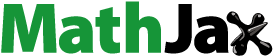
Abstract
The paper presents the evaluation of the performance of various aeroderivative gas turbine models (AGTM) derived from a high bypass turbofan engine (HBTE). The objective of the study is to determine the performance and suitability of the developed AGTM for power generation. Furthermore, the models were specified as Model I (two shafts with FPT), Model II (two shafts with direct-drive LP turbine), and Model III (one shaft with FPT). The useful performance data for the AGTM was generated using the turbomatch simulation code. Additionally, the preliminary design point (DP) performance results estimated the power output and thermal efficiency of Models I, II, and III which ranged between and
respectively. However, after the preliminary analysis, Model III failed the test based on performance and economic constraint while Models I and II were subjected to further performance study. The analysis indicates that Model II runs into surge at about 72% DP power and would require the installation of bleed valves or variable stator vanes for stability. Hence, additional production costs. Nonetheless, Model I was operationally stable at part-load conditions (< 110% DP Power) with no compressor surging. Based on the observed operational performance of the Models, Model 1 was best preferred and thus suggested for possible development.
Public Interest Statement
Aero-engines apart from its application in propelling civil and military aircrafts, it could equally be adapted into aeroderivative models for power generation. The high power to weight ratio and high thermal efficiency has placed them at advantage over the heavy-duty gas turbine generators. Today, there exist two large markets for industrial gas turbines. One for heavy-duty gas turbines designed for land-based applications found in large combined cycle power plants. The other is the light-weighted and compact aeroderivative gas turbines, which operates in simple cycle gas turbine systems. Aeroderivative gas turbine models of the CFM56 high bypass turbofan engine family which is not currently in the market, has been developed for possible industrial application (power generation). Evaluation of the optimum performance of the adapted models at part-load condition has been carried out. The best model has also been suggested to gas turbine manufacturers for possible development.
1. Introduction
In recent times, the use of aero-engines for power generation is becoming common owing to high efficiency and low emission rate (Abam, Ugot, & Igbong, Citation2011, Citation2012). The aero-engines apart from its application in propelling civil and military aircraft, it could equally be adapted into aeroderivative models for power generation. The high power to weight ratio and high thermal efficiency has placed them at advantage over the heavy-duty gas turbine (GT) generators (Al-Hamdan & Ebaid, Citation2005; Bhargava et al., Citation2009). Moreover, the application of the first produced aeroderivative GT was for gas pipeline compression (Bhargava et al., Citation2009; Cohen, Rogers, & Saravanamuttoo, Citation2009), which was later extended to peak-load generation (Al-Hamdan & Ebaid, Citation2005). Today, there exist two large markets for industrial gas turbines. One for heavy-duty gas turbines designed for land-based applications found in large combined cycle power plants. The other is the light-weighted and compact aeroderivative gas turbines, which operates in simple cycle GT systems.
The available aeroderivative engines include models of GE LM6000 for power generation derived from CF6–80C2 turbofan engine, models of Rolls Royce WR-21 for marine application derived from RR RB211 turbofan engine, and GE LM2500 model derived from CF6 high bypass turbofan engine family (CFM International, Citation2000). The CFM 56 HBTE engine family is designed and manufactured by CFM International and entered service in 1993. The engine was designed for long-range flight applications and powers the Airbus A340-211, A340-311 and Boeing 737 aircraft (CFM International, Citation2000; Gunston, Citation2005). Furthermore, the CFM 56 engine has a moderate core size compared to other turbofan engines such as the GE90 (Gilani, Baheta, & Rangkuti, Citation2009; Gunston, Citation2005). The industrial derivative of the CFM 56 engine family does not exist currently in the open market. The objective of the paper is to develop the aeroderivative gas turbine models of the CFM56 engine for possible industrial application (power generation). Additionally, the paper will also evaluate the optimum performance of the adapted models at part-load condition. The performance of these models at this condition is then compared to determine the model with optimum performance.
2. Methodology
The aeroderivative model was created from a high bypass turbofan engine similar to CFM56–5C2 turbofan engine for both takeoff and cruise conditions. The data used for the initial development of the AGTM were validated with existing data in open literature (Bringhenti & Barbosa, Citation2004; Gilani et al., Citation2009; Palmer, Citation1999) before adopting. The design point and off-design (part-load) performance of the AGTM were simulated using turbomatch with the inputs data depicted in Table . However, the performance results were compared vis-à-vis the power output, specific fuel consumption, and thermal efficiency. This was to allow the selection of the best engine model for further investigation. The proposed engine models are described as follows: Model I—two-shaft engine with free power turbine (FPT), Model II—two-shaft engine and a direct-drive low pressure turbine (LPT), and Model III—single shaft engine with free power turbine (FPT).
Table 1. Turbomatch inputs for design point and cruise conditions (Palmer, Citation1999; Roux, Citation2011; Walsh & Fletcher, Citation2004)
2.1. Model description of the high bypass turbofan engine
The preliminary turbomatch set up model used for the turbofan engine is presented in Figure . This is similar to the engine configuration of the CFM56-5C2 turbofan engine. Each brick represents an engine component which forms the structure of the turbomatch codes (Roux, Citation2011; Walsh & Fletcher, Citation2004). The dotted lines represent ducts by which the air from the core is bled to other components. The system comprises the intake nozzle, Fan, LPC (Booster), HPC, HPT, LPT, the duct, and exit nozzle.
In converting the HBTE into an industrial GT for power generation, the bypass turbofan components were removed and the high pressure (HP) core retained. The objective here is to keep as high commonality with the original turbofan engine so that the HP core of the three industrial configurations could operate under identical conditions, and have same HP core design and geometry. This will minimize the number of parts that need to be redesigned and hence reduce the cost of conversion. Also, less modification from the parent engine means less conversion time which may accelerate the supply chain and, therefore, benefits both the manufacturer and customers.
2.2. Thermodynamic approaches
The fundamental thermodynamic equations governing the brick code arrangement for the turbofan engine in Figure are presented. The assumptions in the system control volumes are based on the steady state energy consideration (Equation 1) which includes the following: compression and expansion processes are considered isentropic. The working fluid is considered to have ideal characteristics and with constant composition throughout the control volumes. Constant-pressure specific heat data for the constituent gasses occur as a polynomial function of temperature (Effiom, Abam, & Ohunakin, Citation2015; Lee, Kang, & Kim, Citation2011) as presented in Equation (2). Other specific assumptions for the component by component modeling are summarized in Table .(1)
(1)
(2)
(2)
Table 2. Assumed values for the turbofan turbomatch simulation related to GT models (Lefebvre, Citation1998; Nkoi et al., Citation2013a; Palmer, Citation1999; Sethi, Citation2014)
where, ∊1, ∊2, ∊3, and ∊4 are constants.
2.3. Thermodynamic balances for the different control volumes
2.3.1. Intake nozzle
Equation (3) defines the isentropic ratio between the total and static pressures at the intake nozzle (Figure ) as it relates to the Mach number (Mn) (Gilani et al., Citation2009). At take-off and cruise conditions, Mn is assumed as 0 and 0.8 respectively (Cohen et al., Citation2009). Hence the inlet temperature, velocity and pressure conditions are presented in Equations (4) and (5).(3)
(3)
(4)
(4)
(5)
(5)
(6)
(6)
where(7)
(7)
2.3.2. The Fan component
The fan inlet conditions (Figure ) is given by:(8)
(8)
Applying the isentropic relationship the exit pressure
and temperature
are well-defined respectively (Effiom et al., Citation2015).
(9)
(9)
(10)
(10)
Further applying Equation (10) to the engine model in (Figure ) the fan work FW is expressed by:(11)
(11)
where is the mass flow rate of air in the fan.
2.3.3. Low pressure compressor
Low pressure compressor (LPC) inlet conditions: The temperature and pressure at LPC inlet (Figure ) are equal to the temperature and pressure at the fan outlet.(12)
(12)
LPC outlet conditions are established based on the isentropic correlation (Effiom et al., Citation2015):(13)
(13)
(14)
(14)
From Equation (13) the work output of the LPC is given by:(15)
(15)
2.3.4. High pressure compressor
The inlet conditions of the High pressure compressor (HPC) (Figure ) with assumptions in Table (Roux, Citation2011; Walsh & Fletcher, Citation2004):(16)
(16)
(17)
(17)
The outlet conditions of the HPC are defined by:(18)
(18)
(19)
(19)
Equation (20) expresses the work output of the HPC while Equations (21) and (22) present the non-dimensional flow capacities at inlet and outlet of the HPC respectively.(20)
(20)
(21)
(21)
(22)
(22)
2.3.5. Combustor
Inlet conditions of the combustion chamber were determined following the energy balance within the control volume (9–10) Figure . From Table 99.9% was assumed for combustion efficiency and 13% bled air was used for cooling the HPT blades (Nkoi, Pilidis, & Nikolaidis, Citation2013a, Citation2013b).(23)
(23)
(24)
(24)
The combustor outlet temperature (COT) is expressed in Equation (25) with 95% of compressed air used for combustion (Lefebvre, Citation1998; Sethi, Citation2014).(25)
(25)
(26)
(26)
Heat input to the combustor is expressed as:(27)
(27)
2.3.6. High pressure turbine
In the High pressure turbine (HPT) 13% bled air is used for HPT blades cooling as expressed in Equations (28) and (29) (Nkoi et al., Citation2013a)(28)
(28)
(29)
(29)
The inlet pressure to the HPT and the non-dimensional inlet flow capacity are obtained in Equations (30) and (31) respectively.(30)
(30)
(31)
(31)
Since the HPT drives the HPC and using an HP spool, it implies that the work done by the HPC and HPT is equal. Consequently, the following exit conditions are established.(32)
(32)
(33)
(33)
2.3.7. Low pressure turbine
The Low pressure turbine (LPT) drives the LPC and the fan with the help of a low power shaft (LPS). The LPT outlet conditions T13 and P13 are thus described (Nkoi et al., Citation2013b; Safran Snecma, Citation2011).(34)
(34)
(35)
(35)
2.3.8. Nozzle duct
Equations (36) to (38) presents the energy balance in the nozzle duct, taking into account temperature, pressure and mass flow rate of the nozzle duct.(36)
(36)
(37)
(37)
(38)
(38)
2.3.9. Mixed exhaust nozzle
The isentropic ratio between the total and static pressures at the exhaust nozzle are related to its exit Mach number as expressed in Equations (39) and (40) (Cohen et al., Citation2009; Nkoi et al., Citation2013b).(39)
(39)
(40)
(40)
From Equations (41) and (42) the static pressure of the exit nozzle can be determined by:(41)
(41)
From continuity equation, the exit exhaust mass flow of the nozzle is defined by:(42)
(42)
Where VN is the nozzle exit velocity expressed below:(43)
(43)
The area of the mixed exhaust nozzle can be expressed by the following relation (Cohen et al., Citation2009):(44)
(44)
where tN is the static temperature of the exit nozzle.(45)
(45)
Rearranging Equations (43) to (44) the HBTE thrust produced from the nozzle, and the specific fuel consumption (SFC) of the HBTE can be expressed as in Equations (46) and (47) respectively.(46)
(46)
(47)
(47)
3. High bypass turbofan engine aeroderivative models development
In converting the High bypass turbofan engine (HBTE) into industrial AGTM for power generation, the bypass turbofan components were removed and the HP core retained. The goal is to keep as high commonality with the original turbofan engine so that the HP core of the three industrial configurations could operate under identical conditions, and have same HP core design and geometry. The latter will minimize the number of parts that need to be redesigned and hence minimize the cost of conversion. Also, less modification from the parent engine means less conversion time which may accelerate the supply chain and, therefore, benefits both the manufacturer and customers.
3.1. Model I—Two-spool with a free power turbine model
In Model 1 both the low pressure shaft (LPS) and high pressure shaft (HPS) are retained from the original HBTE with the fan removed. The HPT only drives the HPC while the HPT core is kept identical with that of the HBTE allowing the LPS to rotate faster. The LPT only drives the LPC and modified to reduce the number of stages. A Free power turbine was added downstream to expand the flow to atmospheric pressure and equally extract the remaining energy in the flow to drive the electrical generator. The model schematic and the turbomatch brick arrangement is depicted in Figure (a) and (b).
3.2. Model II—Two spool with integrated power turbine on LPS (direct-drive)
In this model, the two shafts configuration was retained from the original HBTE while the free power turbine was removed. The LPS was directly connected to the electrical generator. The rotational speed of the LPS is fixed due to the requirement of the generator to maintain a frequency of 50 or 60 Hz. Hence, the flow is expanded to the atmospheric pressure by the LPT. The model schematic and the turbomatch brick arrangement is depicted in Figure (c) and (d).
3.3. Model III—Single-spool with FPT
In Model III, the LPS is completely removed. The core gas generator was retained, and a free power turbine (FPT) was mounted downstream to expand the flow to atmospheric pressure, thus extracting the remaining energy in the flow to drive the electrical generator. The model schematic and the turbomatch brick arrangement is depicted in Figure (e) and (f).
3.4. Part-load performance simulation of the GT model with Variable stator vanes or Bleed valves
Part-Load simulation was carried out to investigate if Model II can operate smoothly down to about 50% of its full load with either Bleed valves (BVs) or Variable stator vanes (VSV). For part-load simulation, the COT was used as an engine handle and was varied in order to change the loading condition of the engine, the shaft rotational speed (PCN) of the LP compressor was fixed, while the HP compressor PCN was allowed to vary.
The bleed valves (BVs) schedule was determined by using Turbomatch simulations, in which the surge parameter, Z, is given as an output for each input operating condition (Palmer, Citation1999). The combustor outlet temperature (COT) was reduced to simulate the off-design part-load conditions. Bleed valves were set in Turbomatch by the use of a PREMAS (air splitter) brick between the LP and the HP compressors. Conversely, variable stator vanes (VSVs) were set directly into the Turbomatch program by changing the value of the ‘VSV angle’ brick data item, which is the ‘variable geometry’ (VA) (Cohen et al., Citation2009; Palmer, Citation1999). The VSV angle was varied in steps of 2 degrees until the final chosen angle of 10⁰ was reached to avoid surge at approximately 50% of full-load power.
4. Result and discussion
4.1. HBTE simulation results and validation
Table depicts the turbomatch performance simulation results of the HBTE. The results obtained were compared with that in (CFM International, Citation2000; Palmer, Citation1999; Roux, Citation2011; Safran Snecma, Citation2011). Additionally, DP simulation results from the created models agreed with values in literature. The errors were specified at 0.5, 3 and 2% for the net thrust, fuel flow, and SFC in that order. Also, the obtained values for the cruise condition compared well with only increase error of 5% coming from the specific fuel consumption (SFC) at cruise condition. Nevertheless, the HBTE model created was realistic and consequently, adopted for the advance of possible aeroderivative models.
Table 3. Comparison of simulated results for the HPTE with published data (CFM International, Citation2000; Palmer, Citation1999; Roux, Citation2011; Safran Snecma, Citation2011)
4.2. Results comparison of the aeroderivative models
Table presents the results of the three aeroderivative models. The power output (POT), thermal efficiency (TE) and the specific power (SP) ranged between , 29.8 ≤ ηth ≤ 38.4 % and
for Model I, II and III respectively. The TE for Model I is approximated at 38.4% while Model II is 38.1% with a marginal difference 0.3%. Model III with no HPT cooling has a TE of 29.8% with the lowest POT of 3.46 MW while 10.67 and 10.60 MW was calculated for Models I and II. The HP Turbine entry temperature (TET) of Model III was so high above the original turbofan engine (1,542.7 K). Therefore, HPT cooling was required to reduce the HPT blade temperature and the performance effect obtained. Model III low performance result of POT and ηth makes it technically and economically unviable for further development and consideration.
Table 4. Comparison of simulated results at DP for the aeroderivative Models I, II & III
4.3. Off-design result of part load performance for aeroderivative Models I and II
The part-load conditions for the two models are depicted in Figures –. Moreover, Figure describes the POT at part-load (off design) for varying COT: (i) Model I with FPT (ii) Model II with Power turbine on LPS (VSV – 10 degree fixed), (iii) Model II with power turbine on LPS (Normal) and (iv) Model II with power turbine on LPS (BV = 10% fixed). The performance of the GTs models for these scenarios was close at the initial start for increasing COT (Figure ). However, at DP, the difference in the POT between Model I with FPT and Model II with Power turbine on LPS (VSV – 10 degree fixed) was 17% whereas Model II with power turbine on LPS (BV = 10% fixed) was 10.09%. Similarly, Model I at DP has the same POT with Model II with power turbine on LPS. Consequently, beyond the DP at COT of 1,450 K the difference between Model I with a free power turbine and Model II with power turbine on LPS was 7.14%. The specific fuel consumption (SFC) which ascertains the quantity of fuel consumed by the engine per second to produce 1 MW of power was also used to investigate the performance of Model I and II at part load conditions as presented in Figure . The decrease in SFC is as a result of increase in COT, since less fuel is burnt to achieve optimal power. However, Model II (Normal) runs into surge at increasing SFC above 6.9% and decreasing COT below 1,280 K as a result of decreasing power (part load). The use of 10% fixed BV and 10 degree fixed VSV helped to overcome this effect.
Figure 4a. Part-load performance (off design): thermal efficiency vs. combustion outlet temperature.
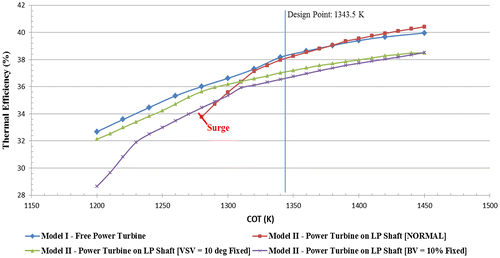
Figure 4b. Part-load performance (off design): specific fuel consumption vs. combustion outlet temperature.
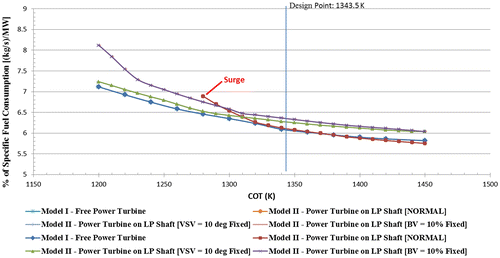
Additionally, a careful study of Figures –. shows that Model I have no surging problems and has a better performance at part load conditions while Model II (Normal) performs well to a point where surging problems occur. An extension from the surge point of Model II (Normal) is detrimental to the compressor. This is because the speed of the LP shaft of Model II is constrained by the speed of the generator. The surging problem on the LP compressor shaft of Model II was then corrected with the use of BVs and VSV fixed at 10% and 10 degrees respectively (see Figures –.). However, Model II without BVs or VSVs shows a better performance than Model I at conditions higher than the designed load (<110% DP Power) Figure . Nonetheless, the engine runs into surge or upwelling at load conditions below 72% of DP load. At this load condition, the engine may not run effectively except BVs or VSVs are used. For clarity the surge point for Models I and II is depicted on the compressor map in Figure for all the scenarios with compressor rotational speed (CN) range of 70–110%.
4.4. Result of the LP Compressor Bleed valve and variable stator vane schedules for Model II
From Figure , the point where bleed valves need to start opening coincides exactly with the design point (100% loaded). Additionally, at powers >100%, the surge margin becomes >15% and no bleed is needed from the LP compressor. At 100% power, the bleed valve begins to open and at powers ≤100% more bleed is needed in order to keep the surge margin steady at 15% while maintaining the operating point from surging. Figure shows the operating line of the compressor map with scheduled VSV angles of 0° and 10° with constant surge margin of 15%. Before the VSVs begin operation at powers >100%, the operating line follows the constant speed line trend. However, when the VSVs begin operation and the angle is gradually increased, the entire compressor map shifts to the left likewise the operating point at constant speed line.
5. Conclusion
Aeroderivative gas turbine models for industrial power generation have been developed from a HBTE. Three configurations were obtained from the HBTE. These include (i) Model I—Two-spool with FPT (ii) Model II—Two spool with integrated power turbine on LPS (iii) Model III—Single-spool with FPT. Initial DP results show that the power output and thermal efficiency of the Models I, II and II were approximated at 10.67, 38.4%, 10.60 MW, 38.1% and 3.46 MW, 29.8% respectively. However, Models I and II were subjected to further performance test based on the good initial result at DP. The performance results indicate that Model II runs into surge at about 70% DP load and hence requires BVs or VSVs which may increase production costs while Model I performed well at designed load (<110% DP Power). Also, its commonality with the parent HBTE reduced modification and thus manufacturing costs. For this reasons Model, I—Two-spool with FPT was considered worthwhile for development. Simulated result of Model II without BVs and VSVs showed that operating Model II at a fixed RPM, leads to compressor surging problems of about 70–75% when the generator is offloaded. Therefore, BVs and VSVs have to be installed on the compressors for stability. Finally, with VSVs operating in a scheduled manner, the performance of the Model II is optimized. Therefore, installing BVs and VSVs on the compressor component of a two-shaft direct drive GT system (Model II) will avoid compressor surging problems, regulate the air flow, and maintain operational component stability at low speeds and low power demands.
Funding
The authors received no direct funding for this research.
Nomenclature | ||
AGTM | = | aeroderivative gas turbine models |
BPR | = | by pass ratio |
BV | = | bleed valve |
CN | = | compressor rotational speed |
COP | = | combustor outlet pressure (kPa) |
COT | = | combustor outlet temperature (K) |
Cp | = | specific heat capacity (kJ kg−1 K−1) |
DP | = | design point |
FAR | = | fuel to air ratio |
FCV | = | fuel calorific value (kJ kg−1) |
FF | = | fuel flow (kg s−1) |
FPT | = | free power turbine |
FPR | = | fan pressure ratio |
GT | = | gas turbine |
HBTE | = | high bypass turbofan engine |
HPC | = | high pressure compressor |
HPCW | = | high pressure compressor work |
HPS | = | high pressure shaft |
HPT | = | high pressure turbine |
LPC | = | low pressure compressor |
LPCW | = | low pressure compressor work |
LPS | = | low pressure shaft |
LPT | = | low pressure turbine |
= | mass flow rate (kg s−1) | |
NDFC | = | non dimensional flow capacity |
P | = | pressure (kPa) |
POT | = | power output (MW) |
PR | = | pressure ratio |
R | = | gas constant of air (J kg−1 K−1) |
RPM | = | revolution per minute |
SFC | = | specific fuel consumption [(kg/s)/MW] |
SP | = | specific power (MW kg−1 s−1) |
SR | = | simulated result |
t | = | static temperature (K) |
T | = | total temperature (K) |
TH | = | Thrust (N) |
v | = | velocity (m s−1) |
VSV | = | variable stator vanes |
Greek symbols | ||
γ | = | specific heat ratio |
η | = | thermal efficiency (%) |
= | isentropic efficiency (%) | |
ρ | = | density (kg m−3) |
ϕ | = | combustion efficiency |
Subscripts | ||
amb | = | ambient |
f | = | fan |
g | = | gas |
in | = | inlet |
out | = | outlet |
recov | = | recovery |
Additional information
Notes on contributors
Samuel O. Effiom
Samuel O. Effiom obtained his MSc degree in Thermal Power (Gas turbine/aerospace propulsion) from Cranfield University, England, UK. He is currently a PhD Research student in Energy and Power engineering at Michael Okpara University of Agriculture, Nigeria. His research interests are: thermal power propulsion systems, Renewable energy systems, CFD, exergy and environment.
Fidelis I. Abam
Fidelis I. Abam is an academic researcher at the Michael Okpara University of Agriculture, Nigeria. He holds a PhD degree in Energy and power technology from the University of Nigeria and a Master’s degree in thermo-fluid engineering from University of Lagos, Nigeria. His research interests are: exergy and environment, renewable energy system and power plant engineering.
Bethrand N. Nwankwojike
Bethrand N. Nwankwojike is an associate professor in the Department of Mechanical Engineering, Michael Okpara University of Agriculture, Umudike, Nigeria. He holds a PhD and M.Eng degree in Mechanical Engineering (Industrial and production engineering/management) from University of Nigeria. His research interests are: Engineering systems design, development, analysis/modelling and optimization.
References
- Abam, F. I., Ugot, I. U., & Igbong, D. I. (2011). Thermodynamic assessment of grid-based gas turbine power plants in Nigeria. Journal of Emerging Trends in Engineering & Applied Science, 2, 1026–1033.
- Abam, F. I., Ugot, I. U., & Igbong, D. I. (2012). Performance analysis and component irreversibilities of a (25 MW) gas turbine plant modeled with a spray cooler. American Journal of Engineering & Applied Science, 5, 35–41.
- Al-Hamdan, Q. Z., & Ebaid, M. S. Y. (2005). Modeling and simulation of a gas turbine engine for power generation. Journal of Engineering for Gas Turbines & Power, 128, 302–311. doi:10.1115/1.2061287
- Bhargava, R. K., Bianchi, M., Campanari, S., De Pascale, A., Negri di Montenegro, G., & Peretto, A. (2009). A parametric thermo-dynamic evaluation of high performance gas turbine based power cycles. Journal of Engineering for Gas Turbines & Power, 132, 022001.1–022001.14. doi:10.1115/1.3155782
- Bringhenti, C., & Barbosa, J. R. (2004). Methodology for gas turbine performance improvement using variable-geometry compressors and turbines. Proceedings of the Institution of Mechanical Engineers, Part A: Journal of Power and Energy, 218, 541–549.10.1243/0957650042456980
- CFM International. (2000). CFM56-5 training manual (2nd ed.). Cincinnati, OH: Author.
- Cohen, H., Rogers, G. F. C., & Saravanamuttoo, H. I. H. (2009). Gas turbine theory (6th ed.). (pp. 42–60). Canada: Pearson Education.
- Effiom, S. O., Abam, F. I., & Ohunakin, O. S. (2015). Performance modeling of industrial gas turbines with inlet air filtration system. Case Studies in Thermal Engineering, 5, 160–167. doi:10.1016/j.csite.2015.03.008
- Gilani, S. I., Baheta, U. H., & Rangkuti, A. T. (2009). Study on the effect of variable vanes on performance of axial compressor for single shaft gas turbine cogeneration plant. Energy and Environment, 3, 40–44. doi:10.1109/ICEENVIRON.2009.5398675
- Gunston, B. (2005). World encyclopedia of aero engines from the pioneers to the present day (5th ed.). Stroud: Sutton Publishing.
- Lee, J. J., Kang, D. W., & Kim, T. S. (2011). Development of a gas turbine performance analysis program and its application. Energy, 36, 5274–5285.10.1016/j.energy.2011.06.032
- Lefebvre, A. H. (1998). Gas turbine combustion (2nd ed.). Abingdon: Taylor and Francis.
- Nkoi, B., Pilidis, P., & Nikolaidis, T. (2013a). Performance assessment of simple and modified cycle turboshaft gas turbines. Propulsion and Power Research, 2, 96–106. doi:10.1016/j.jppr.2013.04.009
- Nkoi, B., Pilidis, P., & Nikolaidis, T. (2013b). Performance of small-scale aero-derivative industrial gas turbines derived from helicopter engines. Propulsion and Power Research, 2, 243–253.
- Palmer, J. R. (1999). The TURBOMATCH scheme for aero/industrial gas turbine engine design point/off-design performance calculation (SoE, User Guide). Bedfordshire: Department of Power and Propulsion, Cranfield University.
- Roux, E. (2011). Turbofan and turbojet engines database handbook (2nd ed.). Elodie Roux.
- Safran Snecma. (2011). Commercial aircraft engines CFM56-5C. Evry Cedex: Engine Specification Brochure.
- Sethi, V. (2014). Gas turbine combustors (Thermal Power MSc course manual, Lecture). Bedfordshire: Department of Power and Propulsion, Cranfield University.
- Walsh, P. P., & Fletcher, P. (2004). Gas turbine performance (2nd ed.). Delhi: Blackwell.10.1002/9780470774533