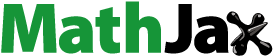
Abstract
Compromised balance with respect to both stable and passively unstable surfaces can potentially affect performance of many occupational, recreational and daily living tasks. Many tasks that require some level of balance maintenance, however, also involve burdens on individuals’ cognitive systems. Consequently the purpose of this project was to investigate the effects of performing cognitive tasks on stable surface and passively unstable surface balance performance. Participants in this study performed balance task on a passible unstable surface, as well as on a stable surface, with and without concurrent quantitative and language based cognitive tasks. Concurrent cognitive tasks did not demonstrate statistically significant effects on stable surface balance. For unstable surface balance, concurrent cognitive tasks had significant effects on trunk and arm segment angular velocities, while significant effects on movement of the passively unstable surface were not observed. The lack of cognitive task effects on stable surface balance suggests that such balance is well maintained with less than full attention. While overall unstable surface balance performance (i.e. unstable surface movement) was also not significantly affected by cognitive task execution, increased trunk and upper extremity movement during cognitive tasks suggests that cognitive tasks can trigger shifting of strategies for passively unstable surface balance.
Public Interest Statement
Insight into concurrent cognitive task effects on strategies and performance of balance on passively unstable surfaces can contribute substantially to the development and usefulness of quantitative balance measures as evaluation tools. The present study’s results demonstrated that diverting attentional resources from a primary unstable surface balance tack can lead to shifting of strategies toward more upper body and upper extremity approaches. Such knowledge may potentially assist future efforts to evaluate cognitive capacities or deficits.
1. Introduction
Numerous investigations have evaluated the effects of altered sensory components (e.g. vision, somatosensory) and physical task characteristics (e.g. foot placement, mass location) on stable (i.e. fixed) surface balance maintenance (Bisson, Chopra, Azzi, Morgan, & Bilodeau, Citation2010; Costello, Matrangola, & Madigan, Citation2012; Hansson, Beckman, & Håkansson, Citation2010; Hunter & Hoffman, Citation2001; Kavounoudias, Roll, & Roll, Citation1998; Lemos, Imbiriba, Vargas, & Vieira, Citation2015; Meyer, Oddsson, & De Luca, Citation2004). Stable surface balance studies have commonly permitted subjects to direct undivided attention to their balance tasks, while sensory or physical parameters were altered. Considerably less effort, however, has been devoted to investigating balance on passively unstable surfaces. The investigator has previously defined passively unstable surfaces as non-fixed surfaces whose movements are dynamically linked to individuals’ movement responses (Quesada, Durham, Topp, Swank, & Biton, Citation2007). Passively unstable surfaces that are encountered in various common settings (e.g. occupational, recreational, and daily living environments) can include: small boat decks, scaffolding, and thick foam mats. The more limited volume of passively unstable surface balance research has similarly allowed full attention to be directed toward balance task performance (Quesada & Beck, Citation2012; Quesada, Nguyen, & Walz, Citation2011; Quesada et al., Citation2007).
Some stable surface balance investigations have imposed cognitive demands on participants during standing (Andersson, Hagman, Talianzadeh, Svedberg, & Larsen, Citation2002; Dault, Frank, & Allard, Citation2001; Hunter & Hoffman, Citation2001; Mitra, Knight, & Munn, Citation2013; Nafati & Vuillerme, Citation2011; Pellecchia, Citation2003; Riley, Baker, & Schmit, Citation2003; Stensdotter, Wanvik, & Lorås, Citation2013; Swan, Otani, & Loubert, Citation2007; Swan, Otani, Loubert, Sheffert, & Dunbar, Citation2004; Van Impe et al., Citation2013; Vuillerme, Nougier, & Teasdale, Citation2000). Such approaches were typically expected to affect cognitive resources that process sensory input and, subsequently determine motor actuations. Findings from such efforts have been mixed, with some reports finding negligible impact of concurrent cognitive tasks, and others suggesting that stable surface balance is either hindered or enhanced.
Many occupational, recreational, and daily living tasks, that require some level of passively unstable balance maintenance, also involve demands on individuals’ cognitive resources. In construction environments, for example, workers are commonly subjected to cognitive demands while on scaffolding, which has been identified as an unstable standing surface associated with reduced postural stability (Min, Kim, & Parnianpour, Citation2012; Simeonov & Hsiao, Citation2001). Recreational and competitive athletes often perform, with substantial cognitive demands, while on passively unstable surfaces such as foam mats and skate boards. Moreover, in some settings the consequences of balance loss or inadequate balance can be especially severe (Malta et al., Citation2012; Shishlov, Schoenfisch, Myers, & Lipscomb, Citation2011). Balancing on stable surfaces may be generally less demanding, however, some tasks performed on stable surfaces can require particularly strong balance capacity, in conjunction with cognitive task performance. Consequently the purpose of this project was to investigate the effects of performing arithmetic and language cognitive tasks on passively unstable surface balance performance, as well as stable surface balance performance.
2. Methods
2.1. Participants
Ten healthy subjects (six males, four females), who composed a convenience sample drawn from the University of Louisville community, participated in this study. All participants were without any neuromuscular, musculoskeletal, vestibular or cardiovascular disorder that would preclude them standing on a stable or passively unstable surface for an extended duration. Means and standard deviations of their ages, weights, and heights were 21.5 ± 2.1 years, 721 ± 85 Newtons, and 176 ± 6 cm. Each individual signed an informed consent form that was approved by this institution’s IRB.
2.2 Data collection
A basic “wobble board” was selected to serve as a passively unstable surface. A wobble board consists of a flat circular board (diameter typically ranging from about 35 to 50 cm) with a spherical section attached to the lower surface (Figure ). The wobble board used in the present study had a surface diameter of approximately 40 cm. Balance maintenance on a wobble board requires an individual to respond dynamically to the board’s movement, while board movement responds passively to an individual’s actions. Consequently, this device conformed to the established definition of a passively unstable surface.
Each passively unstable surface balance task involved standing on the wobble board’s upper surface while attempting to keep the board as still as possible, as well as to prevent edge/ground contact. To diminish effects on task performance due to varied foot placement, feet were positioned for each unstable surface balancing trial, such that the dorsal creases were aligned along a wobble board diameter line. Subjects were provided an opportunity to familiarize (i.e. “warm up”) with the wobble board, prior to testing, to mitigate the potential for short term learning effects, and to facilitate trials that were representative of subjects’ balance capacity on passively unstable surfaces.
For each stable surface balancing trial the subject was instructed to stand as still as possible on a fixed, strain gage, force platform (Bertec Corporation; Columbus, Ohio). Feet were positioned shoulder width apart, with no stagger. Each participant was also instructed to orient facing the laboratory’s positive, horizontal I-axis. Consequently, fore/aft subject movement was in the laboratory’s I-axis direction, while medial/lateral movement was in the laboratory’s J-axis (i.e. horizontal and perpendicular to the I-axis). Subjects were assumed to be inherently familiar with standing on a stable surface, so no warm-up period was provided for the stable surface balance task.
For each passively unstable surface balance task, and each stable surface balance task, the trial duration was 45 s. For each type of balance task, trials were performed: (i) with no additional cognitive demands, (ii) while counting backwards in increments of seven from a given initial three digit number (i.e. while performing a “serial sevens” task), and (iii) while generating words beginning with a given letter (i.e. while performing a “verbal fluency” task). The no cognitive task condition represented a baseline scenario. The cognitive serial sevens and verbal fluency tasks in conditions (ii) and (iii) were intended to involve arithmetic and language cognitive resources, respectively (Francis, Franz, O’Connor, & Thelen, Citation2015; Tangen, Engedal, Bergland, Moger, & Mengshoel, Citation2014).
To enable three-dimensional quantification of wobble board orientation during unstable balance task trials, three passive reflective markers were placed (with approximately 90º interval spacing) at the board’s right, left, and rear edges (relative to a subject’s position; Figure ). Reflective markers were also placed on each subject’s body, in a Helen Hayes arrangement, to enable computation of body segment kinematics.
For all trials, marker spatial coordinates (with respect to a lab-based coordinate system) were obtained, at a 100 Hz sampling frequency, with a Hawk Motion Tracking System (Motion Analysis Corporation; Santa Rosa, California). For each stable surface balance task trial, ground reaction force data were obtained, at a 1,000 Hz sampling frequency, with a strain gage, force platform (Bertec Corporation; Columbus, Ohio).
2.3 Data processing
For passively unstable surface balance trials, wobble board marker coordinate trajectories were used to compute a tilt angle, θtilt, at each sampling frame. This metric represented overall wobble board rotational displacement relative to a horizontal plane (Figure ). To compute θtil, a unit vector perpendicular to the wobble board surface, uperp, was calculated by normalizing the cross product of vectors from the rear to right edge markers, and from the rear to left edge markers,(1)
(1)
Recognizing that the scalar product of uperp and the global (i.e. lab coordinate system based) vertical unit vector, K, was equal to the product of their magnitudes and the cosine of the angle (i.e. the tilt angle) between uperp and K,(2)
(2)
and noting that the magnitudes of uperp and K are unity, θtil was calculated as(3)
(3)
For each passively unstable surface trial, overall balance performance was quantified by determining the standard deviation (θtil, SD) of θtil across all sampling frames. Consequently, greater θtil, SD indicated greater variability of wobble board surface orientation, and was associated with poorer overall passively unstable balance performance.
Wobble board reflective marker coordinates were also used, with standard Euler angle based computations (Winter, Citation2005), to determine board surface angular velocity (ωbrd) at each sampling frame (other than first and last). Mean wobble board angular velocity (ωbrd, mean) was then determined across all sampling frames. Greater ωbrd, mean indicated more rapid wobble board movement, and was considered to be an additional indicator of poorer overall performance of the passively unstable surface balance task.
Body marker coordinates, at each sampling frame for each passively unstable surface trial, were used to compute segment angular velocities for the trunk (ωtrk), lower arms (ωla), and upper arms (ωua). Means for these segment angular velocities (ωtrk, mean, ωla, mean, ωua, mean) were then determined across all sampling frames. Mean trunk angular velocity represented a more body mass center related measure of balance performance. Arm segment angular velocity means quantified the level to which individuals employed compensatory upper extremity strategies for passively unstable surface balance maintenance.
For each stable surface balance trial, center of pressure coordinates at each sampling frame were extracted from the ground reaction force recording. Fore/aft stable surface balance was quantified by the computing the standard deviation of I-axis center of pressure coordinates (COPF/A, SD) across all sampling frames. Medial/lateral stable surface balance was similarly quantified as the standard deviation of J-axis center of pressure coordinates (COPM/L, SD). In this widely employed approach to quantifying stable surface balance performance (Hunter & Hoffman, Citation2001; Pellecchia, Citation2003; Swan et al., Citation2007), greater COP variability is generally associated with greater postural sway. Consequently, greater values of COPF/A, SD and COPM/L, SD are related to poorer stable surface balance performance.
2.4. Statistical analysis
Reported metrics were initially statistically processed, using single factor, three level (no cognitive task, serial sevens task, verbal fluency task) repeated measures analysis of variance (ANOVA), to assess whether statistically significant differences (α level = 0.05) existed between any conditions. No further processing was conducted for ANOVAs with p-values above the indicated α level. For ANOVAs with p-values below the indicated α level), post hoc paired t-tests were conducted to identify the conditions for which significant differences were exhibited.
3. Results
For stable surface balance, mean values of fore/aft center of pressure standard deviations were COPF/A, SD = 5.75 ± 2.85, 9.71 ± 9.81, and 7.11 ± 5.39 mm, with no cognitive task, serial sevens task, and verbal fluency task, respectively (Figure ). Analysis of variance indicated no significant differences (p = 0.16) for COPF/A, SD between any of the conditions. The corresponding mean values for medial/lateral COP standard deviations were COPM/L, SD = 2.38 ± 1.14, 3.96 ± 3.37, and 3.32 ± 2.13 mm (Figure ). Analysis of variance indicated no significant differences (p = 0.22) for COPM/L, SD between any of the conditions. Large standard deviation to mean ratios for cognitive task conditions raised concerns that the parametric statistics assumptions were substantially violated, and prompted additional non-parametric statistical evaluation, using the Friedman test. This test also indicated a lack of statistically significant effect of concurrent cognitive tasks on stable surface balance (p = 0.74 for both COPF/A, SD and COPM/L, SD).
Figure 4. Fore/aft and medial/lateral COP variability for stable surface balance with and without concurrent cognitive tasks.
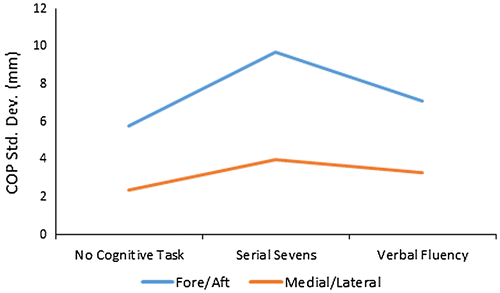
Mean values for wobble board based measures of passively unstable surface balance were θtil, SD = 2.41 ± 0.34º, 2.94 ± 0.83º, and 2.83 ± 0.66º; and ωbrd, mean = 12.71 ± 2.65, 14.83 ± 5.79, and 15.23 ± 5.26º/s (Figure ), with no cognitive task, serial sevens task, and verbal fluency task. For θtil, SD and ωbrd, mean, no significant differences (p = 0.12 and 0.13) were found, via ANOVA, between any of the conditions.
Figure 5. Wobble board based measures of passively unstable surface balance performance with and without concurrent cognitive tasks.
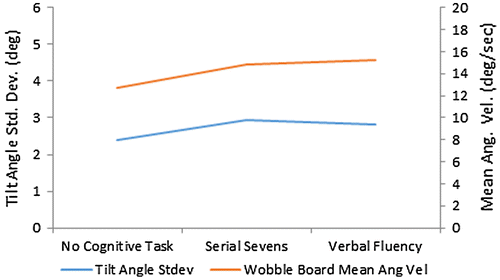
Mean values for the body mass center related measure were ωtrk, mean = 6.51 ± 3.16, 10.56 ± 7.35, and 10.39 ± 6.14º/s (Figure ). Analysis of variance detected significant ωtrk, mean differences between conditions (p = 0.01); and subsequent pairwise comparisons indicated that ωtrk, mean differed, from baseline, for both serial sevens task (p = 0.05), and verbal fluency task (p = 0.02).
Figure 6. Mean trunk and arm segment angular velocities during passively unstable surface balance performance with and without concurrent cognitive tasks.
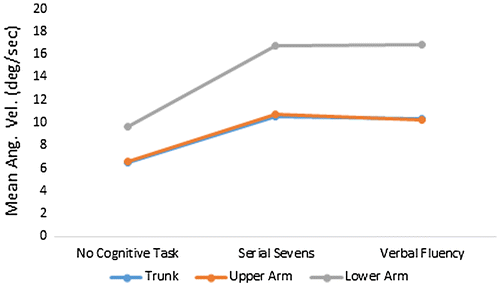
Mean arm segment angular velocities during passively unstable surface balance tasks, with no cognitive task, serial sevens task, and verbal fluency task, were ωla, mean = 9.61 ± 7.87, 16.74 ± 14.16, and 16.86 ± 13.14º/s; and ωua, mean = 6.55 ± 5.24, 10.76 ± 9.87, and 10.21 ± 8.45º/s (Figure ). For mean arm segment angular velocities, ANOVA detected significant differences between cognitive task conditions for ωla, mean (p < 0.001) and ωua, mean (p < 0.001). Pairwise comparisons, subsequent to ANOVA, indicated that serial sevens task effects were significant for ωua, mean (p = 0.005) and ωua, mean, (p = 0.008). Verbal fluency task effects were significant for ωua, mean (p = 0.001) and ωua, mean, (p = 0.004).
4. Discussion
During stable surface balance, lack of statistically significant cognitive task effects, indicates that balancing on a stable surface is substantially maintained with less than full attention available. Such results are consistent with a number of reports that have not observed stable surface balance decrements with concurrent cognitive tasks (Maylor & Wing, Citation1996; Yardley, Gardner, Leadbetter, & Lavie, Citation1999). While stable surface balance decrements with concurrent cognitive tasks have also been reported (Pellecchia, Citation2003), Yardley et al. (Citation1999) have suggested that verbal articulation of cognitive task responses (i.e. speaking numbers/words aloud) can contribute to such decrements. In the current study, it is noted that the lack of significant cognitive effects on stable surface balance was observed, even with verbal articulation of cognitive responses. Some previous investigators have actually observed stable surface balance enhancement with cognitive task execution (Broglio, Tomporowski, & Ferrara, Citation2005; Dault et al., Citation2001; Hunter & Hoffman, Citation2001; Swan et al., Citation2004). However, other similar reports have indicated that stable surface balance enhancement is associated with some concurrent cognitive tasks (particularly more difficult cognitive tasks), but not all types (Riley et al., Citation2003; Swan et al., Citation2007).
Lack of cognitive task effects, on wobble board based metrics (θtil, SD, ωbrd, mean) during passively unstable surface balance, suggests that with or without cognitive tasks, individuals were able to perform the passively unstable surface balance task at similar levels, with specific regard to the instruction to keep the wobble board as still as possible. Such findings in isolation, however, do not facilitate insight regarding whether this passively unstable surface balance task (i.e. keeping wobble board still) is sufficiently non-challenging to tolerate reduced attention availability, or whether use of additional/alternate strategies contributes to maintaining performance of the passively unstable surface balance task, as instructed. Clarification in this regard would be desirable, since use of additional/alternate strategies, during this task, could involve increases in body segment motions, and the motor actuations causing them. From a dynamic systems perspective such additional actuations would modify the process for passively unstable surface balance with concurrent cognitive tasks, relative to the process without cognitive tasks.
Significant cognitive task effects on trunk and upper extremity based metrics (ωtrk, mean, ωla, mean, ωua, mean) during passively unstable surface balance, indicated that greater use of upper body and upper extremity strategies was employed when reduced conscious attention was available. It was additionally noted that multiple subjects commented on not being aware of increased reliance on upper body/extremity strategies.
Significant cognitive effects on upper body/extremity strategies do not appear inconsistent with lack of cognitive task effects, on wobble board based metrics (θtil, SD, ωbrd, mean). Rather, reduced upper body/extremity reliance, when no concurrent cognitive task is performed, suggests general preference for lower extremity strategies, despite potential effectiveness of upper extremity usage. Conversely, increased use of upper extremity strategies, with reduced attentional resources, could suggest that: predominant use of lower extremity strategies requires full attention to effectively maintain control of passively unstable surface balance; subconscious motor controllers have greater affinity for use of upper extremity strategies; or some combination of these scenarios.
A lower extremity strategy dependence on attentional resources would seem to be plausible and to suggest that less desirable strategies may be employed when preferred strategies lose effectiveness. Such a notion seems consistent with observations of shifting, from ankle to hip strategies, which have been described with balance tasks that did not involve a passively unstable surface, but were more demanding than simple unimpaired fixed surface standing (Dokka, Kenyon, & Keshner, Citation2009; Gatev, Thomas, Kepple, & Hallett, Citation1999; Joseph Jilk, Safavynia, & Ting, Citation2014; Runge, Shupert, Horak, & Zajac, Citation1998). Balance task challenges that have been linked to strategy shifting include vision obstruction, narrowed stance width, and virtual scene oscillations. While ankle strategies would seem to be desirable for balance on more stable surfaces, ankle strategy use has been associated with feedforward mechanisms, which would likely require greater attentional resources than feedback mechanisms (Gatev et al., Citation1999).
Subconscious controller preference for increased upper extremity balance strategies would involve the notion that conscious and subconscious motor controllers have differing mechanism tendencies. Such a predisposition could potentially be associated with either sensory/motor reflex loops, which are inherently regulated at subconscious levels, having shorter durations than conscious voluntary actuation cycles (Tsao, Galea, & Hodges, Citation2009); or with specific inclinations for different uses of various body segments (Walker & Perreault, Citation2015). Descriptions of stable surface balance mechanisms have generally not included upper extremity strategies, and have been limited to ankle and hip strategies (Dokka et al., Citation2009; Gatev et al., Citation1999; Joseph Jilk et al., Citation2014; Kuo & Zajac, Citation1993; Runge et al., Citation1998). Substantive upper extremity movements would likely induce increased overall body excursions (and increased COP variability) that are typically not desired during stable surface balance. However, potential for controller preferences to expand beyond ankle and hip strategies, for even fixed surface balance, might become greater as contact area with a standing surface decreases (e.g. standing on a gymnastics balance beam or a protruding rock). Such fixed surface balance with small contact areas can be associated with increased use of upper extremity strategies, more similar to those observed with passively unstable surfaces.
The present study involved limitations that should be considered when assessing results. The results reported here represent a single trial for each condition, and were obtained at a single session for each participant. However, it is noted that when the investigators have obtained multiple trials per condition have been obtained, the reported metrics have demonstrated reproducibility within a subject during a given session (Quesada & Beck, Citation2012). It is also noted that, at baseline (i.e. no imposed conditions), these metrics have been considerably consistent across multiple investigations at this site (Quesada & Beck, Citation2012; Quesada & Davidson, Citation2013; Quesada et al., Citation2011). For passively unstable surface balance tasks, wobble board based metrics (θtil, SD, ωbrd, mean) were selected to quantify overall performance, in conjunction with specific instructions to keep the wobble board as still as possible. However, some subjects may have internally interpreted the task objective to include keeping the whole body as still as possible. Wulf, Töllner, and Shea (Citation2007) have suggested instructions to subjects may affect balance performance on more difficult standing surfaces. Application of such findings to the current study might imply that differences in potential interpretation of instructions might mask whether greater upper extremity strategies are inherently present during passively unstable surface balance, by potentially elevating a participant’s effort to minimize upper extremity motion when not performing concurrent cognitive tasks. While all participants were able to complete all trials on the wobble board, participants were all relatively young and healthy. Persons with actual motor, sensory and/or cognitive deficits might not tolerate trials with the wobble board used in this study. Caution is likely warranted when extrapolating interpretations to populations with such deficits. Similar caution is also appropriate when generalizing to other types of passively unstable surfaces. Some passively unstable surface may not return to an initial orientation when unloaded (e.g. a muddy natural turf field); or they may provide for independent foot orientations (e.g. a foam mat).
Presence of significant experimental findings and potential associated implications provides motivation for additional study. Such efforts should investigate whether imposing concurrent cognitive tasks while restricting upper extremity movement associates with poorer unstable surface balance performance. Balance on other types of passively unstable surfaces should also be studied to assess which aspects of passively unstable surface balance can be generalized, and which ones are more surface specific. Additionally, other populations that are associated with motor, sensory, cognitive, or other deficits should be included in future investigations. The elderly are particularly more prone to balance relevant conditions, including muscle weakness, visual impairment and cognitive deficits.
Funding
The authors received no direct funding for this research.
Additional information
Notes on contributors
Peter M. Quesada
Peter M. Quesada received the PhD degree in bioengineering from the University of California, Berkeley and San Francisco, in 1991. He is currently a Professor in the University of Louisville’s Department of Mechanical Engineering, where he directs the Motion Biomechanics Laboratory, within the Speed School of Engineering’s Center for Ergonomics. He also serves the Mechanical Engineering Department’s Associate Chair and Director of Graduate Studies. He has served as a reviewer of biomechanically related manuscripts for several journals. His research interests include the biomechanics of human movement and its applications in rehabilitation, orthopedics, athletic performance, and ergonomics. Dr Quesada has served on the Executive Committee of the Gait and Clinical Movement Analysis Society.
References
- Andersson, G., Hagman, J., Talianzadeh, R., Svedberg, A., & Larsen H. C. (2002). Effect of cognitive load on postural control. Brain Research Bulletin, 58, 135–139. PII: S0361-9230(02)00770-010.1016/S0361-9230(02)00770-0
- Bisson, E. J., Chopra, S., Azzi, E., Morgan, A., & Bilodeau, M. (2010). Acute effects of fatigue of the plantarflexor muscles on different postural tasks. Gait & Posture, 32, 482–486. doi:10.1016/j.gaitpost.2010.07.006
- Broglio, S. P., Tomporowski, P. D., & Ferrara, M. S. (2005). Balance performance with a cognitive task: A dual-task testing paradigm. Medicine & Science in Sports & Exercise, 37, 689–695. doi:10.1249/01.MSS.0000159019.14919.09
- Costello, K. E., Matrangola, S. L., & Madigan, M. L. (2012). Independent effects of adding weight and inertia on balance during quiet standing. BioMedical Engineering OnLine, 11, 20. doi:10.1186/1475-925X-11-20
- Dault, M. C., Frank, J. S., & Allard, F. (2001). Influence of a visuo-spatial, verbal and central executive working memory task on postural control. Gait and Posture, 14, 110–116. PII: S0966-6362(01)00113-810.1016/S0966-6362(01)00113-8
- Dokka, K., Kenyon, R. V., & Keshner, E. A. (2009). Influence of visual scene velocity on segmental kinematics during stance. Gait & Posture, 30, 211–216. doi:10.1016/j.gaitpost.2009.05.001
- Francis, C. A., Franz, J. R., O’Connor, S. M., & Thelen, D. G. (2015). Gait variability in healthy old adults is more affected by a visual perturbation than by a cognitive or narrow step placement demand. Gait & Posture, 42, 380–385. doi:10.1016/j.gaitpost.2015.07.006
- Gatev, P., Thomas, S., Kepple, T., & Hallett, M. (1999). Feedforward ankle strategy of balance during quiet stance in adults. The Journal of Physiology, 514, 915–928. doi:10.1111/j.1469-7793.1999.915ad.x
- Hansson, E. E., Beckman, A., & Håkansson, A. (2010). Effect of vision, proprioception, and the position of the vestibular organ on postural sway. Acta Oto-Laryngologica, 130, 1358–1363. doi:10.3109/00016489.2010.498024
- Hunter, M. C., & Hoffman, M. A. (2001). Postural control: Visual and cognitive manipulations. Gait and Posture, 13, 41–48. PII: S0966-6362(00)00089-810.1016/S0966-6362(00)00089-8
- Joseph Jilk, D. J., Safavynia, S. A., & Ting, L. H. (2014). Contribution of vision to postural behaviors during continuous support-surface translations. Experimental Brain Research, 232, 169–180. doi:10.1007/s00221-013-3729-4
- Kavounoudias, A., Roll, R., & Roll, J. P. (1998). The plantar sole is a ‘dynamometric map’ for human balance control. NeuroReport, 9, 3247–3252.10.1097/00001756-199810050-00021
- Kuo, A. D., & Zajac, F. E. (1993). Human standing posture: multi-joint movement strategies based on biomechanical constraints. Progress in Brain Research, 97, 349–358.10.1016/S0079-6123(08)62294-3
- Lemos, T., Imbiriba, L. A., Vargas, C. D., & Vieira, T. M. (2015). Modulation of tibialis anterior muscle activity changes with upright stance width. Journal of Electromyography and Kinesiology., 25, 168–174. doi:10.1016/j.jelekin.2014.07.009
- Malta, D. C., Silva, M. M., Mascarenhas, M. D., Sá, N. N., Morais Neto, O. L., Bernal, R. T., … Gawryszewski, V. P. (2012). Características e fatores associados às quedas atendidas em serviços de emergência [Characteristics and factors associated with falls met in emergency services]. Revista de Saúde Pública [Journal of Public Health], 46, 128–137. S0034-8910201200010001610.1590/S0034-89102012000100016
- Maylor, E. A., & Wing, A. M. (1996). Age differences in postural stability are increased by additional cognitive demands. The Journals of Gerontology Series B: Psychological Sciences and Social Sciences, 51B, P143–P154.10.1093/geronb/51B.3.P143
- Meyer, P. F., Oddsson, L. I. E., & De Luca, C. J. (2004). The role of plantar cutaneous sensation in unperturbed stance. Experimental Brain Research, 156, 505–512. doi:10.1007/s00221-003-1804-y
- Min, S. N., Kim, J. Y., & Parnianpour, M. (2012). The effects of safety handrails and the heights of scaffolds on the subjective and objective evaluation of postural stability and cardiovascular stress in novice and expert construction workers. Applied Ergonomics, 43, 574–581. doi:10.1016/j.apergo.2011.09.002
- Mitra, S., Knight, A., & Munn, A. (2013). Divergent effects of cognitive load on quiet stance and task-linked postural coordination. Journal of Experimental Psychology: Human Perception and Performance, 39, 323–328. doi:10.1037/a0030588
- Nafati, G., & Vuillerme, N. (2011). Decreasing internal focus of attention improves postural control during quiet standing in young healthy adults. Research Quarterly for Exercise & Sport, 82, 634–643. doi:10.1080/02701367.2011.10599800
- Pellecchia, G. L. (2003). Postural sway increases with attentional demands of concurrent cognitive task. Gait and Posture, 18, 29–34. PII: S0966-6362(02)00138-810.1016/S0966-6362(02)00138-8
- Quesada, P. M., & Beck,E. M. (2012, May). Effects of added head and torso mass on unstable surface balance performance. Paper presented at the Annual Meeting of the Gait and Clinical Movement Analysis Society, Grand Rapids, MI.
- Quesada, P. M., & Davidson, A. (2013, May). Effects of added head and torso mass on unstable surface balance performance. Paper presented at the Annual Meeting of the Gait and Clinical Movement Analysis Society, Cincinnati, OH.
- Quesada, P. M., Durham, M. P., Topp, R. V., Swank, A. M., & Biton, D. (2007). Quantitative assessment of balance performance on a passively unstable surface. Occupational Ergonomics, 7, 3–10.
- Quesada, P. M., Nguyen, H., & Walz, R. D. (2011, April). Effects of visual impairment on stable and unstable surface balance. Paper presented at the Annual Meeting of the Gait and Clinical Movement Analysis Society, Denver, CO.
- Riley, M. A., Baker, A. A., & Schmit, J. M. (2003). Inverse relation between postural variability and difficulty of a concurrent short-term memory task. Brain Research Bulletin, 62, 191–195. doi:10.1016/j.brainresbull.2003.09.012
- Runge, C. F., Shupert, C. L., Horak, F. B., & Zajac, F. E. (1998). Role of vestibular information in initiation of rapid postural responses. Experimental Brain Research, 122, 403–412. doi:10.1007/s002210050528
- Shishlov, K. S., Schoenfisch, A. L., Myers, D. J., & Lipscomb, H. J. (2011). Non-fatal construction industry fall-related injuries treated in US emergency departments, 1998-2005. American Journal of Industrial Medicine, 54, 128–135. doi:10.1002/ajim.20880
- Simeonov, P., & Hsiao, H. (2001). Height, surface firmness, and visual reference effects on balance control. Injury Prevention, 7(90001), 50i–53.10.1136/ip.7.suppl_1.i50
- Stensdotter, A.-K., Wanvik, A. K., & Lorås, H. W. (2013). Postural control in quiet standing with a concurrent cognitive task in psychotic conditions. Journal of Motor Behavior, 45, 279–287. doi:10.1080/00222895.2013.791241
- Swan, L., Otani, H., & Loubert, P. (2007). Reducing postural sway by manipulating the difficulty levels of a cognitive task and a balance task. Gait & Posture, 26, 470–474. doi:10.1016/j.gaitpost.2006.11.201
- Swan, L., Otani, H., Loubert, P. V., Sheffert, S. M., & Dunbar, G. L. (2004). Improving balance by performing a secondary cognitive task. British Journal of Psychology, 95, 31–40.10.1348/000712604322779442
- Tangen, G. G., Engedal, K., Bergland, A., Moger, T. A., & Mengshoel, A. M. (2014). Relationships between balance and cognition in patients with subjective cognitive impairment, mild cognitive impairment, and Alzheimer disease. Physical Therapy, 94, 1123–1134. doi:10.2522/ptj.20130298
- Tsao, H., Galea, M. P., & Hodges, P. W. (2009). How fast are feedforward postural adjustments of the abdominal muscles? Behavioral Neuroscience, 123, 687–693.10.1037/a0015593
- Van Impe, A., Bruijn, S. M., Coxon, J. P., Wenderoth, N., Sunaert, S., Duysens, J., & Swinnen, S. P. (2013). Age-related neural correlates of cognitive task performance under increased postural load. Age, 35, 2111–2124. doi:10.1007/s11357-012-9499-2
- Vuillerme, N., Nougier, V., & Teasdale, N. (2000). Effects of a reaction time task on postural control in humans. Neuroscience Letters, 291, 77–80. doi:10.1016/S0304-3940(00)01374-4
- Walker, E. H., & Perreault, E. J. (2015). Arm dominance affects feedforward strategy more than feedback sensitivity during a postural task. Experimental Brain Research, 233, 2001–2011. doi:10.1007/s00221-015-4271-3
- Winter, D. A. (2005). Biomechanics and motor control of human movement (3rd ed.).Hoboken, NJ: Wiley & Sons.
- Wulf, G., Töllner, T., & Shea, C. H. (2007). Attentional focus effects as a function of task difficulty. Research Quarterly for Exercise and Sport, 78, 257–264. doi:10.1080/02701367.2007.10599423
- Yardley, L., Gardner, M., Leadbetter, A., & Lavie, N. (1999). Effect of articulatory and mental tasks on postural control. NeuroReport, 10, 215–219.10.1097/00001756-199902050-00003