromatography. During dialysis into buffer A (20 mM Hepes (pH 8.0), and 150 mM NaCl), the HRV3C-His tag was cleaved by incubation with GST-tagged protease HRV3C at a 20:1 MTHFR:HRV3C (w/w) ratio. The protease was removed by glutathione affinity chromatography, and the flow-through was concentrated and subjected to a gel-filtration purification step (Superose 200, GE Healthcare) in buffer A. The peak fractions were collected and analyzed by SDS-PAGE.
In vitro enzymatic activity assays
Enzyme assays and kinetics analysis were performed following previous publications [23,42] with some changes. All experiments were conducted at 25°C and 100 mM potassium phosphate buffer (pH 8.0) with various concentrations of NADH and 5, 10-CH2-THF ranging from 0 to 150 µM and 0 to 1000 µM, respectively. A final concentration of 0.16 µM Rv2172c, Rv2172c T120P, and Rv2172c M172 V was used. Assay was started by adding 5, 10-CH2-THF and monitoring NADH oxidation at 340 nm (ϵ340 = 6,220 M−1 cm−1) for 5 minutes. Initial velocity data were fit with the Michaelis–Menten equation using GraphPad Prism in line with a previous study [42], and the mean values with SDs were plotted.
Absorbance at 340 nm was detected for the first minute in different reactions, and measurements were performed in two repeats as previously reported [23]. The mean values with SDs were plotted and the equations were fitted using GraphPad Prism as previously reported [42]. Then, the activity of each protein was quantified by the initial reaction rate (slope of the reaction fit line within one minute), and the relative percent activities of proteins were determined with respect to Rv2172c (the activity of Rv2172c was taken as 100%). Final results were presented as means with SDs, in three independent experiments. The p values were calculated using two-tailed unpaired t tests. **p < 0.01, ***p < 0.001.
Determination of methionine content in vivo
Samples were prepared as in our previous study [19]. Bacteria samples (∼109 CFU) were crushed, and supernatants were tagged by 5-AIQC. The 5-AIQC–tagged samples were filtered using a 0.22-µm membrane filter before ultraperformance liquid chromatography-tandem mass spectrometry (UPLC–MS/MS) analysis. Methionine was determined as in our previous article [19]. UPLC–MS/MS consisted of an Agilent 1290 UPLC coupled to an Agilent 6470 triple quadrupole mass spectrometer equipped with an electrospray ionization (ESI) source (Agilent Technologies, USA). A total of 1 μL sample was injected on an UPLC column (Agilent ZORBAX RRHD Eclipse XDB C18 column, 2.1 × 50 mm, 1.8 μm particles) with the temperature set at 50°C. The mobile phase consisted of the following: solvent A (ddH2O with 2 mM ammonium bicarbonate) and solvent B (methanol with 0.16% (v/v) formic acid and ammonium formate). The gradient used was as follows: 0–2.5 minutes, 5%–16% B; 2.5–6 minutes, 16%–22.5% B; 6–8.5 minutes, 22.5%–95% B; 8.5–12 minutes, 95% B at a flow rate of 0.5 mL/min. Electrospray ionization was performed using the positive ion mode, the pressure of the nebulizer was 50 psi, the sheath gas temperature was 350°C with a flow rate of 10 L/min, the dry gas temperature was 315°C with a flow rate of 10 L/min, and the capillary was set at 4000 V. Multiple reaction monitoring was used for the quantification of screening fragment ions. Peak determination and peak area integration were performed using Mass Hunter Workstation software (Agilent, Version B.08.00). Standard curves were constructed using least-squares linear regression analysis using the peak area ratio of derivatized individual standards against the nominal concentration of the calibrator. The quantification of samples was performed identically. The bacterial biomasses of the individual samples were determined by measuring the residual protein content of the metabolite extracts [4]. All data obtained by metabolomics were an average of independent sextuplicates. The p values were calculated using two-tailed unpaired t tests (**p < 0.01, ***p < 0.001). The graphs for the determination of methionine in vivo were prepared using GraphPad Prism, and mean values with SDs were plotted.
Results
rv2172c T120P and M172 V mutations are enriched in PAS-R clinical isolates
We analyzed the 7061 M. tuberculosis genomes downloaded from the NCBI database and found that the frequency of mutations on rv2172c gene was 1.06% (75/7061) (). Single amino acid variants on rv2172c were dispersedly identified at positions 32, 37, 39, 40, 77, 92, 93, 120, 166, 172, 194, 195, 212, 216, and 242 (Table S1). We also performed the analysis on the genome sequences of the 173 PAS-R and 803 PAS-S clinical isolates (obtained from previous publications). Surprisingly, the mutations on rv2172c were enriched in the PAS-R clinical isolates (6.36%, 11/173), and only rv2172c T120P and M172 V mutations were identified (, Table S2). The frequency of T120P mutation was 1.16% (2/173) among the PAS-R isolates, and the mutation was not identified in the PAS-S isolates. In addition, 5.2% (9/173) of the PAS-R isolates harbored rv2172c M172 V mutation, whereas only 0.12% (1/803) of the PAS-S isolates had this mutation (). These data indicated that the rv2172c T120P or M172 V mutation might be specifically associated with PAS resistance. For comparison purposes, mutations on thyA, folC, and ribD—the most common molecular markers related to PAS resistance in clinical practice—were also analyzed in the 976 genomes of the PAS-R/S isolates and 7061 genomes from the NCBI (Table S1, Table S2). As shown in , the recognized molecular markers of PAS resistance were enriched in the PAS-R clinical isolates, and the frequencies of mutations on the thyA, folC, and ribD genes were 35.84% (62/173), 29.48% (51/173), and 9.25% (16/173), respectively, which is consistent with previous studies [6,8,9]. All (100%, 2/2) of the PAS-R isolates harboring the rv2172c T120P mutation and most (55.56%, 5/9) of the PAS-R isolates harboring the rv2172c M172 V mutation do not possess mutations on thyA, folC or ribD (Table S2), furtherly implying the unique contribution of the two mutations to PAS resistance.
T120P and M172 V mutations on rv2172c cause high level PAS resistance in M. tuberculosis
The original copy of the rv2172c gene on the M. tuberculosis chromosome could be deleted only in the presence of another copy of rv2172c introduced using a plasmid. To verify the speculation that the rv2172c T120P and rv2172c M172 V mutations are novel molecular markers for PAS resistance, plasmids pMV261::rv2172c, pMV261::rv2172c T120P, and pMV261::rv2172cM172V were transformed into H37Ra, yielding H37Ra pMV261::rv2172c, H37Ra pMV261::rv2172c T120P, and H37Ra pMV261::rv2172cM172V. Subsequently, the original copy of the rv2172c gene was knocked out in these strains, yielding H37Ra Δrv2172c pMV261::rv2172c, H37Ra Δrv2172c pMV261::rv2172cT120P, and H37Ra Δrv2172c pMV261::rv2172cM172V. Verification of rv2172c gene knockout was performed by junction PCR (Figure S1, Table S3). Then, the growth curves of all the strains were plotted. The results showed that the two mutant strains H37Ra Δrv2172c pMV261::rv2172cT120P and H37Ra Δrv2172c pMV261::rv2172cM172V showed a slightly retarded growth comparing with the control strain H37Ra Δrv2172c pMV261::rv2172c (Figure S2). Subsequently, the minimal inhibitory concentrations (MICs) of PAS to all of the strains were tested. The results showed that T120P or M172 V mutation on rv2172c led to high level resistance to PAS in M. tuberculosis ().
Table 1. rv2172c T120P or M172 V mutation leads to PAS resistance in M. tuberculosis
T120P or M172 V mutation may affect the binding of Rv2172c to NADH
To investigate the mechanism of PAS resistance caused by rv2172c T120P and M172 V mutations, we focused on the specific FAD-independent but NADH-dependent MTHFR activity of Rv2172c. A recent study has presented the crystal structure of the MSMEG_6649–NADH complex [24]. MSMEG_6649, a FAD-independent MTHFR from M. smegmatis, is one of the homologues of Rv2172c. The overview of the structures of the MSMEG_6649–NADH complex and several FAD-dependent MTHFR complexes (such as MTHFRs in Escherichia coli, Neisseria meningitidis, Haemophilus influenzae, Thermus thermophilus, and Saccharomyces cerevisiae) is shown in A. We observed that in the similar location where an FAD-dependent MTHFR binds to FAD, MSMEG_6649 binds to NADH. Meanwhile, homology comparison on primary sequences of MTHFRs in different species was performed (B). The FAD-interacting residues of MetF in E. coli or N. meningitidis, and the NADH-binding sites of MSMEG_6649 were marked based on the published studies [24,38-40]. The results showed that FAD-interacting residues (Tyr60, His88, Thr90, Leu117, Arg118, Gly119, Asp120, Tyr131, Ala132, Ala150, Tyr152, His156, Glu158, Asp165, Asn168, Arg171, Lys172, Ile181, and Gln183) were highly conserved in FAD-dependent MTHFRs, but few such residues were identified in the same positions of mycobacterial MTHFRs (B). Conversely, NADH-interacting residues of MSMEG_6649 were highly conserved among mycobacterial MTHFRs, and few such residues were found in the same positions of FAD-dependent MTHFRs (B). Moreover, structure superimposition of Rv2172c (modeled by AlphaFold2) and MSMEG_6649–NADH complex (PDB ID: 7WMZ) showed high similarity (with root-mean-square-deviation value of 1.07 Å), and the amino acids at the NADH-binding sites of MSMEG_6649 were exactly conserved with those at the same location of Rv2172c (C). Thus, we speculated that Rv2172c and MSMEG_6649 may have specific conserved NADH-binding residues and analyzing the potential NADH-dependent MTHFR activity of Rv2172c based on the structure of the MSMEG_6649–NADH complex may be appropriate. We can see that Met172 and Arg119/Met121 stabilize two opposite ends of ligand through hydrophobic interaction and H-bonds, respectively (D). The substitution of Met172 by Val with the shorter side chain may directly affect NADH binding. As for T120P mutation, the pyrrolidine ring of proline is well known for its tendency to alter secondary structures. It is located to a key loop where two neighboring residues Arg119 and Met121 are directly interacting with the ligand. Therefore, T120P mutation may induce conformational change to affect the NADH binding. Taken together, these analyses suggest that T120P or M172 V mutation may affect the binding of Rv2172c to NADH, and the true effects need to be furtherly detected in the subsequent experiments.
Figure 3 Predicting the effect of T120P and M172 V mutations on binding to nicotinamide adenine dinucleotide (NADH) based on the structure of MTHFR–coenzyme complex from different species. (A) Crystal structures of MTHFRs containing either NADH or flavin adenine dinucleotide (FAD) were visualized from a consistent perspective. FAD-dependent MTFHR: E. coli MetF (PDB ID: 1ZPT), N. meningitidis MetF (PDB ID: 7RML), H. influenzae MetF (PDB ID: 5UME), T. thermophilus TTHA_0327 (PDB ID: 3APY), S. cerevisiae MET12 (PDB ID: 6FNU). FAD-independent MTHFR: M. smegmatis MSMEG_6649 (PDB ID: 7WMZ). (B) Multiple sequence alignments of MTHFRs from different species. Residues potentially interacting with NADH or FAD are annotated. (C) Structure superimposition of Rv2172c (modeled by AlphaFold2) and MSMEG_6649–NADH complex (PDB ID: 7WMZ). Residues potentially interacting with NADH are listed in the embedded table. (D) Noncovalent interactions between Met172, Arg119, and Met121 with NADH in mycobacterium MTHFR.
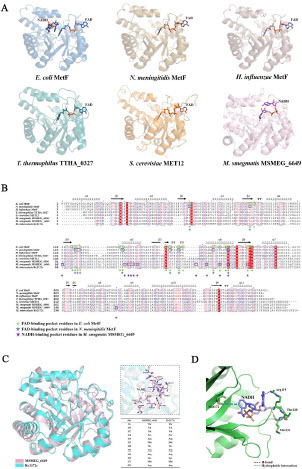
Enzymatic kinetics studies of Rv2172c, Rv2172c T120P, and Rv2172c M172V
Rv2172c, Rv2172c T120P, and Rv2172c M172 V proteins were purified successfully. Then, we performed enzymatic kinetics tests on these proteins and calculated their kinetic constants (). The Michaelis constant (Km) of Rv2172 for NADH was 1.63- and 1.86-fold higher than that of Rv2172c T120P and Rv2172c M172 V, respectively, showing that T120P and M172 V mutations lead to increased affinity for NADH. The catalytic efficiency (kcat/Km) of both Rv2172c T120P and Rv2172c M172 V was higher than that of Rv2172c.
Figure 4 Enzymatic kinetics studies of Rv2172c, Rv2172c T120P, and Rv2172c M172 V. (A) Michaelis–Menten plots of proteins for NADH. Embedded table shows kinetic constants. (B) Michaelis–Menten plots of proteins for 5, 10-CH2-THF. Embedded table shows kinetic constants. The means ± standard deviations (SDs) were determined from two independent replicates.
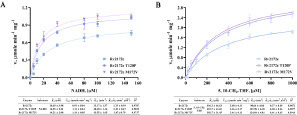
T120P and M172 V mutations increase the MTHFR activity of Rv2172c
To determine the effect of T120P and M172 V mutations on the MTHFR activity of Rv2172c, the catalytic reactions of Rv2172c, Rv2712c T120P, and Rv2172c M172 V were performed under NADH concentrations of 10, 20, 40, and 80 µM, or 5, 10-CH2-THF concentrations of 200, 400, 600, and 800 µM. The experiment was performed for three times (Figure S3, S4). The analysis of the relative MTHFR activity of those proteins showed that the MTHFR activity of the T120P or M172 V mutant protein was higher than that of the wild-type Rv2172c protein at different substrate concentrations, and the enzymatic activity of the M172 V mutant protein was slightly higher than that of the T120P mutant protein ().
Figure 5 Relative MTHFR activities of Rv2172c, Rv2172c T120P, and Rv2172c M172 V. (A) Enzymatic activities are measured in reactions with 250 µM 5, 10-CH2-THF and varied NADH concentrations (10, 20, 40, and 80 µM) (Figure S1). (B) Enzymatic activities are measured in reactions with 100 µM NADH and varied 5, 10-CH2-THF concentrations (200, 400, 600, and 800 µM) (Figure S2). The relative enzymatic activities of the mutant proteins are normalized based on the enzymatic activity of RV2172c (presented as 100%) in each reaction. Data are presented as means ± SDs, in three independent experiments. **p < 0.01, ***p < 0.001.
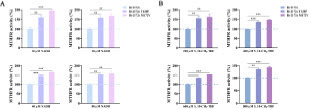
T120P and M172 V mutations on rv2172c increase the biosynthesis of methionine in M. tuberculosis
Rv2172c is the only enzyme that catalyzes the production of 5-CH3-THF in M. tuberculosis, which is essential for the synthesis of methionine and also essential for the in vitro growth of the bacterium (A). Thus, the changed MTHFR activity of Rv2172c may affect the synthesis of methionine. To further determine whether the increased MTHFR activity caused by T120P or M172 V mutation would lead to a corresponding increase in methionine synthesis, we detected the intracellular methionine contents of H37Ra pMV261::rv2172c, H37Ra pMV261::rv2172cT120P, H37Ra pMV261::rv2172cM172V, H37Ra Δrv2172c pMV261::rv2172c, H37Ra Δrv2172c pMV261::rv2172cT120P, and H37Ra Δrv2172c pMV261::rv2172cM172V strains. As shown in B, methionine concentrations were significantly higher in H37Ra pMV261::rv2172cT120P and H37Ra pMV261::rv2172cM172V comparing with that in H37Ra pMV261::rv2172c. Meanwhile, methionine concentrations were significantly higher in H37Ra Δrv2172c pMV261::rv2172cT120P and H37Ra Δrv2172c pMV261::rv2172cM172V comparing with that in H37Ra Δrv2172c pMV261::rv2172c.
Figure 6 rv2172c T120P and M172 V mutations increase the biosynthesis of methionine in M. tuberculosis. (A) MTHFR-catalyzed reaction of Rv2172c in methionine synthesis pathway. (B) The intracellular methionine concentration in H37Ra pMV261::rv2172c, H37Ra pMV261::rv2172cT120P, H37Ra pMV261::rv2172cM172V, H37Ra Δrv2172c pMV261::rv2172c, H37Ra Δrv2172c pMV261::rv2172cT120P, and H37Ra Δrv2172c pMV261::rv2172cM172V strains. **p < 0.01, ***p < 0.001.
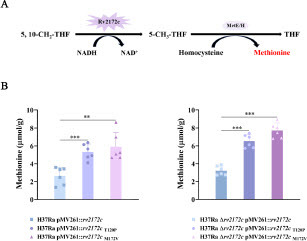
Discussion
PAS has been used as an antituberculosis drug for more than 70 years. Although mutations on thyA, folC, ribD, thyX, and metM have been linked to PAS resistance [5,7,11,13,14], the currently known resistance-related mutations cannot explain all resistance cases. Thus, it is necessary to discover new molecular markers of PAS resistance and reveal the relevant mechanisms. By analyzing genome-wide data of a large number of M. tuberculosis clinical isolates with DST result of PAS, we found that rv2172c T120P and M172 V mutations were enriched in PAS-R isolates, and the frequency of these two mutations was similar to that of the ribD mutations [8]. Subsequent experiments showed that both the T120P and M172 V mutations on the rv2172c gene caused high level resistance to PAS in M. tuberculosis, confirming that rv2172c T120P and M172 V mutations are novel molecular markers of PAS resistance.
Among the known molecular markers of PAS resistance in M. tuberculosis, mutations in thyA or folC lead to loss of function or functional deficiency of the corresponding enzymes, thereby causing PAS resistance [5,7,10]. However, the rv2172c T120P and M172 V mutations led to enhanced affinity for NADH and increased NADH-dependent enzymatic activity of the protein. MTHFR activity of Rv2172c is essential for methionine synthesis in bacteria. Correspondingly, a significant increase in intracellular methionine content was found in M. tuberculosis overexpressing rv2172c T120P and M172 V compared with M. tuberculosis overexpressing the wild-type rv2172c gene. In the 1950s, exogenous methionine was found to be able to antagonize the in vitro antimicrobial activity of PAS [43,44], but no follow-up work has been carried out in subsequent decades. Only in 2018, to explain the basis of methionine-mediated PAS antagonism, Howe et al. raised a hypothesis that methionine might promote inactivation of PAS through N, N-dimethylation by an unidentified methyltransferase [45], because a previous study showed that PAS could be converted to N, N-dimethyl PAS and then lose the antituberculosis activity [4]. However, the authors ultimately excluded this possibility and found that sustained production of para aminobenzoic acid was essential for methionine-mediated PAS resistance [45]. Later, we found that overexpressing the key enzymes of methionine biosynthesis in M. tuberculosis caused PAS resistance, while diminishing methionine biosynthesis made this bacterium more sensitive to PAS [19]. Very recently, Zhang et al. demonstrated that mutations in the promoter region of metM would lead to methionine-mediated PAS resistance[14]. Unlike current studies on methionine-mediated PAS resistance in the commonly recommended 7H10 medium, this resistance phenotype could only be detected in culture medium with additional methionine supply, which underscores the importance of considering the choice of culture media when conducting DST for M. tuberculosis [14]. In this study, we showed that T120P or M172 V mutation on rv2172c resulted in increased MTHFR activity, which in turn led to increased biosynthesis of methionine in M. tuberculosis and hence cause methionine-mediated PAS resistance, revealing a new mechanism of PAS resistance in M. tuberculosis clinical isolates. This is the first report showing that mutations within the coding region of a gene lead to increased enzymatic activity of the corresponding protein, thereby causing drug resistance in M. tuberculosis.
Rv2172c is the sole MTHFR in M. tuberculosis. Thus, it is essential for growth of the bacteria and cannot be knocked out even in the presence of exogenous methionine [19]. Therefore, the protein is an ideal target for antituberculosis drug development, and it is important to reveal the potential catalytic mechanism. NADH binding is required for the enzymatic activity of Rv2172c. Here, we showed that Rv2172c and MSMEG_6649 seemed to have specific conserved NADH-binding residues, which was supported by the experimental results that mutation at Thr120 or Met172 affected NADH binding and the MTHFR activity of Rv2172c. It should also be noted that T120P or M172 V mutation increase the MTHFR activity of Rv2172c, which is reminiscent of the possible cross-resistance between PAS and potential new antituberculosis drugs targeting Rv2172c.
Although rv2172c T120P or M172 V mutation is demonstrated to cause high level PAS resistance in M. tuberculosis, the detected frequency of these two mutations in PAS-R clinical isolates is not as high as that of mutations on thyA or folC. We observed that the two mutant stains with the rv2172c T120P and M172 V mutation both showed a slightly retarded growth in vitro comparing with the strain with the wild-type rv2172c. A previous study demonstrated that methionine synthesis affects the survival of M. tuberculosis in mice [46]. These data indicate that the two mutations (T120P and M172 V) on rv2172c might impose fitness costs, which merits further investigation.
Besides, rv2172c M172 V mutation was identified in one PAS-S isolate (Sample ID: ERR1664635). The inconsistency between genotypic result and phenotypic DST is not rare in clinical isolates. For example, the rpoB S450L mutation, which is well known to lead to rifampicin resistance, is also indentified in rifampicin-sensitive clinical isolates [25]. Moreover, we also observed that three PAS-S isolates harbor the folC I43 T mutation (Table S2), which was frequently identified in PAS-R clinical isolates [8,9]. These inconsistencies are generally attributed to the complex genetic background of clinical strains. We analyzed the genome data of the PAS-S isolate harboring the rv2172c M172 V mutation, and found missense mutations on pabC, metH and glyA1 (Table S4). All these three genes are involved in folate synthesis and metabolism. The pabC gene is essential for para aminobenzoic acid biosynthesis, and metH gene encodes one of the two methionine synthases in M. tuberculosis. Previous studies showed that sustained production of para aminobenzoic acid was essential for methionine-mediated PAS resistance [45], and decreased methionine production could cause increased sensitivity to PAS [19]. Thus, in this specific isolate, mutations on pabC and metH might hinder the biosynthesis of para aminobenzoic acid and methionine and then reverse the PAS resistance phenotype caused by the rv2172c M172 V mutation, which requires further investigation.
In conclusion, we verified rv2172c T120P and rv2172c M172 V mutations as novel molecular markers of PAS resistance in M. tuberculosis clinical isolates and revealed that the two mutations enhance the MTHFR activity of Rv2172c by increasing the affinity for NADH, thereby leading to increased biosynthesis of methionine and, consequently, methionine-mediated PAS resistance. Our study will improve the molecular diagnosis of PAS resistance in M. tuberculosis clinical isolates and facilitate development of new antituberculosis drugs targeting Rv2172c.
Author's contributions
J.-T. X., Investigation, Formal analysis, Data Curation, Visualization, Writing - Original Draft. J.-F. Y., Conceptualization, Methodology, Investigation, Formal analysis, Writing - Review & Editing. T.C., Software, Data Curation, Formal analysis. A. F., Investigation, Validation. P. Y., Methodology, Resources. J. G., Validation, Supervision. H.-J. Y., Resources, Supervision. J.-Y.D., Conceptualization, Resources, Supervision, Funding acquisition, Writing - Review & Editing. All authors have read and agree to the final version of this manuscript.
Figure S3.tif
Download TIFF Image (7.4 MB)Table S4.xlsx
Download MS Excel (10.8 KB)Figure S4.tif
Download TIFF Image (7.7 MB)Figure S2.tif
Download TIFF Image (281 KB)Figure S1.tif
Download TIFF Image (1.1 MB)Table S2.xlsx
Download MS Excel (44.4 KB)Supplementary Figures and Figure legends.docx
Download MS Word (16.5 MB)Table S3.xlsx
Download MS Excel (12.7 KB)Table S1.xlsx
Download MS Excel (45.1 KB)Acknowledgments
We thank Shanghai Metabolome Institute-Wuhan (www.smi-wh.cn/index.asp) for the assistance in the methionine content determination. We also thank LetPub (www.letpub.com) for its linguistic assistance during the preparation of this manuscript.
Disclosure statement
No potential conflict of interest was reported by the authors.
Funding
This work was supported by the Ministry of Science and Technology of the People’s Republic of China under Grant 2021YFA1300901.
References
- Millington KA, White RG, Lipman M, et al. The 2023 UN high-level meeting on tuberculosis: renewing hope, momentum, and commitment to end tuberculosis. Lancet Respir Med. 2024 Jan;12(1):10-13.
- Organization WH. Global Tuberculosis Report 2023 2023. Available from: https://www.who.int/teams/global-tuberculosis-programme/tb-reports/global-tuberculosis-report-2023
- Lehmann J. Para-aminosalicylic acid in the treatment of tuberculosis. Lancet. 1946 Jan 5;1(6384):15.
- Chakraborty S, Gruber T, Barry CE, 3rd, et al. Para-aminosalicylic acid acts as an alternative substrate of folate metabolism in Mycobacterium tuberculosis. Science. 2013 Jan 4;339(6115):88-91.
- Zhao F, Wang XD, Erber LN, et al. Binding pocket alterations in dihydrofolate synthase confer resistance to para-aminosalicylic acid in clinical isolates of Mycobacterium tuberculosis. Antimicrob Agents Chemother. 2014;58(3):1479-87.
- Mathys V, Wintjens R, Lefevre P, et al. Molecular genetics of para-aminosalicylic acid resistance in clinical isolates and spontaneous mutants of Mycobacterium tuberculosis. Antimicrob Agents Chemother. 2009 May;53(5):2100-9.
- Rengarajan J, Sassetti CM, Naroditskaya V, et al. The folate pathway is a target for resistance to the drug para-aminosalicylic acid (PAS) in mycobacteria. Mol Microbiol. 2004 Jul;53(1):275-82.
- Zhang X, Liu L, Zhang Y, et al. Genetic determinants involved in p-aminosalicylic acid resistance in clinical isolates from tuberculosis patients in northern China from 2006 to 2012. Antimicrob Agents Chemother. 2015 Feb;59(2):1320-4.
- Luo M, Li K, Zhang H, et al. Molecular characterization of para-aminosalicylic acid resistant Mycobacterium tuberculosis clinical isolates in southwestern China. Infect Drug Resist. 2019;12:2269-2275.
- Yu JF, Xu JT, Feng A, et al. Competition between H(4)PteGlu and H(2)PtePAS Confers para-Aminosalicylic Acid Resistance in Mycobacterium tuberculosis. Antibiotics (Basel). 2023 Dec 21;13(1).
- Zheng J, Rubin EJ, Bifani P, et al. para-Aminosalicylic acid is a prodrug targeting dihydrofolate reductase in Mycobacterium tuberculosis. J Biol Chem. 2013 Aug 9;288(32):23447-56.
- Cheng YS, Sacchettini JC. Structural Insights into Mycobacterium tuberculosis Rv2671 Protein as a Dihydrofolate Reductase Functional Analogue Contributing to para-Aminosalicylic Acid Resistance. Biochemistry. 2016 Feb 23;55(7):1107-19.
- Zeng R, He L, Zhang B, et al. Association between mutations in a thyX-hsdS.1 region and para-aminosalicylic acid resistance in Mycobacterium tuberculosis clinical isolates. Emerg Microbes Infect. 2023 Dec;12(2):2276339.
- Zhang Y, Wang S, Chen X, et al. Mutations in the promoter region of methionine transporter gene metM (Rv3253c) confer para-aminosalicylic acid (PAS) resistance in Mycobacterium tuberculosis. mBio. 2024 Feb 14;15(2):e0207323.
- Sheppard CA, Trimmer EE, Matthews RG. Purification and properties of NADH-dependent 5, 10-methylenetetrahydrofolate reductase (MetF) from Escherichia coli. J Bacteriol. 1999 Feb;181(3):718-25.
- Matthews RG, Sheppard C, Goulding C. Methylenetetrahydrofolate reductase and methionine synthase: biochemistry and molecular biology. Eur J Pediatr. 1998 Apr;157 Suppl 2:S54-9.
- González JC, Banerjee RV, Huang S, et al. Comparison of cobalamin-independent and cobalamin-dependent methionine synthases from Escherichia coli: two solutions to the same chemical problem. Biochemistry. 1992 Jul 7;31(26):6045-56.
- Guzzo MB, Nguyen HT, Pham TH, et al. Methylfolate Trap Promotes Bacterial Thymineless Death by Sulfa Drugs. PLoS Pathog. 2016 Oct;12(10):e1005949.
- Yu JF, Xu JT, Yang SS, et al. Decreased Methylenetetrahydrofolate Reductase Activity Leads to Increased Sensitivity to para-Aminosalicylic Acid in Mycobacterium tuberculosis. Antimicrob Agents Chemother. 2022 Jan 18;66(1):e0146521.
- Pejchal R, Sargeant R, Ludwig ML. Structures of NADH and CH3-H4folate complexes of Escherichia coli methylenetetrahydrofolate reductase reveal a spartan strategy for a ping-pong reaction. Biochemistry. 2005 Aug 30;44(34):11447-57.
- Igari S, Ohtaki A, Yamanaka Y, et al. Properties and crystal structure of methylenetetrahydrofolate reductase from Thermus thermophilus HB8. PLoS One. 2011;6(8):e23716.
- Froese DS, Kopec J, Rembeza E, et al. Structural basis for the regulation of human 5,10-methylenetetrahydrofolate reductase by phosphorylation and S-adenosylmethionine inhibition. Nat Commun. 2018 Jun 11;9(1):2261.
- Sah S, Lahry K, Talwar C, et al. Monomeric NADH-Oxidizing Methylenetetrahydrofolate Reductases from Mycobacterium smegmatis Lack Flavin Coenzyme. J Bacteriol. 2020 May 27;202(12).
- Li J, Yang M, Li W, et al. Structural and functional characterization of a mycobacterial methylenetetrahydrofolate reductase utilizing NADH as the exclusive cofactor. Biochem J. 2023 Jul 26;480(14):1129-1146.
- Hall MB, Coin LJM. Assessment of the 2021 WHO Mycobacterium tuberculosis drug resistance mutation catalogue on an independent dataset. Lancet Microbe. 2022 Sep;3(9):e645.
- Zhao B, Liu C, Fan J, et al. Transmission and Drug Resistance Genotype of Multidrug-Resistant or Rifampicin-Resistant Mycobacterium tuberculosis in Chongqing, China. Microbiol Spectr. 2022 Oct 26;10(5):e0240521.
- Chen X, He G, Wang S, et al. Evaluation of Whole-Genome Sequence Method to Diagnose Resistance of 13 Anti-tuberculosis Drugs and Characterize Resistance Genes in Clinical Multi-Drug Resistance Mycobacterium tuberculosis Isolates From China. Front Microbiol. 2019;10:1741.
- Nonghanphithak D, Kaewprasert O, Chaiyachat P, et al. Whole-genome sequence analysis and comparisons between drug-resistance mutations and minimum inhibitory concentrations of Mycobacterium tuberculosis isolates causing M/XDR-TB. PLoS One. 2020;15(12):e0244829.
- Che Y, Lin Y, Yang T, et al. Evaluation of whole-genome sequence to predict drug resistance of nine anti-tuberculosis drugs and characterize resistance genes in clinical rifampicin-resistant Mycobacterium tuberculosis isolates from Ningbo, China. Front Public Health. 2022;10:956171.
- Brown AC, Bryant JM, Einer-Jensen K, et al. Rapid Whole-Genome Sequencing of Mycobacterium tuberculosis Isolates Directly from Clinical Samples. J Clin Microbiol. 2015 Jul;53(7):2230-7.
- Doyle RM, Burgess C, Williams R, et al. Direct Whole-Genome Sequencing of Sputum Accurately Identifies Drug-Resistant Mycobacterium tuberculosis Faster than MGIT Culture Sequencing. J Clin Microbiol. 2018 Aug;56(8).
- Phelan J, O'Sullivan DM, Machado D, et al. The variability and reproducibility of whole genome sequencing technology for detecting resistance to anti-tuberculous drugs. Genome Med. 2016 Dec 22;8(1):132.
- Witney AA, Gould KA, Arnold A, et al. Clinical application of whole-genome sequencing to inform treatment for multidrug-resistant tuberculosis cases. J Clin Microbiol. 2015 May;53(5):1473-83.
- Li H, Kayani M, Gu Y, et al. Transmitted Extended-Spectrum Extensively Drug-Resistant Tuberculosis in Beijing, China, with Discordant Whole-Genome Sequencing Analysis Results. J Clin Microbiol. 2015 Aug;53(8):2781-4.
- Chen S, Zhou Y, Chen Y, et al. fastp: an ultra-fast all-in-one FASTQ preprocessor. Bioinformatics. 2018 Sep 1;34(17):i884-i890.
- Feuerriegel S, Köser C, Trübe L, et al. Thr202Ala in thyA is a marker for the Latin American Mediterranean lineage of the Mycobacterium tuberculosis complex rather than para-aminosalicylic acid resistance. Antimicrob Agents Chemother. 2010 Nov;54(11):4794-8.
- Bardarov S, Bardarov S, Pavelka MS, et al. Specialized transduction: an efficient method for generating marked and unmarked targeted gene disruptions in Mycobacterium tuberculosis, M. bovis BCG and M. smegmatis. Microbiology (Reading). 2002 Oct;148(Pt 10):3007-3017.
- Guenther BD, Sheppard CA, Tran P, et al. The structure and properties of methylenetetrahydrofolate reductase from Escherichia coli suggest how folate ameliorates human hyperhomocysteinemia. Nat Struct Biol. 1999 Apr;6(4):359-65.
- Pejchal R, Campbell E, Guenther BD, et al. Structural perturbations in the Ala --> Val polymorphism of methylenetetrahydrofolate reductase: how binding of folates may protect against inactivation. Biochemistry. 2006 Apr 18;45(15):4808-18.
- Pantong W, Pederick JL, Maenpuen S, et al. Biochemical and structural characterization of meningococcal methylenetetrahydrofolate reductase. Protein Sci. 2023 Jun;32(6):e4654.
- Varadi M, Anyango S, Deshpande M, et al. AlphaFold Protein Structure Database: massively expanding the structural coverage of protein-sequence space with high-accuracy models. Nucleic Acids Res. 2022 Jan 7;50(D1):D439-d444.
- Hajian B, Scocchera E, Shoen C, et al. Drugging the Folate Pathway in Mycobacterium tuberculosis: The Role of Multi-targeting Agents. Cell Chem Biol. 2019 Jun 20;26(6):781-791.e6.
- Hedgecock LW. Antagonism of the inhibitory action of aminosalicylic acid on Mycobacterium tuberculosis by methionine, biotin and certain fatty acids, amino acids, and purines. J Bacteriol. 1956 Dec;72(6):839-46.
- Hedgecock LW. Antagonism of methionine in aminosalicylate-inhibition of Mycobacterium tuberculosis. J Bacteriol. 1958 Apr;75(4):417-21.
- Howe MD, Kordus SL, Cole MS, et al. Methionine Antagonizes para-Aminosalicylic Acid Activity via Affecting Folate Precursor Biosynthesis in Mycobacterium tuberculosis. Front Cell Infect Microbiol. 2018;8:399.
- Berney M, Berney-Meyer L, Wong KW, et al. Essential roles of methionine and S-adenosylmethionine in the autarkic lifestyle of Mycobacterium tuberculosis. Proc Natl Acad Sci U S A. 2015 Aug 11;112(32):10008-13.