ABSTRACT
Hard tissue reconstruction of bone has attracted the interest of clinical researchers to overcome the anomalous effect on general well-being. Bone is a supporting skeleton structure that also plays a pivotal role in the normal physiological functioning body. The introduction of bone tissue engineering has been instrumental in overcoming fragmental defined bone to diseases, accidents, or debilitating conditions. For this, various bone grafting materials are used to restore the patency. These various bone grafting options have shown promising results. But the search for ideal grating material continues. However, the available grafting resources may not ensure regeneration. And the grafts like autogenous grafts that provide the capacity, are often, acquired in limited quantity. Its incapacity to fill the defect has led to the requirement of alternative material. Marine sources have shown acceptable clinical outcomes and are rendered safe for use also have a rich calcium phosphate-containing scaffold, over which the new cells are formed owing to its porous and 3D grains. The wide range of available marine sources has been put into clinical trials. The collagen found in the Marine sources has shown comparatively better results than that of the available synthetic alternatives.
Graphical abstract
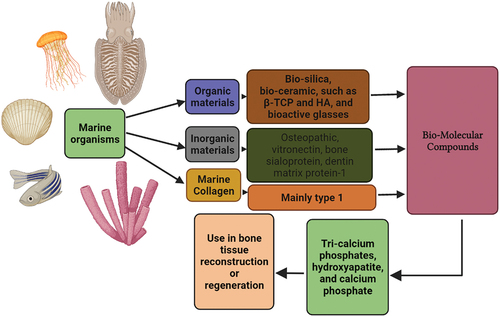
Introduction
Bone tissue reconstruction is the repairing of damaged fragments during bone fractures, tumor resections, or various bone diseases such as osteoarthritis, osteoporosis, osteomalacia, and osteogenesis imperfect [Citation1]. ‘Bone tissue engineering’ is a multi-disciplinary field as it connects with genetics, clinical medicine, mechanical engineering, and materials science [Citation2]. It is also used as a central tool for the development of regenerative medicines, utilized in biomimetics, cells, and tissue for regeneration [Citation3]. The National Science Foundation (NSF) was the pioneer to introduce this term in the year 1988. The idea of bone tissue regeneration is based on understanding the structural and functional components of the tissues during both the physiologic and pathologic scenarios. To this, the science of engineering techniques, their principle, and their application was amalgamated into tissue engineering [Citation4]. The key objective is to patch the defective region and return it to its original function [Citation5]. This review discusses the marine source as a potential alternative in the field of medicine and dentistry for bone tissue reconstructive indications.
Nowadays, the most daunting considerations of bone tissue engineering are the management of osteoporosis in elderly humans over 60 years of age [Citation6]. The aged females have a major risk than the males because women’s bones are protected by estrogenic hormone, the release of which decreases after menopause. Every year, Europe and the United States have reported approximately 400,000- and 600,000- people recovered from bone regeneration without any side effects [Citation7]. These statistics come with a high cost of more than $2.5 billion. The several available investigative reports predict that this may be doubled in the future, not just in the United States and Europe, but also in other countries [Citation8]. Besides, the limitations and complications of bone tissue regeneration are based on autologous or allogeneic transplantations using autografts and allografts techniques for clinical purposes [Citation9]. To date, an autograft is a gold standard procedure for bone grafts, which also has the highest quality level in bone recovery for both trauma and dentistry fields owing to its histocompatibility and non-immunogenicity. These grafts often lead to secondary trauma as the development of disturbance in donor bone like the iliac or fibula [Citation10]. Dentistry grafts are acquired from intraoral sites like the jawline, maxillary tuberosity, and ascending branch of the ramus. When a larger quantity is required, extraoral sites like iliac or tibia were considered. Autogenous graft utilizes individuals’ cells for regeneration. This minimizes the possibility of contamination and disease transmission like hepatitis. Also have some disadvantages like lack of vascularization, possible visceral injury during harvesting, infection at the donor site, high patient morbidity, pain, less quantity, and limited availability while advantages are excellent osteogenic, osteoconductive, and Osteoinductive properties [Citation11]. In the autograft method, the surgical time is increased due to additional anesthetic procedures which is a clear limitation [Citation12]. On other hand, the allograft technique of bone tissue reconstruction is procured from another individual. This must be done during the life of the donor individual or must be obtained from cadavers and preserved in a tissue repository [Citation10]. Allografts can be used as demineralized or mineralized that are normally preserved under freezing. The allografts have some advantages such as little inferior inductive ability because of insufficient growth factor, availability with different sizes, osteoconductive, and no donor site morbidity. Various shortcomings like the absence of osteogenic and vascularization properties, high cost, comparatively high graft rejection, and risk of transmission from a donor like human T-lymphotropic virus, hepatitis C, hepatitis B, HIV, etc [Citation13]. Allografts are broadly used in foot and ankle surgery which is a costly treatment and also an exhaustive process to eliminate its antigenic capacity. The denovo-formed bone does not comprise regenerative potential due to a lack of Osteo-inductivity [Citation14]. Considering the above mentioned loopholes, the regenerative approaches demand the usage of compounds with lesser side effects.
Bone tissue reconstructions are advanced bio substitutes in life sciences for both bone and tissue regeneration that proposes fixing up, animating, re-attempting, and improvement of wounded tissues, which are generated due to sickness, injury, or common inadequacies [Citation15]. The scaffolds and growth factors play a crucial role in bone tissue engineering [Citation16]. The 2-dimensional structural biology of cell bio-molecular signaling and interaction, and its shape are a major component that brings about the change. Reports have suggested that one of the advantages of the 2D scaffold is that the conventional cell 2D monolayer culture can grow specifically on flat surfaces [Citation17]. While the disadvantage is that there is no representation of a 3D complicated environment in vivo, which is nonproductive for use in clinical & therapeutic applications. Not under any condition like a 2D monolayer scaffold, a 3D scaffold is also broadly used in tissue engineering for bone tissue recovery [Citation18]. In spite, both material and biological engineering has three-dimensional (3D) models for the regeneration of natural tissue with their original structure and function. Although, reasonable scaffolds used in biomedical applications like the wellspring of biomaterial enhance mechanical properties to empower cells to perfect biocompatibility to help cell affiliation, biodegradability acceptability, duplication, development, differentiation, and movement [Citation19].
The 3D scaffold is composed of a couple of biomaterials with good mechanical and physical properties that are the major requirement for cell regeneration in bone tissue engineering. Unlike 2D scaffold that grows only flat cells, 3D scaffold grows on hydrogel cell encapsulation that proliferates in multi-cell layered tissue. This release gel-like matrix facilitates organ formation [Citation20]. The hydrogel protects and lets cells survive in a complex ECM matrix while keeping the intercellular signaling active [Citation21]. Although, the cell should be present in an artificial scaffold to be able to proliferate and differentiate during the regenerative process. Moreover, it provides a scope of neo-vascularization because of its pore size, oxygen availability, and aided nutrition and excretory function [Citation22]. Besides supplying, proliferation, migration, polarity, cell adhesion, and structural tensile strength are also facilitated. Also, extracellular matrix (ECM) and growth factors play a key role in bone tissue engineering. The ECM provides physical support to the cells and a suitable atmosphere for differentiation, cell proliferation, or morphogenesis [Citation23]. This corresponds to tissue regeneration occurring in damaged or injury sites by an artificial environment of cells providing a good scaffold of artificial ECM to initiate differentiation and cell proliferation. A suitable scaffold is the combination of cells or signaling molecules like chemokines, cytokines, and growth factors genes that can stimulate tissue regeneration. In some cases, growth factors and cell lines are necessary to accelerate tissue regeneration [Citation24].
Tissue engineering and regenerative medicine are two sectors where researchers are working, where cell lines play a vital role. Mesenchymal stem cells (MSCs) and adipose-derived stem cells (ADSCs) (MSCs), can distinguish into several lineages, activate immunogenicity, and their genetic stability is important for long-term cultures. According to the International Fat Applied Technology Society, human ADSCs are multipotent, immunomodulating, angiogenetic, and malleable, and are often isolated from adipose tissue. Because ADSCs are biocompatible, they represent a prospective treatment option for a variety of disorders [Citation25]. ADSCs are widely used in bone tissue engineering owing to their potential differentiation [Citation26] of mesenchymal cells like adipocytes, osteoblasts, and chondrocytes that support 3D scaffold activity. Human mesenchymal stem cells (hMSCs)are used for the management of diseases because of their immunogenicity, potency to differentiate, and cryo-preservation [Citation27]. The International Society for Cellular Therapy (ISCT) and the International Federation for Adipose Therapeutics (IFATS) have established fundamental criteria for mesenchymal stem cells, which include the presence of a specific set of cell surface markers, the absence of expression of CD14, CD34, CD45, and HLA-DR surface proteins, and the ability to differentiate into adipocytes, chondroblasts, and osteoblasts in vitro. Bone marrow can be used to extract HMSCs [Citation28] amniotic membrane, amniotic fluid, adipose tissue, endometrium, dental tissue, placenta, peripheral blood, menstrual blood, skin, salivary gland, fatal membrane, Wharton’s jelly, synovial fluid, and foreskin. Several growth factors found at the regenerative site have been discovered to serve an important role in various mechanisms of tissue regeneration, according to research. For example, epidermal growth factor (EGF) has osteogenic activity while Angiogenic activity is demonstrated by vascular endothelial growth factors and fibroblast growth factors [Citation29]. Fibroblast growth factor (FGF) has potential to the regenerate tissues like hard tissues, skin, veins, muscle, fat, ligament, bone, tooth, and nerves It can also regenerate bone and muscle owing to its potential activity of endothelial cell migration, proliferation, and survival [Citation30]. FGF also repairs blood vessels by differentiating to another cell as per requirement. The role of insulin-like growth factor-1 in bone, cartilage, and muscle regeneration was examined using osteoprogenitor cell proliferation and differentiation. The activity pattern of transforming growth factor-3 was similar [Citation31]. The vascular endothelial growth factor can regenerate bone, and blood vessels by enhancing the vascular formation and upregulating osteoblastic activity that is directly proportional to adjunctive BMP2 availed at the site [Citation32]. Platelet-derived growth factor (PDGF) is synthesized in human fibroblasts and smooth muscle cells from IGF-I (insulin-like growth factor I) or somatomedin C [Citation33]. In the initial stage, PDGF is released from platelets at the injury site; later it is also released from monocytes and macrophages to clot the blood [Citation34]. PDGF has incredible potential to quickly recover the wound region [Citation35], however, a low amount of PDGF is ineffective to heal the wound [Citation36]. Endothelial cell proliferation allows PDGF-AA and PDGF-BB to rebuild cartilage and bone. Also, repair blood vessels by replication of osteoblast and regenerate muscle by a synthesis of in vitro Type 1 collagen. PDGF is used to help ulcers in orthopedic clinical management and also repair periodontal tissue. Bennett and Schultz studied that the peptide growth factors can regenerate the eye tissue, and corneal cells (endothelial cells epithelial cells, and fibroblasts). EGF and TGF-alfa present in tears repair the corneal area wounds by an exocrine pathway [Citation37].
Marine sources have rich biodiversity and incredible potential for the advancement of biomaterials in bone tissue regeneration. On the basis of natural elements, the marine sources are biodegradable, biocompatible, osteoconductive, and stimulate osteogenesis without any toxic side effects. Therefore, marine sources are preferred over other materials when it comes to the scaffold. Typically, it is a huge challenge in science to use a marine source with extensive biodiversity in tissue engineering, where regeneration of defective bone is achieved. Keeping their potential in mind, the available various marine sources are still untapped. As indicated in , this review aims to highlight some of the naturally existing marine sources that can be used in bone tissue engineering.
Methodology
A fundamental and thorough review of the literature was undertaken until 2021 to determine the biological applications of marine sources derived biomatter from the utilization in bone tissue regeneration. A large number of offline and online databases were considered. Hindawi was the primary data source for this review article and research papers published by a number of respectable publishers, including Elsevier, Springer, and Taylor & Francis imprints. Some internet databases were also searched using terms related to data mining, NCBI, PubMed, Google Scholar, ProQuest, Scopus, and EBSCO are a few examples. The paid articles were accessed through Siksha O Anusandhan University’s Center Library. Other sources of literature, Conference proceedings, publications, Web pages, and book chapters, among other things, were investigated and accessible to maximize information regarding present bottlenecks, the degree of the study conducted, and the prospective utility of the issue. Several organic and inorganic bioactive compounds from various marine sources are discussed in this review. The main focus of this study is on current updates on marine-derived biomaterials, which will give researchers a complete understanding of the most recent developments and future research in the field of marine biotechnology.
Marine origin derived organic & inorganic sources
Organic and inorganic chemicals used in bone tissue regeneration can be found in abundance in the marine environment. Although the pharmacological and medicinal implications of numerous marine-derived collagens are considerable, these marine-based composite scaffolds for bone tissue production are still in the early stages of development. The current chapter included an overview of a variety of marine sources and their potential applications in Marine collagen composite materials or scaffolds that mimic bone is being developed for bone tissue engineering. This chapter’s cumulative information is intended to help novice marine biologists who are interested in biomedicine, specifically bone tissue regeneration. The majority of marine regenerating materials have both organic and inorganic components, as illustrated in . This is found to be homologous to that of the other investigated bone substitutes like allograft bone replacement materials. Where the newly formed bone consists of the following [Citation38–40]. Bio-ceramics type of bone substitute materials are mostly derived from marine sources and have shown promising results. The certain organic component of marine sources like seaweed, sponge, sea anemone, jellyfish, and others have rich collagenous content required for bone formation that is believed to be clinically better than the synthetic materials [Citation41,Citation42]. Hydroxyapatite and bio-silica are the best bio-ceramics in bone tissue engineering. Hydroxyapatite (HA) extracted from cuttlefish [Citation43], bovine bone [Citation44], and fish [Citation45] forms the core component of the bones and the teeth. In addition to sponges and corals, snail shells also produced HA by various methods discussed in the table below. HA has stable physiological pH, excellent biological properties, and strong chemical bonds among bone which is a vital requirement for a faster regenerative correction after bone damage [Citation46].
Table 1. Composition of marine sources as bone regenerative materials.
Bio-silica-based marine sponge spicule can develop bone meshwork by mineralization as a result of their physical and chemical qualities. From several biological investigations, it was found that the cell culture utilizes similar components present in a sponge (bio-silica and biogenic polyphosphate) [Citation47]. Bio-silica is commonly extracted from sponges, diatoms, radiolarians, and choanoflagellates [Citation48] by the thermal calcination method [Citation49]. According to physiological function, silica affirmation occurs before the ossification process. From biological study investigate the existence of silicate and the arrangement of cDNA in collagen (COLch) of marine sponge Chondrosiareniformis [Citation50]. Reported that the marine sponges-derived chitin has excellent potential in dental tissue regeneration while the sponge COLch mRNA protein can be directly used for the healing process [Citation51]. In addition to silica-collagen, the mongrel compound has the capacity for cell proliferation, adherence, and human stem cell differentiation demonstrating biocompatibility for bone regeneration [Citation52].
The cuttlefish (Sepia) has a unique internal shell known as a cuttlebone. This cuttlebone is suitable for bone graft materials that are often used as good templates for human bone tissue regenerations. It can be used directly on tissue injury or defect areas due to its buoyancy [Citation53], chemical composition [Citation54], crystallography, and good mechanical properties. This makes it suitable for direct usage on human bone tissue reconstruction making the process faster without any infection. In addition, cuttlebone is also used as a xenograft for bone defect treatment in male rabbits [Citation55]. Corals are a type of marine bio-ceramic that was popular in the early 1990s and is now accessible in the form of bio-coral and Interpore. A 3D coral skeleton structure was seen to encourage hard tissue formation and be replaced by a new bone in an animal investigation [Citation56].
Cellular growth and development are the basis for a regenerative activity to occur [Citation57]. Bone regeneration is indicated in the defect of trauma, infection, tumor, skeletal abnormalities, and avascular necrosis, osteoporosis. The two variants of the fish scale: ‘Sparidae’ and ‘Chanos’ are a rich reservoir of a collagenous protein named Marine collagen protein (MCP). These are the key component that enhances the osteoblastic activity and suppresses osteoclastic activity. MCP not only enhances cell proliferation but actively participates in mineralization activity as well. It acts as a potential marker. Alkaline phosphatase activities are also noted with it. Another remarkable property is that it differentiates osteoblast cells like hMSCs [Citation58].
Marine sponge collagen (Porifera, dictyoceratida) is composed of polymeric containing l-cysteine hydrochloride for lacerate remedial [Citation59] and cysteine (biopolymer) has the potency to heal the wound region. The marine sponge is progressively used for tissue repair because of its cell conductivity and exploratory framework [Citation60]. Nowadays bone substitute materials are used for bone tissue reconstruction as the chemistry and topography of these materials have proper differentiation potency, proliferation, and cell growth. The marine sponge, which contains calcareous or siliceous spicules or collagen/spongin, creates a porous biomimetic environment that promotes osteogenesis in vitro by replicating the cancellous architecture of bone tissue and the complicated canal system [Citation61]. Marine seashell is the best biogenic material consisting of carbonate with richly interspersed micro-structures in a densely compact structure [Citation62]. Marine seashells can improve the osteogenic response to human tissue due to strong mechanical, Osteoinductive properties and orthopedic application [Citation63]. The seashells can convert into ceramic biomaterials like calcium phosphate ceramics, hydroxyapatite, and tri-calcium phosphates, which are directly used in bone substitute or repair [Citation64].
Marine skeletons contain interconnecting porous structures (20–500 µm) that allow nutritious exchange and cell infiltration via vascular channels. The carbonate porous skeleton can maintain the structural integrity of scaffolds. The stronger calcium phosphate is also useful bioactive natural material for regenerative medicines [Citation65]. Like other marine skeletons, sea urchin has good crystal architecture, microstructure, biomimicking property, and a multifunctional single material that forms a new bone material [Citation66]. By using advanced technology, marine microfluid can be used to replicate the marine skeleton for the regenerative procedure in humans [Citation67].
Some coral skeletons have intertwined types of pore architecture. The inorganic composition of corals corresponds to that of human bone. It is the trabecular and porosity pattern that mimics the natural setting and is therefore used [Citation68]. Commonly natural coral contains 99% calcium carbonate which is completely used in bone graft materials like osteoporosis, fracture repair, spinal surgery, trephine hole replacement, hip fractures, tibial osteotomies, maxillocranio facial, bone defects, cosmetic surgery, and periodontal injury, etc because the animal and human bodies have the potential to rapidly absorbed calcium carbonate for the formation of new bone tissue on a coralline scaffold [Citation69]. But Zoanthid and Palythoa coral species are produced a toxic substance known as Palytoxin which directly promotes osteoclast instead of osteoclast formation [Citation70].
Nacre is a mother of pearl. It represents the complex meshwork of organic material interspersed with inorganic. The outer covering is rich in proteins and glycoproteins and organic polysaccharides. In vitro study has discovered a hydrophilic component of oysters that is a soluble matrix in an aqueous bio-environment [Citation71]. Nacre has the potency to increase osteoblastic differentiation and increased Col-I, osteocalcin, and ALP expression. Nacre has antioxidant potential due to the release of free radicals which can support bone regeneration.
Another rich source of collagen is obtained from sharks. Both its skin and teeth obtained from Prionaceglauca and Isurusoxyrinchus have the regenerative capacity. Pallela et al. reported that Irciniid collagens from the sea sponge Ircinia fusca were combined with natural hydroxyapatite, chitosan matrix, Thunnus obesus bone, and chitosan-hydroxyapatite-marine sponge collagen have the capacity to regenerate bone [Citation72]. Collagen, HA, and chitosan-composite sponge are classified as type-2 collagen obtained from the cartilaginous structure of Prionaceglauca Shark [Citation73]. MC/hydroxyapatite-based sponge has the property to enhance human osteoblastic cells like MG-63 [Citation74] HA crystals and poly-methyl-methacrylate (PMMA) have also shown acceptable regenerative potential. Mredha et al., observed the repairing potential of collagen and PDMA agents obtained from Bester-sturgeon fish [Citation75]. Similar experimentation with collagen obtained from other sources like salmon and jellyfish displayed clinically acceptable results [Citation76].
One study suggested that the collagen hydrolyzate (CH) is composed of Rhizostoma Pulmo collagen functionalized with hydroxy-phenyl-propionic acid (HPA) and marine gelatin Rhizostoma Pulmo collagen functionalized with hydroxy-phenyl-propionic acid (HPA) and marine gelatin has a significant health supplement effect in-vivo model, calculated dose of CH in the concentration of 40, 80, 160 mg/kg were used along with fish food in daily basis for two weeks to confirm the bone regeneration potency of zebrafish, the result reveals that CH produces closure of gap about 149 μm at the dose of 160 mg/kg, while in the untreated group the gap size only 238 μm, suggests CH has significant bone regeneration property because of alkaline phosphate and calcium carbonate [Citation77]. CH was tested in vitro on MC3T3-E1 osteoblast and RAW264.7 osteoclast cell lines at three different doses of 0.25, 0.5, and 1 mg/ml, followed by alkaline phosphatase, Alizarin red, and In TRAP In staining. qPCR was used to measure the expression of osteogenic genes; 0.5 mg/ml CH boosted ALP activity and calcium deposition while decreasing the size of osteoclasts. Furthermore, osteocalcin and RUNX2 (Runt-related transcription factor 2) gene expression was elevated. The therapeutic potential of CH for fracture repair and gap-filling depends on concentration, and it enhanced osteoblasts while decreasing osteoclasts [Citation78]. The biological investigation found that marine collagen has a faster regenerative capacity with a safety profile than other synthetic collagen due to its being rich in alkaline phosphate and calcium carbonate [Citation79]. The zebrafish & human body have 69% genome similarity, and 84% disease & epidemiological relevance so that marine collagen could be used in human bone tissue reconstruction [Citation80]. Likewise, freshwater collagen also plays a crucial role in bone tissue regeneration as shown in .
Articular cartilage damage is a musculoskeletal disease that cannot be treated completely and so total joint replacement is essential. The cartilage is an avascular structure with limited capacity for self-regeneration. Tissue engineering in this field offers to repair, the regenerative, reconstructive capacity of articular cartilage therapy. Based on clinical studies, Marine Collagen Peptide (MCP) is used as a therapeutic intervention against human osteoarthritis and osteoporosis as it is helpful in matrix synthesis of cartilaginous components and pain management [Citation81]. Marine biopolymers and polysaccharides play a crucial role in biomedical applications. Chitin is a natural mucopolysaccharide, found in exoskeletons of arthropods or fungi, yeast cell walls, crab/shrimp shells, Crawfish lobster, Crayfish, and Plankton [Citation82]. Other potential sources are diatoms and squid. The nematodes and lobsters also possess properties favorable for regeneration [Citation83]. The powder from Chitosan promotes inductivity in human osseous environments. It is bio-compatible and is safely used [Citation84]. The main potential activity of chitosan is found associated with the stem cells of bone marrow origin (BMSCs) both in the laboratory and clinical trials [Citation85]. Alginates, due to their minimized antigenicity and good acceptability, are a favorable option too. This material with chelating property is also easily moldable into desired shapes that best fill the defect area [Citation86]. Its hygro-gel formulation promotes new cartilage formation [Citation87]. The Orthopaedicians prefer this material as it fills the defect with ease and with less dexterity. Remarkably, the growth factor released with Bone morphogenetic protein and Tumor growth factor-alpha is noteworthy [Citation88]. In described the marine flora and fauna-derived bio-molecular compounds with their application in the regenerative field.
Table 2. Marine flora and fauna-derived bio-molecular compounds with their application in the regenerative field.
The complex polysaccharide obtained from Undaria pinnatifida – fucoidan is used in the management of osteoarthritis [Citation89]. Lowering its molecular weight enhances its regenerative potential [Citation90]. The alkaline phosphatase activity is promoted by this complex polysaccharide. Collagen type 1 is expressed at the defect site. Besides, Runx-2, osteopontin, and osteocalcin enhance osteogenic cells to differentiate into pluripotent cells [Citation91]. A simple process of bone formation or regeneration is described in .
The organic and inorganic bioactive molecules of marine sources play a pivotal role in the formation of osteoblasts in the presence of c-Fms, M-CSF, and OPG, that is prevented the binding of RANK and RANKL signaling pathways and helps the proliferation and survival of osteoblast. The NO acts as a positive inducer for osteoblast formation through eNOs. The prostaglandinE2 (PGE2) can activate EP2 to inhibit cAMP while EP3 activates cAMP through α3-GTP, the cAMP is induced the bone formation by PKA and CREB, PGE2 inhibits osteoblast through EP1, PLC-γ, and Ca2+ channel. The parathyroid hormone (PTH) promotes osteoblast by PTH1R and inhibits osteoclast in the presence of OPG (-) and RANKL (+) pathways. The 25(OH)D3can activate 1α,25(OH)2D3, which stimulates bone calcification via downregulation of RUNX2anddirectly promotes bone resorption. On other hand, the organic and inorganic bioactive molecules of marine sources are sometimes released cytokines and growth factors that are responsible for the binding of RANK and RANKL signaling pathways and lead to preosteoclasts after that their fusion to form osteoclasts by activating growth factors like TGF-β, IGFs, FGFs, PDGFs, BMPs. The coral reef releases palytoxin which is responsible for activating osteoclast. The molecular mechanism of bone regeneration in .
Applications of marine-derived organic and inorganic biomaterials used in bone tissue repair and regeneration
In recent years, the need for bone biomaterials, often known as bone graft alternatives, has increased. In general, Natural bone is a composite material composed of both organic and inorganic components. Collagen fibers, particularly tropocollagen, which promotes the bone hardness and is plentiful in marine sources, make up the majority of the organic constituents. Inorganic components include calcium (Ca) and phosphorus (P) in the form of hydroxyapatite (HA) crystals, as well as sodium (Na), potassium (K), magnesium (Mg), fluoride (F), chlorine (Cl), and carbonate (CO3 2), which provide bone strength and are also present in marine sources. Natural bone is made up of many scales that can be cortical or cancellous. The cortical bone holds 99% of the calcium and 90% of the phosphate in the human body on its surface. It has a low porosity of 5%–10%, making it relatively dense and durable. Cancellous bone is a form of bone that is spongy and dispersed throughout the bone. It is composed of intertwining lamellar trabeculae containing hematopoietic cells, adipose tissue, and blood arteries. Cancellous bone accounts for around 20% of all bone in the human body, despite having a porosity range of 50% to 90% and a specific surface area over 20 times that of cortical bone. These unique compositions and architectures equip bone with extraordinary qualities that allow it to perform a wide range of jobs. Bone composition and structure, on the other hand, vary depending on the location of the lesion, the patient’s age, genetic inheritance, and living situations, resulting in a wide variety of bone-implant needs. As a result, developing bone biomaterials that suit the needs of bone healing has long been difficult. In several biological studies, bone biomaterials have been discovered to be similar to biomaterials obtained from marine sources. In this case, marine-derived biomaterials can be employed as bone grafts, bone repair, or bone regeneration [Citation92]. Bioactive ceramics offered from the Marine Source include Ca–P ceramics, Ca–Si ceramics, bioactive glasses, and calcium sulfates Two Ca–P ceramics with compositions comparable to bone are HA [Ca10(PO4)6(OH)2] and tricalcium phosphate [TCP, Ca3(PO4)2]. Not only are they biocompatible and osteoconductive, but they may also Osseo merge with the issue site. Furthermore, by providing an alkaline environment, the breakdown products, and released ions can benefit human metabolism by boosting cell activity and speeding up bone healing. The most abundant mineral in natural bone biomaterial is HA. It has a theoretical density of 3.16 grams per cubic centimeter and a Ca/P molar ratio of 1.67. It is capable of guiding the new bone formation and creating chemical bonds with bone tissue after implantation, with a bonding strength 5–7 times that of bioinert ceramics and genuine bone. Meanwhile, in the human body, HA has a high affinity for amino acids, proteins, and organic acids, and its hydroxyl groups have a significant attraction for them via hydrogen bonding. HA, on the other hand, is extremely crystallin and stable. However, employing bioactive ceramics as bone biomaterials has significant limitations, including insufficient hardness and strength [Citation92]. Collagen, chitosan, and cellulose all-natural marine polymers that can induce osteoblast development. In addition to having great bioactivity, cell affinity, and hydrophilicity, the biopolymers are nontoxic, have minimal adverse effects, and are widely available. The majority of their breakdown products are amino acids, which are easily absorbed by the human body. Collagen, as one of the most significant extracellular components, aids cell migration and expansion by establishing the structure of the ECM, giving cells the flexibility they require. Collagen implants were created using a freeze-drying process, and in vivo data showed that collagen implants improved cell migration and blood vessel formation. In contrast to bone morphogenetic protein 2 (BMP-2) and dexamethasone (Dex), both growth factors were identified to be produced concurrently with a material breakdown in collagen composite fibers, allowing bone marrow mesenchymal stem cells (BMSCs) to differentiate into osteoblasts. Chitosan is the only type of alkaline amino-polysaccharide that chitin produces. In terms of structure and characteristics, chitosan is quite similar to the amino-polysaccharides found in the ECM. Its surface is densely packed with positive charges, which are favorable groups that can be adjusted to meet individual needs (for example, sulfonation, esterification, and etherification). To obtain the porosity, chitosan was freeze-dried and then employed in cell culture studies using rat calvaria osteoblasts. Chitosan has been shown to promote osteoblast proliferation and differentiation. After cells were cultivated on chitosan, calcium deposition and new bone formation were also seen. Similar investigations have indicated that chitosan improves osteoblast differentiation [Citation93].
Collagen is found in ophthalmic shields, burn/wound sponges, mini pellets and tablets for protein delivery, gel formulation with liposomes for sustained drug delivery, as a controlling material for transdermal delivery, nanoparticles for gene delivery, and basic matrices for various cell culture systems. Although collagen’s applications vary depending on the presence of disease in the human body, the physio-functional value of diverse collagens is essentially the same. The use of marine collagen in the manufacture of medical, cosmetic, and biological products appears promising. Collagen has been found in sponges (Porifera), jellyfish (Cnidaria), octopus and squid (Mollusca), and fish. Not only are there several sources of marine invertebrate collagen, However, marine vertebrate collagen, which is identical to human collagen, is also present. Collagen is abundant in fish and could be employed in a range of bioprocess and biomedical engineering applications. Other marine mammals with high mean bone collagen levels, such as baleen whales, sea otters, and walruses, have been widely examined in bio-physicochemical research. The majority of skeletal bones are made up of osteoconductive collagen (mainly type I) and carbonate substituted HAp. When collagen, HAp, and other natural and synthetic macromolecules are combined, they promote osteoblast growth and hence speed up osteogenesis. Mineralized porosity structures are found in corals, algae (diatoms), and sponges, and some of these structures have been biomineralized and are presently employed as bone transplant materials. Despite structural hardening and calcification, collagen from marine animals has been discovered to be a functionally relevant bone grafting material. The isolation, characterization, and design of collagen for in vitro and in vivo usage as biomedical materials for bone tissue engineering is an ongoing process that begins with marine sources [Citation94].
Marine sources derived bio-ceramic xenografts
Marine sources provided bio-ceramics with hierarchical porosity patterns similar to human trabecular bone, making them suitable bone transplant materials with osteoconductive activity. Aragonite, a crystalline ceramic structure, is widely used in the exoskeletons of various coral species (calcium carbonate). Porites and Goniopora, two reef-building coral skeletons, are commercially employed as bone transplants due to their abundance and regular structure. Thermal treatment of these bio-ceramics eliminates immunogenic responses, leaving only trace amounts of intra-crystalline proteins. Bio-ceramics have a compression weakness and calcium carbonate absorption is too quick, limiting its application. A hydrothermal conversion from natural calcium carbonate composition to hydroxyapatite can be carried out to harden coral skeletons and sustain significant compressive pressures exerted in load-bearing long bones. The resulting hydroxyapatite degrades slowly, enhancing graft durability, and total resorption can take a year or more. One year following surgery for lumbar spine degeneration, 100% coralline hydroxyapatite resorption (mixed with local bone and bone marrow) was reported in dorsal and lateral fusion. After multiple biological testing, the biomaterial was detected in hindfoot arthrodesis, concluding its utility as a bone graft in clinical foot procedures, with extrusion occurring in all cases and a resorption rate that was too slow (graft presence up to 6 years after surgery). Researchers created a method for dental implantation recipient site surgical preparation that employs coralline HA granules and homologous fibrin glue to reduce the risk of failure due to mechanical instability and low retention at the surgical site. Another possibility is grafts created from converted coral skeletons that haven’t been converted to hydroxyapatite but still include some calcium carbonates [Citation95]. Biodegradability has been increased to aid in bone remodeling and spontaneous bone regeneration. By developing these oceanic forms in artificial aquaria, contamination issues (intracrystalline proteins that accompany biogenic crystals) and environmental impact are addressed. Aquaculture provides for precise control of environmental variables as well as the addition of specific ions of interest during the growth stage (as silicate or phosphate ions). This approach improves the bioactivity of the resultant mineral product by improving the calcium carbonate structure. Commercial coralline-derived grafts are now available to doctors. Two products on the market that combine DBM allografts with coralline-graft granules are StaGraftTM DBM PLUS (Zimmer Biomet) and Pro-Osteon®500 R coralline hydroxyapatite/calcium carbonate granules. These items are recommended for filling bony voids or gaps in the extremities and pelvis that are not intrinsic to the skeletal stability of the structure, as well as autograft extender, bone void filler in the spine (posterior spine), and autograft extender in the spine, and craniofacial defect fillers in craniotomies no larger than 25 cm2 [Citation96].
Marine sources derived used in xenografts research
Even though marine sources are used in Xenograft research, their rapid disintegration rate, low endurance, and instability make them unsuitable for most long-term implant applications, and commercially accessible unconverted coral (calcium carbonate) is currently unavailable. Furthermore, coralline grafts with partial coral conversion to hydroxyapatite have poor mechanical qualities for load-bearing applications requiring great structural strength. As a result, unique strategies for filling micro and nano-holes while retaining large pores, such as converting coral to pure hydroxyapatite and then coating it with a sol-gel-derived HA, have been examined. In the physiological environment, this technique increased biaxial strength twofold, resulting in better durability, longevity, and strength for load-bearing bone graft applications that demand high strength. The coralline genesis in this example is anecdotal. Even though marine sources are used in Xenograft research, their quick disintegration rate, low endurance, and instability render them inappropriate. Because, in addition to chemical modifications, morphological changes are produced to the porosity of the original structure. Previously, fluorine and zirconia-doped coralline HA were utilized to improve mechanical strength, as was the recent addition of Sr ions to natural coralline HA to promote bone formation while inhibiting bone resorption. In an alveolar defect animal model, nano-hydroxyapatite/coralline grafts coated with VEGF dramatically improved neovascularization and mineralization. Nacre, seashells (foraminifera, bivalve mollusks such as oysters and mussels), sponge skeletons, diatom frustules, sea urchin spines, cuttlefish bone, and other fish bones such as tuna, and shark teeth are traditional marine bio-ceramic materials. When manufactured as powders, these ceramics possessed rod-like particles with an average size of less than a micron and good ‘in vitro’ biocompatibility independent of the fish source. Several marine bio-ceramic materials, such as seashells, fish bones, and shark teeth, are trash or byproducts of the fishing and food industries and so abundant. The re-valuation of this waste into higher-value items, such as bone transplants, adds to long-term sustainability. CaCO3 is the most common component of its structure, as it is in nacre, oyster, and mussel shells, calcareous sponge skeletons, sea urchin spines, and cuttlefish bone [Citation97]. Others are silicon-based, such as sponge skeletons and diatom frustules. Fish bones and shark teeth are notable because their calcium phosphate composition is similar to that of human bones. Nacre is by far the most researched calcium carbonate source as a bone graft. It is formed of highly crystallized cellular calcium carbonate powder and pseudohexagonal aragonite nanograins wrapped in an intracrystalline organic matrix. Thermal treatment at 550–600 degrees Celsius, the temperature at which aragonite is already changed to calcite (which occurs at 300–400 degrees Celsius), could eliminate it if organic content is reduced to 1.7% or less. A recent study that collated ‘in vivo’ and ‘in vitro’ research data validated the osteoinductive capability, as well as osteoconductivity, biocompatibility, and biodegradability. When implanted in numerous regions for diverse activities such as human maxillary alveolar bone, load-bearing site fillers, cranial deficiency fillers, and intervertebral fusion using different animal models, it promoted new bone formation. As a result, this material might meet the increasing need for load-bearing and osteoinductive bone fillers. Because of its exceptional mechanical properties, such as Young’s modulus of 70 GPa (dry) or 60 GPa (wet), which is equivalent to steel, nacre has a lot of potential in the field of bone substitutes. The lack of commercially accessible nacre products for clinical usage may be due to regulatory concerns or simply economic hurdles (such as profitability) preventing it from accessing the bone graft market. A substantial amount of literature is currently available on the synthesis of nacre-mimetic composites. The previously mentioned fishing by-product shark tooth is another source of calcium phosphate that is now being researched. Heat processing eliminates the biological material, and shark tooth enamel and dentine are two direct sources of bioapatite. Dentine is a porous biphasic bio-ceramic with a globular shape and bimodal porosity (50 m and 0.5–1.0 m). contains a list of bio-ceramic xenografts derived from marine sources that are now commercially available. Shark enamel is composed of 91% apatite fluorapatite in the form of aligned elongated crystals with the shortest dimension of 0.5–1.0 m and a trace of whitlockite/-TCP. The enameled bio-ceramic will aid to increase bone transplant mechanical properties of bulk moduli and stiffness constants since it is six times tougher than dentine and has significant mechanical capabilities. Fluor ions integrated into the apatite lattice also provide acid resistance and are hypothesized to function as a cell growth factor enhancer, especially on osteoprogenitor cells and/or undifferentiated osteoblasts. As a result, its presence may accelerate bone healing and regeneration by causing osteoprogenitor and undifferentiated precursor cells to grow into osteoblasts. Furthermore, the presence of fluorapatite reduces resorption. The non-apatite phase of shark dentine would increase and change (as required) the graft’s resorption rate. Furthermore, the presence of trace elements that play essential roles in bone metabolisms, such as Mg, Na, Sr, K, Al, and Fe, lends these grafts osteoinductive properties. These shark teeth grafts outperformed commercial synthetic and bovine bone grafts in terms of early osteogenic activity (p 0.01) on MC3T3-E1 pre-osteoblasts after 21 days of incubation, indicating their significant bone regeneration results. Furthermore, after three weeks of implantation in a rodent model, the porosity provided by dentine, combined with the inter-granular voids, allowed for new bone cell ingrowth (osteoconductive properties), indicating higher osseointegration than commercial synthetic granules (biphasic HA/-TCP (60% /40%)). The presence of bone formation not only around but also in the center of the major fault suggests that the porous nature of the ceramic possesses osteoinductive capabilities. Furthermore, as compared to standard synthetic grafts, these grafts had much higher bone mineral density (p 0.05). As a result, shark teeth are a promising source of bone grafts, possessing osteoinductive qualities and a moldable resorption level that no other biological bone graft has yet commercialized [Citation97].
Table 3. List of Marine source-derived bio-ceramic xenografts available in market.
Marine-based collagen scaffolds in bone tissue engineering
Osteoconductive collagen (mostly type I) and carbonate-substituted HAp comprise the majority of skeletal bones. As a result, an implant composed of such components is more likely than a monolithic device to replicate and utilize many components. When collagen, HAp, and other natural and synthetic macromolecules are combined, osteoblast growth and osteogenesis are stimulated and accelerated. In contrast, cell-based bone tissue engineering research necessitates the selection of an appropriate scaffold matrix. According to Liu and Sun, Impact of Marine-Based Biomaterials on the Immunoregulatory Properties of Bone Marrow-Derived Mesenchymal Stem Cells: Fish Collagen as a Potential Bone Tissue Engineering bio-material [Citation98] The use of synthetic graft materials and scaffolds that facilitate ex vivo bone tissue growth is limited in current bone regeneration procedures. As a result, biomimetic approaches to evaluating naturally occurring porous structures and employing them as templates for bone formation have grown in importance. Corals, algae (diatoms), and sponges all feature mineralized porous structures, some of which are employed as bone graft materials (Biocoral, Pro Osteon 200 R, Pro Osteon 500 R, Algipore, and others), while others are still being studied. Despite its visibly calcified components, marine collagen has been acknowledged as a functionally useful bone grafting material. Collagen and its composite scaffolds are isolated, analyzed, and developed for in vitro and in vivo scaffolding. It’s a never-ending search to extract, analyze, and manufacture collagen and its composite scaffolds from marine sources for in vitro and in vivo application as biomedical materials for bone tissue synthesis. Marine sponges have a structure and chemical composition that matches the cancellous architecture of bone tissue as well as the complex canal system to produce a porous biomimetic environment that promotes osteogenesis in vitro. As a functional component of composite biomaterials, marine sponge collagen/spongin is functionally equivalent to vertebrate collagen. Previously, Chondrosia reniformis collagen was combined with silica templating to create hydrogels that aid in the adhesion, proliferation, and differentiation of human mesenchymal cells (hMSCs) into osteoblast-like cells [Citation99]. When compared to individual monocomponent bone substitutes, bi-, tri-, and multi-component composite scaffolds are becoming increasingly important in terms of retaining the biomimetic matrix’s physicochemical and physiofunctional properties. Because of their close closeness to the original bone, HAp and collagen composites (Col) can copy and replace skeletal bones. A three-component system composed of marine sponge collagen, HAp, and chitosan was also discovered to be a viable bioscaffold for bone tissue engineering tests. Jellyfish collagen scaffolds are becoming increasingly popular due of the lack of pollutants in their body parts. As a result, researchers are interested in obtaining collagen from jellyfish umbrellas or arms. Despite the fact that the integration of these collagens with other bio-macromolecules is still in its early stages, few attempts have been made to create bone tissue engineering scaffolds or devices. Previously, freeze-drying and cross-linking with 1-ethyl-3-3-dimethyl aminopropyl) carbodiimide hydrochloride (EDC) and N-hydroxy succinimide were used to create porous jellyfish collagen-based scaffolds (NHS). These scaffolds can be used to create bone tissue when combined with other bone augmentation materials such as HAp and chitosan. Furthermore, bone marker expression and calcium accumulation in jellyfish collagen can be employed to investigate MSC osteogenic development. Marine fish collagens are used in a range of biomedical applications. The potential of salmon skin collagen/HAp composite scaffolds has recently been studied. Using the biomimetic mineralization principle, salmon skin collagen/HAp composite scaffolds have recently been investigated for their potential utility in bone tissue engineering. The proposed scaffolds have interconnected porosity, high resorbability, suitable cyclic compression stability, and elastic mechanical properties. Human mesenchymal stem cells (hMSCs) can also attach to and grow on these scaffolds, allowing for osteogenic differentiation. Another work made biomimetic bioabsorbable composites of HAp and collagen powder (HA-C) from salmon bone and skin using dissolution–precipitation. When A-C powders were implanted into the dorsal subcutaneous tissue of rats, collagen was totally absorbed and body fluid was infiltrated into big agglomerated particles, and bioabsorption by multi gigantic cell infiltration around the surface layers of HAp particles was seen [Citation100].
Scope for the future
Bone tissue reconstruction using marine sources offers promising clinical data as observed in the table above. However, it will be interesting to unfold its efficacy when used or mixed with another grating material or blood concentrates. One of the major shortcomings related to cases of marine sources is their compactness which avoids neo-angiogenesis and pericyte cells to initiate the regenerative process. The addition of the latest blood concentrates or growth factors might improve its property comparable to the gold standard graft.
Conclusions
Marine sources are enriched with wide sources of diversity with abundant biomaterial for the potential use of mankind. The elective choice of grafting materials offers a regenerative goal for reconstructive cases. Besides, they are cost-effective, and biologically acceptable with minimized chances of graft rejection and disease transmission. One of the remarkable features is the procurement which is adequate for the large defect sizes that may help the shortcomings of autogenous grafting material. Thus, unfolding new scope of better alternatives in the field of tissue regeneration.
List of Abbreviations
Ca2+ - Calcium ion
c-Fms- Colony-stimulating factor-1 receptor
cAMP- Cyclic adenosine monophosphate
CREB- cAMP response element-binding protein
eNOs- Endothelial nitric oxide synthase
EP1 – Early expressed protein 1
EP2- Early expressed protein 2
EP3- Early expressed protein 3
M-CSF- Macrophage colony-stimulating factor
OPG- Osteoprotegerin
NO- Nitric oxide
PTH- Parathyroid hormone
PTH1R- Parathyroid hormone 1 receptor
PKA- Protein kinase A
PLC-γ – Phospholipases Cγ
RANK- Receptor activator of nuclear factor kappa B
RANKL- Receptor activator of nuclear factor kappa-B ligand
RUNX2- Runt related transcription factor-2
1α,25(OH)2D3 −1α,25 Dihydroxy vitamin D3
α3GTP - α3 Guanosine triphosphate
Author contributions
SB conceptualizes the methodology. GN perfume the data collection, analysis, validation, and preparation. RB supported the scientific discussions. AS, DK and AK supported during the validation and preparation of the final manuscript. All the authors contribute to the final manuscript.
Acknowledgments
The authors are grateful to Prof (Dr). Sudam Chandra Si, Dean, and Prof (Dr). Manoj Ranjan Nayak, President, Centre of Biotechnology, Siksha O Anusandhan University, for providing all facilities.
Disclosure statement
The following author(s) state that there is no financial or non-financial conflict of interest in the publishing of this paper.
References
- Yu J, Xia H, Teramoto A, et al. Res. A.The effect of hydroxyapatite nanoparticles on mechanical behavior and biological performance of porous shape memory polyurethane scaffolds. J Biomed Mater. 2018;106(1):244–254.
- Berthiaume F, Maguire T, Yarmush J, et al. Tissue engineering and regenerative medicine: history, progress, and challenges Annu. Rev Chem Biomol Eng. 2011;2:403–430.
- Cheng X, Shao Z, Li C, et al. Isolation, characterization and evaluation of collagen from jellyfish rhopilema esculentum kishinouye for use in hemostatic applications. PLoS One. 2017 12; e0169731. 10.1371/journal.pone.0169731.
- Langer R. Perspectives and challenges in tissue engineering and regenerative medicine. Adv Mater. 2009 21;21(32–33):3235–3236.
- Chaudhari A, Vig A, Baganizi, D.R K, et al. Future prospects for scaffolding methods and biomaterials in skin tissue engineering: a review. Int J Mol Sci. 2016;17. 10.3390/ijms17121974. 1974.
- Zheng Z, Yu C, Wei H. Injectable hydrogels as three-dimensional network reservoirs for osteoporosis treatment. Tissue Eng Part B Rev. 2021;27(5):430–454.
- Hing K, Philos A. Bone repair in the twenty-first century: biology, chemistry or engineering. A Math Phys Eng Sci. 2004;362(1825):2821–2850.
- Baroli B, Pharm J. Sci.From natural bone grafts to tissue engineering therapeutics: brainstorming on pharmaceutical formulative requirements and challenges. Journal of Pharmaceutical Sciences. 2009;98(4):1317–1375.
- Dimitriou R, Jones E, McGonagle D, et al. Bone regeneration: current concepts and future directions. BMC Med. 2011 9;9(1):66.
- Martin I, Suetterlin R, Baschong W, et al. Enhanced cartilage tissue engineering by sequential exposure of chondrocytes to FGF-2 during 2D expansion and BMP-2 during 3D cultivation. J Cell Biochem. 2001;83(1):121–128.
- Gibbs D,M, Vaezi M,Y, Oreffo, R,O S. Hope versus hype: what can additive manufacturing realistically offer trauma and orthopedicsurgery. Regen Med. 2014 9;9(4):535–549.
- Schultz G, White S, Mitchell, R M, et al. Science.Epithelial wound healing enhanced by transforming growth factor-alpha and vaccinia growth factor. Science (New York, N.Y.). 1987;235(4786):350–352.
- Moya M, Cheng L, M H, et al. The effect of FGF-1 loaded alginate microbeads on neovascularization and adipogenesis in a vascular pedicle model of adipose tissue engineering. Biomaterials. 2010 31. 2816–2826. 10.1016/j.biomaterials.2009.12.053
- Entezari A, Swain M, Gooding V, et al. A modular design strategy to integrate mechanotransduction concepts in scaffold-based bone tissue engineering. Acta.Biomater. 2020;118:100–112.
- Gregor A, Filová E, Novák M, et al. Designing of PLA scaffolds for bone tissue replacement fabricated by ordinary commercial 3D printer. J Biol Eng. 2017;11(1):31.
- Januariyasa I, Ana K, I D, et al. Biol. Appl.Nanofibrous poly(vinyl alcohol)/chitosan contained carbonated hydroxyapatite nanoparticles scaffold for bone tissue engineering. Mater Sci Eng C Mater. 2020;107:110347.
- Hoarau-Véchot J, Rafii A, Touboul C, et al. Halfway between 2D and animal models: are 3D cultures the ideal tool to study cancer-microenvironment interactions. Int J Mol Sci. 2018;19(1):181.
- Duval K, Grover H, Han L,H, et al. Modeling physiological events in 2D vs. 3D cell culture. Physiology Bethesda. 2017;32:266–277.
- Sachlos E, Czernuszka J, Eur T. Making tissue engineering scaffolds work. Review: the application of solid freeform fabrication technology to the production of tissue engineering scaffolds. Cell. Mater. 2003;5:29–40.
- Augustyniak J, Bertero A, Coccini T, et al. Organoids are promising tools for species-specific in vitro toxicological studies. Journal of Applied Toxicology: JAT. 2019;39(12):1610–1622.
- Diaz-Rodriguez P, López-Álvarez M, Serra J, et al. Current stage of marine ceramic grafts for 3D bone tissue regeneration. Mar Drugs. 2019;17(8):471.
- Nayak G, Bhuyan, R S, Sahu A, et al. Review on biomedical applications of marine algae-derived biomaterials. Univers J Public Health. 2022;10(1):15–24.
- Ohlstein B, Kai T, Decotto E, et al. The stem cell niche: theme and variations. Curr Opin Cell Biol. 2004;16(6):693–699.
- Houacine C, Yousaf SS, Khan I, et al. Potential of natural biomaterials in nano-scale drug delivery. Curr Pharm Des. 2018;24(43):5188–5206.
- Ciuffi S, Zonefrati R, Brandi M, et al. Adipose stem cells for bone tissue repair. Cases Miner Bone Metab. 2017;14:217–226.
- Gao S, Zhao P, Lin C, et al. Differentiation of human adipose-derived stem cells into neuron-like cells which are compatible with photocurable three-dimensional scaffolds. Tissue Engineering. Part A. 2014;20(7–8):1271–1284.
- Bunnell B, Flaat A, Gagliardi M, et al. Methods.Adipose-derived stem cells: isolation, expansion and differentiation. Methods (San Diego, Calif.). 2008;45(2):115–120.
- Shafaei H, Kalarestaghi H. Adipose-derived stem cells: an appropriate selection for osteogenic differentiation. Journal of Cellular Physiology. 2020;235(11):8371–8386.
- Aggarwal S, Pittenger M,F. Human mesenchymal stem cells modulate allogeneic immune cell responses. Blood. 2005;105:1815–1822.
- O’Keefe R,J, Mao J. Bone tissue engineering and regeneration: from discovery to the clinic–an overview. Tissue. Eng. Part. B. Rev. 2011 17; 389–392. 10.1089/ten.TEB.2011.0475.
- Rophael J, Craft A, R O, et al. Angiogenic growth factor synergism in a murine tissue engineering model of angiogenesis and adipogenesis. Am.J.Pathol. 2007;171(6):2048–2057.
- Gothard D, Smith E, Kanczler L, et al. Tissue engineered bone using select growth factors: a comprehensive review of animal studies and clinical translation studies in man. Eur Cell Mater. 2014;28:166–208.
- Fedorovich N, Alblas, J E, Hennink W,E, et al. Organ printing: the future of bone regeneration. Trends.Biotechnol. 2011;29(12):601–606.
- Tang D, Tare R, Yang S, et al. Biofabrication of bone tissue: approaches, challenges and translation for bone regeneration. Biomaterials. 2016;83:363–382.
- Holesh J, Bass E, A N, et al. Physiology, Ovulation. In: Stat.Pearls. Treasure Island (FL): StatPearls Publishing; 2021.
- DiCorleto P, Bowen-Pope, DF E. Cultured endothelial cells produce a platelet-derived growth factor-like protein. Proc Natl Acad Sci U S A. 1983;80(7):1919–1923.
- Buckley A, Davidson J, Kamerath M, et al. U. S. A. Sustained release of epidermal growth factor accelerates wound repair. Proc Natl Acad Sci. 1985;82(21):7340–7344.
- Kim D, Lee B, Thomopoulos S, et al. Nat Commun.The role of confined collagen geometry in decreasing nucleation energy barriers to intrafibrillar mineralization. Nature Communications. 2018;9(1):962.
- Young MF. Int. Bone matrix proteins: their function, regulation, and relationship to osteoporosis. Osteoporos. 2003;14(3):S35–S42.
- Bonjour J,P. Nutritional disturbance in acid-base balance and osteoporosis: a hypothesis that disregards the essential homeostatic role of the kidney. Br J Nutr. 2013;110(7):1168–1177.
- Coppola D, Oliviero M, Vitale G,A, et al. Marine collagen from alternative and sustainable sources: extraction, processing and applications. Mar Drugs. 2020;18:214.
- Singh R, Kumar M, Mittal A, et al. Microbial enzymes: industrial progress in 21st century. Biotech. 2016;6(3):174.
- Ivankovic H, Gallego F, Tkalcec G, et al. Med.Preparation of highly porous hydroxyapatite from cuttlefish bone. J Mater Sci Mater. 2009;20(5):1039–1046.
- Amna T. Valorization of Bone Waste of Saudi Arabia by Synthesizing Hydroxyapatite. Appl.Biochem.Biotechnol. 2018;186(3):79–788.
- Venkatesan J, Lowe B, Manivasagan P, et al. Isolation and Characterization of Nano-Hydroxyapatite from Salmon Fish Bone. Materials Basel. 2015;8:5426–5439.
- Zhu Y, Goh C, Shrestha A. Biomaterial properties modulating bone regeneration. Shrestha A. Macromol.Biosci. 2021;21(4):e2000365.
- Guillemin G, Patat J, Fournie L, et al. The use of coral as a bone graft substitute. J Biomed Mater Res. 1987;21(5):557–567.
- Schröder H, Wang C, Tremel X, et al. Biofabrication of biosilica-glass by living organisms. Nat Prod Rep. 2008;25(3):455–474.
- Wang S, Wang X, Draenert F,G, et al. Bioactive and biodegradable silica biomaterial for bone regeneration. Bone. 2014;67:292–304.
- Weeks R, Russ G, Alcala R, et al. Effectiveness of marine protected areas in the Philippines for biodiversity conservation. Conserv Biol. 2010;24(2):531–540.
- Petrenko I, Bazhenov V, Galli, R V, et al. Chitin of poriferan origin and the bioelectrometallurgy of copper/copper oxide. Int J Biol Macromol. 2017;104(Pt B):1626–1632.
- Perić K, Ž R, Alkildani, S P, et al. An introduction to bone tissue engineering. Int J Artif Organs. 2020;43(2):69–86.
- García-Enriquez S, Guadarrama H, Reyes-González, I E, et al. Mechanical performance and in vivo tests of an acrylic bone cement filled with bioactive sepia officinalis cuttlebone. J Biomater Sci Polym Ed. 2010;21(1):113–125.
- Palaveniene A, Harkavenko V, Kharchenko V, et al. Cuttlebone as a marine-derived material for preparing bone grafts. Biotechnol.(NY). Mar. 2018; 20: 363–374.
- Liu S, Li D, Chen X, et al. Surf .B.Biointerfaces.Biomimetic cuttlebone polyvinyl alcohol/carbon nanotubes/hydroxyapatite aerogel scaffolds enhanced bone regeneration. Colloids. 2021;112221. 10.1016/j.colsurfb.2021
- Palaveniene A, Harkavenko V, Kharchenko V, et al. Cuttlebone as a marine-derived material for preparing bone grafts. Biotechnol. NY. Mar. 2018;20:363–374.
- Hutmacher D,W. Biomaterials.Scaffolds in tissue engineering bone and cartilage. Biomaterials. 2000;21(24):2529–2543.
- Hu CH, Yao CH, Chan TM, et al. Effects of different concentrations of collagenous peptide from fish scales on osteoblast proliferation and osteoclast resorption. Chin. J Physiol. 2016 59; 191–201.
- Matsumoto R, Uemura T, Xu Z, et al. Rapid oriented fibril formation of fish scale collagen facilitates early osteoblastic differentiation of human mesenchymal stem cells. Biomed Mater Res. 2015;103(8):2531–2539.
- Langasco R, Cadeddu B, Formato M, et al. Natural collagenic skeleton of marine sponges in pharmaceutics: innovative biomaterial for topical drug delivery. Mater Sci Eng C Mater Biol Appl. 2017;70(Pt 1):710–720.
- Lin Z, Solomon K, Zhang, X L, et al. In vitro evaluation of natural marine sponge collagen as a scaffold for bone tissue engineering. Int J Biol Sci. 2011;7(7):968–977.
- Kolk A, Handschel J, Drescher W, et al. Current trends and future perspectives of bone substitute materials - from space holders to innovative biomaterials. J.Craniomaxillofac.Surg. 2012;40(8):706–718.
- Alakpa E, Burgess V, K E, et al. Topography produces higher crystallinity in bone than chemically induced osteogenesis. ACS. Nano Nacre. 2017 11; 6717–6727.
- Hammouche S, Khan W, Drouin H, et al. In vitro Evaluation of Natural Marine Sponge Collagen as a Scaffold for bone tissue engineering. Curr Stem Cell Res. 2012;7(5):336–346.
- Chen C, Rosi L, N L. Angew Peptide-based methods for the preparation of nanostructured inorganic materials. Chem Int Ed Engl. 2010;49(11):1924–1942.
- Shapiro O, Kramarsky-Winter H, Gavish E, et al. A coral-on-a-chip microfluidic platform enabling live-imaging microscopy of reef-building corals. Nat.Commun. 2016;7(1):10860.
- Macha I, Ben-Nissan J. Marine skeletons: towards hard tissue repair and regeneration. Marine Drugs. 2018;16(225). DOI:10.3390/md16070225
- Farabegoli F, Blanco L, Rodríguez L, et al. Phycotoxins in marine shellfish: origin, occurrence and effects on humans. Mar Drugs. 2018;16:188.
- Chaturvedi R, Singha P, Dey, S K. Water soluble bioactives of nacre mediate antioxidant activity and osteoblast differentiation. PLoS One. 2013;8(12):e84584.
- Kim H, Lee K, Ko C,Y, et al. Biomaterials.The role of nacreous factors in preventing osteoporotic bone loss through both osteoblast activation and osteoclast inactivation. Biomaterials. 2012;33(30):7489–7496.
- Diogo G, Senra S, E L, et al. Marine collagen/apatite composite scaffolds envisaging hard tissue applications. Mar Drugs. 2018;16:269.
- Pallela R, Venkatesan J, Janapala V, et al. Biophysicochemical evaluation of chitosan-hydroxyapatite-marine sponge collagen composite for bone tissue engineering. J Biomed Mater Res A. 2012;100(2):486–495.
- Elango J, Zhang J, Bao B, et al. Rheological, biocompatibility and osteogenesis assessment of fish collagen scaffold for bone tissue engineering. Int J Biol Macromol. 2016;91:51–59.
- Parisi J, Fernandes R, K R, et al. Incorporation of collagen from marine sponges (spongin) into hydroxyapatite samples: characterization and in vitro biological evaluation. Mar Biotechnol (NY). 2019 21;21(1):30–37.
- Mredha M, T I, Kitamura N, et al. Anisotropic tough double network hydrogel from fish collagen and its spontaneous in vivo bonding to bone. Biomaterials. 2017;132:85–95.
- Bernhardt A, Paul B, Gelinsky M. Biphasic scaffolds from marine collagens for regeneration of osteochondral defects. Mar Drugs. 2018 16;16(3):91.
- Rigogliuso S, Salamone M, Barbarino E, et al. Production of injectable marine collagen-based hydrogel for the maintenance of differentiated chondrocytes in tissue engineering applications. Int J Mol Sci. 2020;21:5798.
- Yamada S, Yamamoto K, Nakazono A, et al. Functional roles of fish collagen peptides on bone regeneration. Dent Mater J. 2021;40(6):1295–1302.
- Codrea C, Croitoru I, A M, et al. Advances in osteoporotic bone tissue engineering. J Clin Med. 2021;10:253.
- Giardoglou P, Beis D. On zebrafish disease models and matters of the heart. Biomedicines. 2019;7:15.
- Cheung R, C N, T B, et al. Marine peptides: bioactivities and applications. Marine Drugs. 2015;13(7):4006–4043.
- Younes I, Rinaudo M. Chitin and chitosan preparation from marine sources. Structure, properties and applications. Mar Drugs. 2015 13;13(3):1133–1174.
- Ezoddini-Ardakani F, Navabazam A, Fatehi F, et al. Histologic evaluation of chitosan as an accelerator of bone regeneration in microdrilled rat tibias. Dent Res J. 2012;9:694–699.
- Liu H, Peng H, Wu Y, et al. Biomaterials.The promotion of bone regeneration by nanofibrous hydroxyapatite/chitosan scaffolds by effects on integrin-BMP/Smad signaling pathway in BMSCs. Biomaterials. 2013;34(18):4404–4417.
- Lee K, Mooney, D,J, Y. Alginate: properties and biomedical applications. Prog Polym Sci. 2012;37(1):106–126.
- Senni K, Pereira J, Gueniche F, et al. Marine polysaccharides: a source of bioactive molecules for cell therapy and tissue engineering. Marine Drugs. 2011;9(9):1664–1681.
- Kolambkar Y, Dupont M, K M, et al. Biomaterials.An alginate-based hybrid system for growth factor delivery in the functional repair of large bone defects. Biomaterials. 2011;32(1):65–74.
- Park S, Chun B, K R, et al. The differential effect of high and low molecular weight fucoidans on the severity of collagen-induced arthritis in mice. Phytother Res. 2010;24(9):1384–1391.
- Changotade S, Korb I, Bassil, J G, et al. Potential effects of a low-molecular-weight fucoidan extracted from brown algae on bone biomaterial osteoconductive properties. J Biomed Mater Res. 2008;87(3):666–675.
- Park S, Lee J, K W, et al. The sulfated polysaccharide fucoidan stimulates osteogenic differentiation of human adipose-derived stem cells. Stem Cells. 2012;21:2204–2211.
- Gao C, Peng S, Feng P, et al. Bone biomaterials and interactions with stem cells. Bone Res. 2017;5:17059.
- Elsayed H, Rincón Romero A, Ferroni L, et al. Bioactive glass-ceramic scaffolds from novel ‘inorganic gel casting’and sinter-crystallization. Mater. 2017;10(2):171.
- Webber M, Khan J, O F, et al. A perspective on the clinical translation of scaffolds for tissue engineering. Ann Biomed Eng. 2015;43(3):641–656.
- Messina A, Marini M, L O, et al. A step-by-step procedure for bone regeneration using calcium phosphate scaffolds: from site preparation to graft placement. E J Craniofac Surg. 2019;30(1):149–153.
- Macha I, Ben-Nissan J, Mar B. Marine skeletons: towards hard tissue repair and regeneration. Drugs. 225. 2018. 16 10.3390/md16070225.
- Sethmann I, Luft C, Kleebe H. Development of phosphatized calcium carbonate biominerals as bioactive bone graft substitute materials, part I: incorporation of magnesium and strontium ions. J J Funct Biomater. 2018;9(4):69.
- Diaz-Rodriguez P, López-Álvarez M, Serra J, et al. Current stage of marine ceramic grafts for 3D bone tissue regeneration. Mar Drugs. 2019 17;17(8):471.
- Liu C,S, Sun J. Impact of marine-based biomaterials on the immunoregulatory properties of bone marrow-derived mesenchymal stem cells: potential use of fish collagen in bone tissue engineering. J. ACS. Omega. 2020;20(43):2836.
- Hoyer B, Bernhardt A, Heinemann S, et al. Biomimetically mineralized salmon collagen scaffolds for application in bone tissue engineering. M Biomacromolecules. 2012;13(4):1059–1066.
- Pallela R, J V, V R, et al. Biophysicochemical evaluation of chitosan hydroxyapatite-marine sponge collagen composite for bone tissue engineering. Kim J Biomed Mater Res A. 2012;100(2):486–495.
- Sun F, Zhou H, Lee J. Various preparation methods of highly porous hydroxyapatite/polymer nanoscale biocomposites for bone regeneration. Acta.Biomater. 2011;7(11):3813–3828.
- Kim S, Park E, Adv K. Recent advances of biphasic calcium phosphate bioceramics for bone tissue regeneration Exp. Med Biol. 2020;1250:177–188.
- Marie B, Marin F, Marie A, et al. Evolution of nacre: biochemistry and proteomics of the shell organic matrix of the cephalopod Nautilus macromphalus. Chembiochem: a European Journal of Chemical Biology. 2009;10(9):1495–1506.
- Sanz-Herrera J, Reina-Romo, E A. Cell-biomaterial mechanical interaction in the framework of tissue engineering: insights, computational modeling and perspectives. Int J Mol Sci. 2011;12(11):8217–8244.
- Lee K, Mooney Y, D J. Alginate: properties and biomedical applications. Prog Polym Sci. 2012;37(1):106–126.
- Shahidi F, Abuzaytoun R. Chitin, chitosan, and co-products: chemistry, production, applications, and health effects. Adv Food Nutr Res. 2005 49; 93–135. 10.1016/S1043-4526(05)49003-8.
- Fitton JH. Therapies from fucoidan; multifunctional marine polymers. Mar Drugs. 2011;9(10):1731–1760.
- Ivankovic H, Gallego F, Tkalcec G, et al. Preparation of highly porous hydroxyapatite from cuttlefish bone. J Mater Sci Mater Med. 2009;20(5):1039–1046.