ABSTRACT
Breast cancer belongs to diseases with the highest mortality rates. Euphorbia hirta is an herbal plant with various pharmacological properties and is predicted to have good anti-breast cancer properties. This current study analyzed a potent compound from E. hirta as an anti-breast cancer candidate and predicted its mechanism. The Bioactive compounds in E. hirta were screened for drug-likeness, toxicity, membrane permeability, and bioactivity. Protein target prediction utilized four databases, followed by functional annotation analysis. Molecular docking employed AutoDock Vina, and molecular dynamic simulation used Yet Another Scientific Artificial Reality Application with parameters reflecting human cell physiology. Kaempferol was the potent compound in E. hirta that passed the screening and was predicted to have better anti-breast cancer activity than that of other compounds. Kaempferol targets AKT1 and ERα, the proteins correlating with breast cancer progression. The molecular docking results showed that Kaempferol bound to inhibitor sites on both AKT1 and ERα. In addition, Molecular dynamic analysis showed that the interaction between Kaempferol with AKT1 and ERα was stable, as seen from the RMSD, RMSF, hydrogen bonding, and MM/PBSA binding energy values. Therefore, Kaempferol was a bioactive compound from E. hirta with promising anti-breast cancer activities by targeting the AKT1 and ERα.
Introduction
Breast cancer ranks first in global cancer occurrence, with approximately 2.26 million documented cases annually, constituting 11.7% of the total reported cancer cases and 24.5% of cancers diagnosed in women [Citation1]. In 2023, solely within the United States, it is estimated that there will be 1,958,310 new cancer cases and 609,820 cancer-related deaths [Citation2]. It is estimated that one in eight women worldwide will be diagnosed with breast cancer [Citation3]. The highest mortality rate is in low-income countries because it has various medical limitations [Citation4]. Breast cancer occurs due to the dysregulation of pathways related to cell proliferation and cell survival [Citation5].
The PI3K/Akt and Estrogen Receptor (ER) signaling pathways are commonly dysregulated in breast cancer cells [Citation6,Citation7]. The PI3K/Akt signaling pathway relates to the induction of proliferation and survival of tumor cells [Citation8]. This pathway is activated when receptor tyrosine kinases, such as IGF1R, EGFR, HER2, and HER3, bind to their ligands. The activated RTK phosphorylates PI3K, then PI3K activates the AKT1 protein. AKT1 activates the signaling cascade, resulting in the expression of genes related to cell growth and proliferation [Citation7]. In breast cancer, the PI3K/Akt pathway experiences overactivity due to the overexpression of proteins and mutations in the pathway [Citation9]. Mutations in the AKT1 are frequently found in patients with breast cancer. This mutation causes AKT1 to be overactivated, upregulating the PI3K/Akt signaling pathway [Citation10]. The ER signaling pathway also contributes to breast cancer progression. ERα and ERβ are involved in the ER signaling pathway, but only ERα plays a role in cancer cell progression. ERα is activated after binding to estrogen and then translocates to the nucleus to become a transcription factor for proliferation and metastasis-related genes [Citation11]. In most breast cancer cases, ERα is overexpressed, thus triggering the proliferation and metastasis of tumor cells [Citation12]. Inhibition of PI3K/Akt and ER signaling pathways is an accurate strategy to inhibit breast cancer progression.
Euphorbia hirta is an herb with a high potential for anti-breast cancer. E. hirta is a plant of the Euphorbiaceae family. It spread to several countries in Asia and Australia [Citation13]. Previous research stated that crude extract of E. hirta has good anticancer activity in the lungs, liver, and leukemia through in vitro studies [Citation14–16]. The activity of the E. hirta extract is influenced by the bioactive compounds contained therein. Previous studies have stated that Guava contains compounds with good pharmacological activity, such as rutin, quercetin, Kaempferol, etc [Citation13]. However, no studies have revealed which compounds in E. hirta have the highest anticancer activity. Identifying which compounds in E. hirta have the best anti-breast cancer properties will be useful for drug development. In the present study, an in-silico study was performed to screen the bioactive compound of E. hirta that has the most potential as an anti-breast cancer with a predictable mechanism.
Method
Data mining of compounds contained in Euphorbia hirta
The compounds contained in E. hirta were retrieved from previous studies [Citation17,Citation18]. Canonical SMILES of compounds contained in E. hirta were obtained from the PubChem database (https://pubchem.ncbi.nlm.nih.gov/).
Screening for drug-likeness, toxicity, membrane permeability, and bioactivity
Drug-likeness screening was conducted using the SWISS ADME web server (http://www.swissadme.ch/index.php) with the parameters used, namely Lipinski, Veber, and Egan [Citation19]. Compounds passing the drug-likeness screening were followed by toxicity screening using the ProTox web server (https://tox-new.charite.de/protox_II/) to select nontoxic compounds for humans [Citation20]. Membrane permeability screening was performed using the PerMM web server (https://permm.phar.umich.edu/) to select compounds that could easily pass through the lipid bilayer [Citation21]. Compounds that passed the above screenings were continued for bioactivity screening using the PASS Online webserver (http://www.way2drug.com/passonline/) [Citation22]. The parameters used were related to breast cancer progression.
Target protein determination
The direct protein targets of Kaempferol compounds were determined using the SWISS Target Prediction (http://www.swisstargetprediction.ch/), STITCH (http://stitch.embl.de/), and CTD (http://ctdbase.org) databases [Citation23–25]. The selected target proteins were related to breast cancer progression according to the cBioportal database (https://www.cbioportal.org/). Indirect protein targets were determined using the STRING database (https://string-db.org/). Target protein visualization was performed using Cytoscape 3.7.2 software.
Functional annotation
Functional annotation was performed to group target proteins based on their role in the cell. Functional annotation analysis was performed using the DAVID web server (Database for Annotation, Visualization, and Integrated Discovery) (https://david.ncifcrf.gov/) [Citation26]. The databases used were Gene Ontology (GO) and the Kyoto Encyclopedia of Genes and Genomes (KEGG pathway). GO would group proteins into three domains: Biological Process, Cellular Component, and Molecular Function. The KEGG pathway will classify target proteins based on their role in human cellular pathways.
Ligand preparation
The 3D structure of Kaempferol was obtained from the PubChem database (https://pubchem.ncbi.nlm.nih.gov/). The inhibitors for each protein were retrieved from the protein pdb file. All ligands were prepared by minimizing the conformational energy using the Open babel plugin integrated into the PyRx 8.0 software [Citation27].
Protein preparation
The 3D structures of the AKT1 (PDB ID: 6hhf) and ERα (PDB ID: 3ert) proteins were obtained from the RCSB PDB database (https://www.rcsb.org/). The proteins were prepared by removing water molecules and contaminant ligands using the Biovia Discovery Studio 2019 software (Dassault Systèmes Biovia, San Diego, California, USA).
Molecular docking
Specific docking was performed on the active sites of AKT1 and ERα proteins using the AutoDock Vina tools integrated with PyRx 8.0 [Citation28–30]. The grid coordinates for specific docking are in . The docking result with the lowest binding affinity value was selected. Docking results were visualized using the Discovery Studio Visualizer 2019 to analyze the binding pose and chemical interaction.
Table 1. Grid coordinate for specific docking.
Molecular dynamic simulation and binding energy evaluation
Molecular dynamics simulations were conducted using Yet Another Scientific Artificial Reality Application version 19.12.14 software with the AMBER14 force field [Citation31]. Conditions in the system were regulated according to the human’s physiological conditions, such as temperature 310K, pH 7.4, salt content 0.9%, and pressure 1 bar. The simulation lasted for 100 ns with autosave every 25 ps. The Root-Mean-Square Deviation (RMSD) values and the number of protein hydrogen bonds were analyzed using the md_analyze macro program. The Root-Mean-Square-Fluctuation (RMSF) was analyzed using the md_analyzeres macro program. The binding free energy of the protein and ligand complexes was evaluated using the Molecular Mechanics Poisson – Boltzmann Surface Area (MM/PBSA) method with 0.65 kJ/mol/Å2 surface tension and omitting the entropy term [Citation32]. The binding free energy was calculated every 24 ps after equilibrium. The binding pose of the ligand was also evaluated every 20 ns after equilibrium.
Result
Screening based on drug-likeness, toxicity, membrane permeability, and bioactivity
This screening identified the compounds with the best anti-breast cancer activity. Drug-likeness screening showed that ten compounds had no violation in the Lipinski, Veber, and Egan parameters. Therefore, these ten compounds passed the drug-likeness selection (). The ten compounds underwent toxicity screening to identify nontoxic compounds for the human body. The screening results identified five nontoxic compounds for humans: epicatechin, chrysin, syringic acid, Kaempferol, and quercitol (). The five compounds continued to the selection based on membrane permeability. The results showed that all compounds could penetrate the plasma membrane (). The five compounds were continued for bioactivity screening. The results of the bioactivity screening showed that Kaempferol had a high Pa value for almost all bioactivity parameters (). Therefore, it is predicted that Kaempferol has the best anticancer activity in E. hirta. The two-dimensional structure of Kaempferol can be seen in .
Figure 1. Drug-likeness and toxicity screening. a) drug-likeness screening using Lipinski, Veber, and Egan rules. b) toxicity examination based on LD50 and the probability of inducing toxicity.
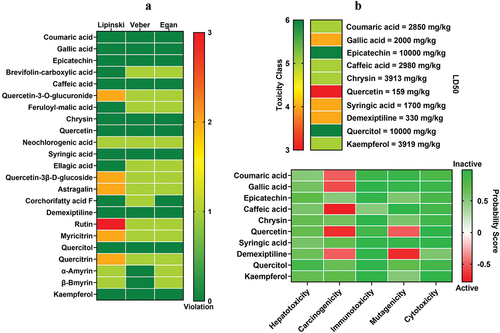
Figure 2. Screening of membrane permeability and bioactivity. a) simulation of the chrysin, epicatechin, Kaempferol, quercitol, and syringic acid penetrating the lipid bilayer. b) the energy transfer value of the six compounds penetrating the phospholipid membrane. c) bioactivity screening. d) the 2D structure of the Kaempferol.
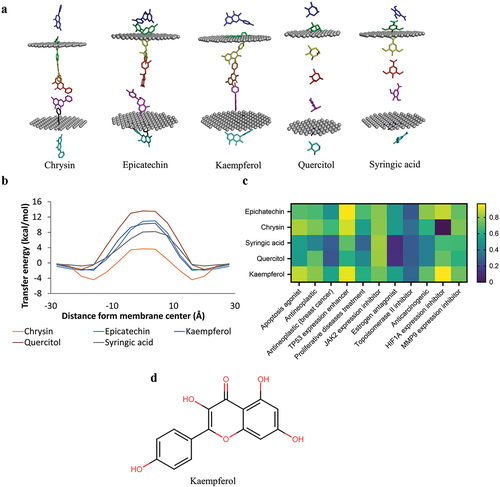
Target protein prediction and functional annotation
Based on three databases, the direct targets of Kaempferol were ABCC1, AHR, CYP1B1, MMP2, ALOX12, ESR2, ESR1, CDK1, and AKT1 (). The nine proteins’ relation to patients’ breast cancer progression were analyzed using cBioportal. The analysis showed that AKT1 and ESR1(ERα) proteins were most altered in patients with breast cancer (). The proteins interacting with AKT1 and ERα can be seen in . Functional annotation analysis showed that all Kaempferol’s direct and indirect target proteins were involved in PI3K-Akt, FoXo, and JAK-STAT signaling pathways, including other pathways related to cancer cell growth. According to the GO database, the target proteins are involved in biological processes related to proliferation and cell survival ().
Figure 3. Prediction of protein targets and functional annotation. a) prediction of the direct target of the Kaempferol in the nine protein targets. b) analysis of target proteins with cBioportal found AKT1 and ERα proteins to be involved in breast cancer progression. c) proteins interacting with AKT1 and ERα proteins. d) functional annotation of target proteins.
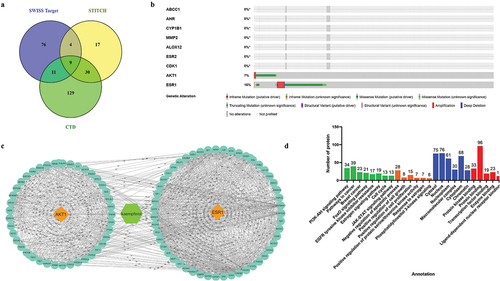
Interaction between Kaempferol and AKT1 and ERα
Molecular docking results showed that Kaempferol bound to AKT1 and ERα at the same site as an inhibitor with a low binding affinity value. The interaction between Kaempferol and AKT1 resulted in a binding affinity value of −9.2 kcal/mol. Kaempferol bound to AKT1 by forming three hydrogen bonds and four hydrophobic interactions. The interaction of Kaempferol on AKT1 protein residues had similarities with the interaction of inhibitors with AKT1, namely Ser205, Trp80, Leu264, and Val270 ( and ). The interaction between Kaempferol and ERα had a binding affinity value of −8.2 kcal/mol. Kaempferol interacted with ERα by forming one hydrogen bond and seven hydrophobic interactions. Kaempferol bound to ERα at the same residue as inhibitors Leu387, Ile424, Met421, Leu346, Ala350, Leu387, Met388, and Leu525 ( and ). The docking results predicted that Kaempferol could be a competitive inhibitor of AKT1 and ERα proteins. Next, the stability of the interaction between Kaempferol and AKT1 and ERα was analyzed by MD simulation.
Figure 4. 3D and 2D visualization of protein-ligand interactions. a) interaction between AKT1-inhibitor and AKT1-Kaempferol. b) interaction between ERα-inhibitor and ERα-Kaempferol.
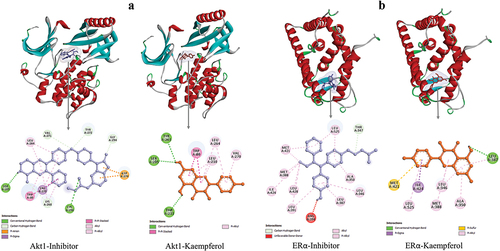
Table 2. Chemical interaction between Akt1 and ERα with the ligands.
The stability of the interaction between Kaempferol and AKT1 and ERα
MD simulation was used to analyze the stability of protein structure and the stability of protein-ligand interactions. After interacting with Kaempferol, the RMSD backbone parameter analyzed the structural stability of the AKT1 and ERα proteins, the number of hydrogen bonds, and the RMSF value. The RMSD ligand movement and binding energy analyzed the stability of the interaction between AKT1 and ERα with Kaempferol. The simulation results showed that the RMSD backbone value of the AKT1-Kaempferol complex is below 3Å (). The number of AKT1 hydrogen bonds interacting with Kaempferol was similar to that of AKT1 interacting with inhibitors (). The RMSF values of AKT1 were also mostly below 3Å (). The simulation results indicate that the structure of AKT1 binding to Kaempferol is stable during the simulation. The RMSD ligand movement represents the movement of the ligand during the simulation. The simulation showed that the RMSD ligand movement value at 60–100 ns had minimal fluctuations (). This indicated stable Kaempferol binding to AKT1 from the 60th ns. The MM/PBSA binding energy represents the stability of protein-ligand interactions. The AKT1-Kaempferol complex had a low binding energy value () and was stable until the end of the simulation (). This showed that the AKT1-Kaempferol interaction was stable for 100 ns.
Figure 5. Simulation of the molecular dynamics of the interaction between AKT1 and the ligand. a) RMSD backbone proteins. b) RMSD ligand movement. c) number of hydrogen bonds of the AKT1 protein. d) MM/PBSA binding energy values of the AKT1-inhibitor and AKT1-Kaempferol complexes. e) RMSF values of AKT1-inhibitor and AKT1-Kaempferol complexes.
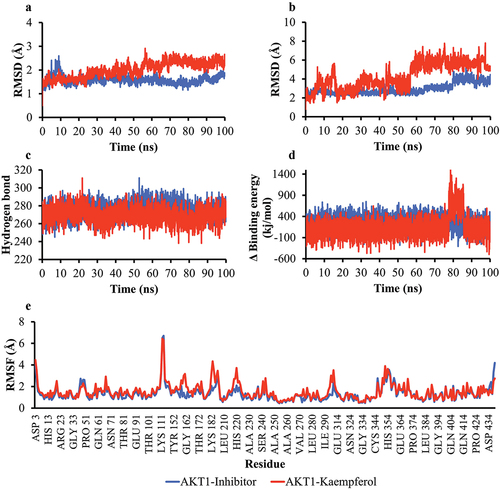
Table 3. Calculations on the binding free energy of the complexes.
The simulation results on the ERα-Kaempferol complex also showed good stability. The RMSD backbone value was below 3Å and had minimal fluctuation (). The number of hydrogen bonds in the ERα-Kaempferol complex was similar to that of the ERα-inhibitor (). The RMSF values were also mostly below 3Å, indicating that most of the amino acids moved slightly during the simulation (). The RMSD value of the ligand movement of the ERα complex had minimal fluctuations from 4 ns until the end of the simulation (). The ERα-Kaempferol complex had a more stable MM/PBSA binding energy than the ERα-inhibitor complex (). The simulation results showed that the interaction between ERα and Kaempferol is stable.
Figure 6. Simulation of the molecular dynamics of the interaction between ERα and ligands. a) RMSD backbone proteins. b) RMSD ligand movement. c) number of hydrogen bonds of the Akt1 protein. d) MM/PBSA binding energy value of ERα-inhibitor and ERα-Kaempferol complexes. e) RMSF values of ERα-inhibitor and ERα-Kaempferol complexes.
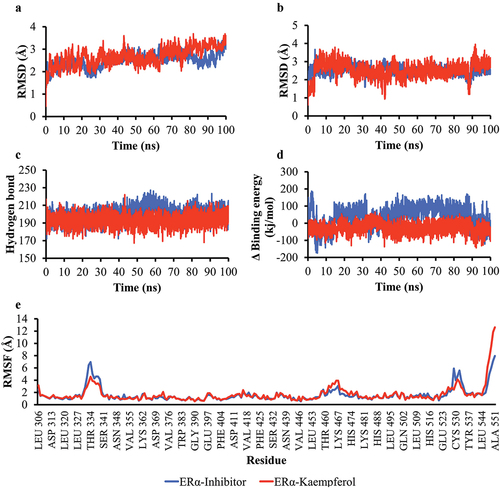
Changes of Kaempferol binding pose seen from hydrogen bond position
MD simulation also analyzed the position of Kaempferol’s hydrogen bonds in proteins. During the simulation, there was a change in the position of the hydrogen bond in the interaction between Kaempferol and AKT1. At 0 ns, Kaempferol formed three hydrogen bonds with Thr211, Ser205, and Thr263. At the 25th, 50, 70, and 100 ns times, the positions of the hydrogen bonds were alternated. At 50 ns, Kaempferol did not form hydrogen bonds with AKT1, resulting in a high binding energy value. At 75 and 100 ns, Kaempferol formed hydrogen bonds with Lys268 again (). Changes in hydrogen bond positions also occurred in Kaempferol and Erα interactions. At 0 ns, Kaempferol only formed hydrogen bonds with Leu387. At 5 to 100 ns, Kaempferol formed more hydrogen bonds, namely in Glu419 and Glu353, so the binding energy value was stable ().
Discussion
E. hirta contains pharmacologically important bioactive compounds. This study will predict only which candidate compounds had the highest anti-breast cancer potential. The bioactive compounds from E. hirta were screened with drug-likeness parameters, toxicity, membrane permeability, and bioactivity to obtain compounds with the most potent anti-breast cancer activity. The screening results showed that the compound in E. hirta with the most potential as an anticancer agent was Kaempferol.
Kaempferol (3,5,7-trihydroxy-2-(4′-hydroxyphenyl)-4 H-chrome-4-one; ) is a flavonoid with high antioxidant activity [Citation33]. Kaempferol has a 3-OH in the aromatic ring and a 5,7-dihydroxy group in ring A. It has relatively large three-ring planar and hydrophobic aromatic systems and a double bond between C2–C3 in ring C. This structure allows Kaempferol to have a high affinity for the lipid bilayer [Citation34]. The structure of Kaempferol has two intramolecular hydrogen bonds, which add to the stability of the structure. Kaempferol has a high polarity, allowing it to bind strongly to proteins [Citation35]. The structure of Kaempferol causes the compound to have various pharmacological activities. Kaempferol can significantly inhibit Nitric Oxide (NO) production and tumor necrosis factor-alpha (TNF-α) expression in LPS-stimulated RAW cells [Citation36]. Kaempferol also has high antiproliferative activity in HepG2, CT26, and B16F1 cells and can inhibit NO release [Citation37]. This compound can inhibit the PI3K/Akt pathway in liver and lung cancer [Citation38]. This study states that Kaempferol has a high potential as an AKT1 and ERα inhibitor.
Kaempferol is predicted to have good anticancer activity. The drug-likeness analysis stated that Kaempferol had no violation in the Lipinski, Veber, and Egan parameters. Compounds passing the drug-likeness analysis have drug-like characteristics: Dissolve easily in the blood, penetrate cell membranes, and are easily metabolized and transported [Citation39]. Kaempferol is also nontoxic to humans, as seen from the results of the toxicity analysis using ProTox. ProTox II uses the QSAR principle in analyzing the test compounds’ toxicity, so it can be said that the Kaempferol does not have a structural resemblance to the toxic compounds found previously [Citation20]. Kaempferol can also penetrate the plasma membrane easily based on membrane permeability analysis with perMM (). The permeability of the membrane of a compound is affected by the transfer of energy. Energy transfer is the energy required to pass through the plasma membrane; the energy transfer value will change according to the position of the compound in the lipid bilayer [Citation21]. Hydrophobic molecules have the highest energy transfer values in the middle and lowest of the ends of the lipid bilayer. Hydrophobic molecules have the lowest energy transfer in the middle and the highest at the edges [Citation40]. In addition, based on the PASS Online bioactivity analysis, Kaempferol has good anti-breast cancer activity. PASS Online predicts the bioactivity of a query compound with the QSAR principle with a minimum Pa (Probable to be active) value of more than 0.3, which means it has a certain bioactivity. High values of Pa mean a high possibility of certain bioactivity of a compound [Citation22].
Kaempferol could stably bind to AKT1 and ERα proteins based on molecular docking and dynamics. Kaempferol binds to AKT1 and ERα at the inhibitor site and has a low binding affinity value. A low binding affinity value leads to a strong interaction between the protein and the ligand [Citation41]. Kaempferol also forms hydrogen bonds, which help stabilize interactions [Citation42]. The AKT1 and ERα protein structures were still stable after interacting with Kaempferol, as indicated by the RMSD backbone value, the number of hydrogen bonds, and RMSF. RMSD backbone calculates the average displacement of each atom in the protein backbone during the simulation. The protein structure is stable if the backbone RMSD value is below 3 Å [Citation43]. The number of hydrogen bonds greatly influences the stability of the protein structure because hydrogen bonds form the secondary structure of the protein [Citation44]. In more detail, the stability of protein structure can be seen through RMSF.
RMSF calculates the fluctuation of each amino acid in a protein. If most amino acids have RMSF values below 3 Å, then the protein structure is stable [Citation45]. In addition to the stable structure of AKT1 and ERα proteins. The interaction between Kaempferol and AKT1 and ERα is also stable, as seen from the RMSD ligand movement and MD binding energy values. RMSD ligand movement represents the movement of the ligand during the simulation. RMSD values with minimal fluctuations indicate that the ligand is stable when it binds to a protein [Citation46]. MM/PBSA binding energy represents the binding energy value of the ligand in the protein. A low binding energy value means a stable interaction between the protein and the ligand [Citation47]. Kaempferol can bind stably to AKT1 and ERα proteins and is highly predicted to become an inhibitor of these proteins.
AKT1 is a central protein in the PI3K/Akt signaling pathway. The AKT1 protein has a size of ~ 55.9 kDa comprising three domains: the N-terminal pleckstrin homology, kinase, and regulatory [Citation48]. AKT1 activation occurs when Thr308 and Ser473 residues are phosphorylated by PDK1, when PI3K has been activated. After that, AKT1 binds to the substrate, and the ATP molecule, AKT1, transfers phosphate from ATP to the substrate, activating the substrate [Citation49]. The substrates of the AKT1 protein are TSC (Tuberous Sclerosis Complex) and Mdm2 (Mouse double minute 2 homolog). Phosphorylated TSCs become inactive and lose their ability to suppress mTORC1. mTORC1 activity is related to tumor cell growth. Mdm2 activated by AKT1 will inhibit p53 activity, thereby inducing tumor cell survival [Citation50]. Therefore, inhibiting the AKT1 protein will inhibit the activation of proteins related to cancer progression [Citation51].
ERα is an intracellular receptor protein and an intracellular transcription factor involved in regulating the expression of certain genes after capturing estrogen. ERα has a molecular weight of 65 kDa and 595 amino acids. This protein is divided into three: N-terminal domain (NTD), DNA binding domain (DBD), and Ligand binding domain (LBD) [Citation52]. ERα activation occurs when LBD binds to estrogen, which then ERα forms a dimer. The ERα dimer translocates to the nucleus and acts as a transcription factor [Citation53]. DBD from ERα will bind to the Estrogen Response Element in the 5′-GGTCAnnnTGACC-3′ sequence [Citation54]. One of the target genes of ERα is CCND1, which regulates the cell cycle [Citation55]. In breast cancer, ERα is overexpressed due to increased promoter activity of the ERα gene (ESR1), amplified the ESR1 gene, and inhibited ERα degradation through ubiquitination and proteasomal pathways [Citation56]. The inhibition of ERα, a key growth factor for cancer cells, has demonstrated its effectiveness in suppressing the proliferation of breast cancer cells [Citation57].
This study suggested that Kaempferol has promising anti-breast cancer properties. It is predicted that high Kaempferol can inhibit the activity of AKT1 and Erα proteins, which are involved in breast cancer progression. However, these in-silico predictions must be validated using in vitro and in vivo approaches. The discovery of Kaempferol as an anticancer agent will be a breakthrough in natural anticancer research.
Conclusion
Kaempferol, the most promising anticancer agent against breast cancer in E. hirta, exhibits drug-like properties, is nontoxic to humans, can efficiently penetrate the plasma membrane, and demonstrates high anticancer activity. Moreover, Kaempferol has shown stable binding to AKT1 and ERα, enabling the prediction of its inhibitory effect on the activity of these two proteins. Notably, AKT1 and ERα are important proteins of the PI3K/Akt and ER signaling pathways, which play critical roles in breast cancer. Thus, Kaempferol displays anti-breast cancer activity by effectively inhibiting the AKT1 and ERα proteins.
Acknowledgments
This research was supported by Bioinformatics course for giving facilities during this research.
Disclosure statement
No potential conflict of interest was reported by the author(s).
Data availability statement
The data supporting the findings of this study, including figures, tables, and raw data, are in the figshare repository and can be accessed using the link: https://doi.org/10.6084/m9.figshare.23742036.
Additional information
Funding
References
- Chhikara BS, Parang K. Global cancer statistics 2022: the trends projection analysis. Chem Biol Lett. 2023;10(1):451.
- Siegel RL, Miller KD, Wagle NS, et al. Cancer statistics, 2023. Ca A Cancer J Clinicians. 2023;73(1):17–48. doi: 10.3322/caac.21763
- DeSantis CE, Ma J, Gaudet MM, et al. Breast cancer statistics, 2019. CA A Cancer J Clin. 2019;69(6):438–451. doi: 10.3322/caac.21583
- Ahmed K, Asaduzzaman S, Bashar MI, et al. Association Assessment among risk factors and breast cancer in a low-income country: Bangladesh. Asian Pac J Cancer Prev. 2015;16(17):7507–7512. doi: 10.7314/APJCP.2015.16.17.7507
- Paul MR, Pan T, Pant DK, et al. Genomic landscape of metastatic breast cancer identifies preferentially dysregulated pathways and targets. J Clin Investig. 2020. doi: 10.1172/JCI129941
- Clusan L, Ferrière F, Flouriot G, et al. A basic review on estrogen receptor signaling pathways in breast cancer. Int J Mol Sci. 2023;24(7):6834. doi: 10.3390/ijms24076834
- Paplomata E, O’Regan R. The PI3K/AKT/mTOR pathway in breast cancer: targets, trials and biomarkers. Ther Adv Med Oncol. 2014;6(4):154–166. doi: 10.1177/1758834014530023
- Golestan S, Soltani BM, Jafarzadeh M, et al. LINC02381 suppresses cell proliferation and promotes apoptosis via attenuating IGF1R/PI3K/AKT signaling pathway in breast cancer. Funct Integr Genomics. 2023;23(1):40. doi: 10.1007/s10142-023-00965-w
- Mukohara T. PI3K mutations in breast cancer: prognostic and therapeutic implications. Breast Cancer (Dove Med Press). 2015;111. doi: 10.2147/BCTT.S60696
- Wu W, Chen Y, Huang L, et al. Effects of AKT1 E17K mutation hotspots on the biological behavior of breast cancer cells. J Clin Exp Pathol. 2020;13(3):332–346.
- Roy SS, Vadlamudi RK. Role of estrogen receptor signaling in breast cancer metastasis. Int J Breast Cancer. 2012;2012:1–8. doi: 10.1155/2012/654698
- Holst F, Stahl PR, Ruiz C, et al. Estrogen receptor alpha (ESR1) gene amplification is frequent in breast cancer. Nat Genet. 2007;39(5):655–660. doi: 10.1038/ng2006
- Kumar S, Malhotra R, Kumar D. Euphorbia hirta: its chemistry, traditional and medicinal uses, and pharmacological activities. Phcog Rev. 2010;4(7):58. doi: 10.4103/0973-7847.65327
- Sharma N, Samarakoon K, Gyawali R, et al. Evaluation of the antioxidant, anti-inflammatory, and anticancer activities of Euphorbia hirta ethanolic extract. Molecules. 2014;19(9):14567–14581. doi: 10.3390/molecules190914567
- Tran N, Nguyen M, Le KP, et al. Screening of antibacterial activity, antioxidant activity, and anticancer activity of Euphorbia hirta Linn Extracts. Appl Sci. 2020;10(23):8408. doi: 10.3390/app10238408
- Kalaivani S, Jayanthi S, Revathi K, et al. Phytochemical profile of Euphorbia hirta plant extract and its in vitro anticancer activity against the liver cancer HepG2 cells. Vegetos. 2023. doi: 10.1007/s42535-023-00621-5
- Abu Bakar FI, Abu Bakar MF, Abdullah N, et al. Optimization of extraction conditions of phytochemical compounds and anti-gout activity of Euphorbia hirta L. (Ara Tanah) using response surface methodology and liquid chromatography-mass spectrometry (LC-MS) analysis. Evid Based Complement Alternat Med. 2020;2020:1–13. doi: 10.1155/2020/4501261
- Mekam PN, Martini S, Nguefack J, et al. Phenolic compounds profile of water and ethanol extracts of Euphorbia hirta L. leaves showing antioxidant and antifungal properties. S Afr J Bot. 2019;127:319–332. doi: 10.1016/j.sajb.2019.11.001
- Daina A, Michielin O, Zoete V. SwissADME: a free web tool to evaluate pharmacokinetics, drug-likeness and medicinal chemistry friendliness of small molecules. Sci Rep. 2017;7(1):42717. doi: 10.1038/srep42717
- Banerjee P, Eckert AO, Schrey AK, et al. ProTox-II: a webserver for the prediction of toxicity of chemicals. Nucleic Acids Res. 2018;46(W1):W257–W263. doi: 10.1093/nar/gky318
- Lomize AL, Hage JM, Schnitzer K, et al. PerMM: a web tool and database for analysis of passive membrane permeability and translocation pathways of bioactive molecules. J Chem Inf Model. 2019;59(7):3094–3099. doi: 10.1021/acs.jcim.9b00225
- Filimonov DA, Lagunin AA, Gloriozova TA, et al. Prediction of the biological activity spectra of organic compounds using the pass Online web resource. Chem Heterocycl Comp. 2014;50(3):444–457. doi: 10.1007/s10593-014-1496-1
- Daina A, Michielin O, Zoete V. SwissTargetPrediction: updated data and new features for efficient prediction of protein targets of small molecules. Nucleic Acids Res. 2019;47(W1):W357–W364. doi: 10.1093/nar/gkz382
- Davis AP, Grondin CJ, Johnson RJ, et al. Comparative toxicogenomics database (CTD): update 2021. Nucleic Acids Res. 2021;49(D1):D1138–D1143. doi: 10.1093/nar/gkaa891
- Kuhn M, Szklarczyk D, Franceschini A, et al. STITCH 3: zooming in on protein-chemical interactions. Nucleic Acids Res. 2012;40(D1):D876–D880. doi: 10.1093/nar/gkr1011
- Sherman BT, Hao M, Qiu J, et al. DAVID: a web server for functional enrichment analysis and functional annotation of gene lists (2021 update). Nucleic Acids Res. 2022;50(W1):W216–W221. doi: 10.1093/nar/gkac194
- O’Boyle NM, Banck M, James CA, et al. Open babel: an open chemical toolbox. J Cheminform. 2011;3(1):33. doi: 10.1186/1758-2946-3-33
- Trott O, Olson AJ. AutoDock Vina: improving the speed and accuracy of docking with a new scoring function, efficient optimization, and multithreading. J Comput Chem. 2009;31(2):NA–NA. doi: 10.1002/jcc.21334
- Weisner J, Landel I, Reintjes C, et al. Preclinical efficacy of Covalent-Allosteric AKT inhibitor Borussertib in combination with trametinib in KRAS -mutant pancreatic and colorectal cancer. Cancer Res. 2019;79(9):2367–2378. doi: 10.1158/0008-5472.CAN-18-2861
- Shiau AK, Barstad D, Loria PM, et al. The structural Basis of estrogen receptor/Coactivator Recognition and the antagonism of this interaction by tamoxifen. Cell. 1998;95(7):927–937. doi: 10.1016/S0092-8674(00)81717-1
- Krieger E, Vriend G. YASARA view—molecular graphics for all devices—from smartphones to workstations. Bioinformatics. 2014;30(20):2981–2982. doi: 10.1093/bioinformatics/btu426
- Genheden S, Ryde U. The MM/PBSA and MM/GBSA methods to estimate ligand-binding affinities. Espert Opin Drug Discov. 2015;10(5):449–461. doi: 10.1517/17460441.2015.1032936
- Chen AY, Chen YC. A review of the dietary flavonoid, kaempferol on human health and cancer chemoprevention. Food Chem. 2013;138(4):2099–2107. doi: 10.1016/j.foodchem.2012.11.139
- Ulrih NP, Maričić M, Ota A, et al. Kaempferol and quercetin interactions with model lipid membranes. Food Res Int. 2015;71:146–154. doi: 10.1016/j.foodres.2015.02.029
- Milenković D, Dimitrić Marković JM, Dimić D, et al. Structural characterization of kaempferol: a spectroscopic and computational study. Maced J Chem Chem Eng. 2019;38(1):49. doi: 10.20450/mjcce.2019.1333
- Hung TM, Dang NH, Kim JC, et al. Phenolic glycosides from Alangium salviifolium leaves with inhibitory activity on LPS-induced NO, PGE2, and TNF-α production. Bioorg Med Chem Lett. 2009;19(15):4389–4393. doi: 10.1016/j.bmcl.2009.05.070
- Wang J, Fang X, Ge L, et al. Antitumor, antioxidant and anti-inflammatory activities of kaempferol and its corresponding glycosides and the enzymatic preparation of kaempferol. PLoS One. 2018;13(5):e0197563. doi: 10.1371/journal.pone.0197563
- Imran M, Salehi B, Sharifi-Rad J, et al. Kaempferol: a key emphasis to its anticancer potential. Molecules. 2019;24(12):2277. doi: 10.3390/molecules24122277
- Bickerton GR, Paolini GV, Besnard J, et al. Quantifying the chemical beauty of drugs. Nat Chem. 2012;4(2):90–98. doi: 10.1038/nchem.1243
- Lomize AL, Pogozheva ID. Physics-based method for modeling passive membrane permeability and translocation pathways of bioactive molecules. J Chem Inf Model. 2019;59(7):3198–3213. doi: 10.1021/acs.jcim.9b00224
- Pantsar T, Poso A. Binding affinity via docking: fact and fiction. Molecules. 2018;23(8):1899. doi: 10.3390/molecules23081899
- Majewski M, Ruiz-Carmona S, Barril X. An investigation of structural stability in protein-ligand complexes reveals the balance between order and disorder. Commun Chem. 2019;2(1):110. doi: 10.1038/s42004-019-0205-5
- Martínez L, Kleinjung J. Automatic identification of Mobile and rigid substructures in Molecular dynamics Simulations and fractional structural fluctuation analysis. PLoS One. 2015;10(3):e0119264. doi: 10.1371/journal.pone.0119264
- Pace CN, Fu H, Lee Fryar K, et al. Contribution of hydrogen bonds to protein stability: hydrogen bonds and protein stability. Protein Sci. 2014;23(5):652–661. doi: 10.1002/pro.2449
- Kumar N, Sood D, Tomar R, et al. Antimicrobial peptide designing and optimization employing large-scale flexibility analysis of protein-peptide fragments. ACS Omega. 2019;4(25):21370–21380. doi: 10.1021/acsomega.9b03035
- Wargasetia TL, Ratnawati H, Widodo N, et al. Bioinformatics study of sea cucumber peptides as antibreast cancer through inhibiting the activity of overexpressed protein (EGFR, PI3K, AKT1, and CDK4). Cancer Inform. 2021;20:1–11. doi: 10.1177/11769351211031864
- Bhardwaj VK, Singh R, Sharma J, et al. Identification of bioactive molecules from tea plant as SARS-CoV-2 main protease inhibitors. J Biomol Struct Dyn. 2021;39(10):3449–3458. doi: 10.1080/07391102.2020.1766572
- Ajmani S, Agrawal A, Kulkarni SA. A comprehensive structure–activity analysis of protein kinase B-alpha (Akt1) inhibitors. J Mol Graph Model. 2010;28(7):683–694. doi: 10.1016/j.jmgm.2010.01.007
- Lin K, Lin J, Wu W-I, et al. An ATP-Site on-off switch that restricts phosphatase accessibility of Akt. Sci Signal. 2012;5(223). doi: 10.1126/scisignal.2002618
- Ciruelos Gil EM. Targeting the PI3K/AKT/mTOR pathway in estrogen receptor-positive breast cancer. Cancer Treat Rev. 2014;40(7):862–871. doi: 10.1016/j.ctrv.2014.03.004
- Saura C, Roda D, Roselló S, et al. A first-in-human phase I study of the ATP-Competitive AKT inhibitor ipatasertib demonstrates robust and safe targeting of AKT in patients with solid tumors. Cancer Discov. 2017;7(1):102–113. doi: 10.1158/2159-8290.CD-16-0512
- Kumar R, Zakharov MN, Khan SH, et al. The dynamic structure of the estrogen receptor. J Amino Acids. 2011;2011:1–7. doi: 10.4061/2011/812540
- Arnal J-F, Lenfant F, Metivier R, et al. Membrane and nuclear estrogen receptor alpha actions: from tissue specificity to medical implications. Physiol Rev. 2017;97(3):1045–1087. doi: 10.1152/physrev.00024.2016
- Yaşar P, Ayaz G, User SD, et al. Molecular mechanism of estrogen-estrogen receptor signaling. Reprod Med Biol. 2017;16(1):4–20. doi: 10.1002/rmb2.12006
- Feng Y, Spezia M, Huang S, et al. Breast cancer development and progression: risk factors, cancer stem cells, signaling pathways, genomics, and molecular pathogenesis. Genes Dis. 2018;5(2):77–106. doi: 10.1016/j.gendis.2018.05.001
- Miyoshi Y, Murase K, Saito M, et al. Mechanisms of estrogen receptor-α upregulation in breast cancers. Med Mol Morphol. 2010;43(4):193–196. doi: 10.1007/s00795-010-0514-3
- Xin L, Min J, Hu H, et al. Structure-guided identification of novel dual-targeting estrogen receptor α degraders with aromatase inhibitory activity for the treatment of endocrine-resistant breast cancer. Eur J Med Chem. 2023;253:115328. doi: 10.1016/j.ejmech.2023.115328