Abstract
The sulfonation of poly(p-phenylenediamine) (P(pPD)) polymer was accomplished by oxidative copolymerization of p-phenylenediamine (pPD) with 2,5-diaminobenzenesulfonic acid (DABSA) and 2-aminobenzenesulfonic acid (2ABSA) monomers with 50/50 mol% ratios, using ammonium persulfate ((NH4)2S2O8) as an oxidant. The pPD-co-DABSA and pPD-co-3ABSA copolymers obtained were fully characterized and compared by Fourier transform infrared spectroscopy (FT-IR), 1H nuclear magnetic resonance spectroscopy (1H NMR), Gel permeation chromatography (GPC), Wide-angle X-ray diffraction (WAXD) and Thermogravimetric analysis (TGA). The yield, molecular weight, solubility, degree of sulfonation, molecular structure and thermal stability of the copolymers were shown to be dependent on the type of comonomer. The solubility of pPD-co-DABSA and pPD-co-2ABSA copolymers was significantly improved compared to the P(pPD) polymer in most solvents. Conversely, the yield and molecular weight values obtained for the pPD-co-DABSA and pPD-co-2ABSA copolymers were lower than those obtained for P(pPD) polymer. The TGA results showed that P(pPD), pPD-co-DABSA and pPD-co-2ABSA copolymers have good thermal stability and decompose above 200°C in nitrogen.
Public Interest Statement
In the last few decades, poly(p-phenylenediamine) (P(pPD)) as a typical conductive polymer has attracted a large attention from researchers because of its varied structure, high environmental and thermal stability, adsorption of heavy metal ions and attractive application in technology. Conversely, the poor mechanical properties, infusibility and slightly solubility of P(pPD) homopolymer in most common organic solvents have limited it from widely practical use. Therefore, this study focused on improving the solubility, maintaining the original functionalities and providing novel interesting performances of P(pPD) polymers. This was done by introducing of sulfonic groups to P(pPD) polymers which greatly improved the solubility, fusibility, processability, environmental stability and optical properties without substantially losing conductivity of the P(pPD) polymer materials.
1. Introduction
Poly(p-phenylenediamine) (P(pPD)) as a typical conductive polymer has attracted a large attention from researchers just as other polymers such as biopolymer Chitosan, because of their varied structure, high environmental and thermal stability, adsorption of heavy metal ions, energy storage and attractive application in technology (Huang, Lu, & Li, Citation2012; Li, Huang, Duan, & Yang, Citation2002; Ramkumar & Sundaram, Citation2016). Conversely, the poor mechanical properties, infusibility and slightly solubility of P(pPD) homopolymer in most common organic solvents cause difficulties in the determination of the molecular structure and restrict it from widely practical use (Cataldo, Citation1996; Li, Huang, & Yang, Citation2001). Therefore, it is interesting to design structures with the purpose of increasing the fusibility and solubility, thus improving the processability of P(pPD) polymer. However, to improve the solubility of P(pPD) polymer and maintain the original functionalities, partial phenazine structure and in addition provide novel interesting performances, introduction of sulfonic groups to aromatic polymers can greatly improve the solubility, environmental stability and optical properties without substantially losing conductivity of aromatic polymer materials (Mav, Zigon, Sebenik, & Vohlidal, Citation2000; Mav-Golež, Pahovnik, Bláha, Žigon, & Vohlídal, Citation2011; Tsuchida, Kaneko, & Kurimura, Citation1970).
Sulfonated aromatic polymers (SAPs) are being largely investigated due to their potential applications in specific areas, such as catalytic coatings electrodialysis, rechargeable batteries, light-emitting diodes, junction devices, enzymatic activity, corrosion protection, fuel cells, ionophores and absorbents towards heavy metal ions (Huang et al., Citation2014; Pradeep, Arunraj, & Dhatchinamurthy, Citation2014). Thus, several aromatic polymers have been sulfonated over the last few years, such as polyaniline (PAN) (Mav et al., Citation2000; Mav-Golež et al., Citation2011; Tsuchida et al., Citation1970), polyimides (Genies et al., Citation2001), polysulfones (Drioli et al., Citation2004), poly(etherketones) (Gil et al., Citation2004), polyamides (Nolte, Ledjeff, Bauer, & Mülhaupt, Citation1993), polyarylene(ether sulfones) (Qi & Pickup, Citation1998), substituted poly(1,4-phenylenes) (Benavente, Garcı́a, Riley, Lozano, & de Abajo, Citation2000), poly(phenylene sulfides) (Qi, Lefebvre, & Pickup, Citation1998). The percentage of sulfonation which is defined as the molar ratio of sulfur to nitrogen in sulfonated aromatic copolymers can be controlled by selecting varying of experimental conditions and by choosing various molar proportions of the two monomers.
The P(pPD) polymer sulfonation using 2,5-diaminobenzenesulfonic acid (DABSA) and 2-aminobenzenesulfonic acid (2ABSA) is described in this study. The purpose of the study was to obtain sulfonated P(pPD) polymers (SP(pPD)) by copolymerization of pPD with DABSA and 2ABSA monomers, as can be seen in Scheme . The obtained pPD-co-DABSA and pPD-co-2ABSA copolymers were fully characterized and presented in this paper as shown in Table .
Table 1. Properties of the P(pPD), pPD-co-DABSA and pPD-co-2ABSA copolymers prepared by chemical oxidative polymerization
2. Experimental
2.1. Materials
The pPD, 2ABSA, ammonium persulfate ((NH4)2S2O8) and all other solvents and reagents were obtained from Fluka or Aldrich and were used as received.
2.2. Preparation of P(pPD) polymer and pPD-co-DABSA and pPD-co-2ABSA copolymers
Copolymerization reactions of with 50/50 molar ratios were carried out by chemical oxidative polymerization as follows: pPD (1.7 g (0.016 mol)) and DABSA (1.47 g (0.008 mol)) monomers were added to 150 mL of distilled water in a 500 mL glass flask in an oil bath at 70°C and was magnetically stirred under nitrogen atmosphere. In a separate flask, the oxidant solution consisting of (NH4)2S2O8 (4.0 g) in 40 mL of distilled water was prepared. The polymerization reaction was started by steadily adding oxidant solution dropwise into the monomer solution for 20 min. The reaction mixture was continuously stirred for 24 h. The precipitated copolymer was then filtered, washed carefully with sufficient water until the filtered solution was colorless, to remove any unreacted monomers and oligomers. The precipitated copolymer obtained was vacuum-dried for 24 h at 50°C for further characterization studies. Similar procedure was done to copolymerize pPD with 2ABSA. The copolymers were assigned as pPD-co-DABSA 50/50 and pPD-co-2ABSA 50/50 in Table .
2.3. Equipment
Fourier transform infrared spectroscopy (FT-IR) was used to characterize the molecular structure of aromatic diamine polymers. Infrared spectra of the samples were recorded on a Bruker VERTEX 80 FT-IR spectrometer at room temperature. The analysis was performed between 500 and 4,000 cm−1. The resolution used was 2 cm−1 and the results were based on an average of 22 scans. Attenuated total reflection (ATR) method was used to measure powder samples. ATR method involved pressing the samples against a high-refractive-index prism and measuring the infrared spectrum using infrared light that is totally internally reflected in the prism.
1H nuclear magnetic resonance spectroscopy (1H NMR) spectra were recorded at room temperature on a 600 SB Ultra Shield™ Plus NMR spectrometer equipped with an Oxford magnet (14.09 T), operating at 600 MHz. Samples (20–30 mg) for NMR analyses were dissolved in deuterated dimethyl sulfoxide (DMSO-d6).
Gel permeation chromatography (GPC) was used to determine weight average molecular weight and polydispersity (PD). Samples were analyzed with a PL-GPC 220. A flow rate of 1.0 mL/min was used. The analyses were carried out in dimethylacetamide (DMAC).
Wide-angle X-ray diffraction (WAXD) analysis was performed on a Bruker AXS D8 Advance diffractometer at room temperature with filtered CuKα radiation. All samples were scanned at 2θ angles, ranging from 0° to 80°, with a sampling width of 0.02°, where 2θ is the diffraction angle. A few tenths of a gram of the polymer in a powder form was characterized using WAXD for each sample.
Thermogravimetric analysis (TGA) measures the change in the weight of polymer sample as a function of temperature, and was determined on a TGA Q100 instrument (TA Instruments) with a resolution of 0.1 mg, in a nitrogen atmosphere. Approximately 5–10 mg of each sample was analyzed by heating from 30° to 900°C at a rate of 10°C/min.
3. Results and discussion
3.1. Polymerization yield and molecular weight
In general, it was found that the oxidative polymerization yield and molecular weight of P(pPD) homopolymer higher than those of pPD-co-DABSA and pPD-co-2ABSA copolymers (Table ). The decrease of the yield values of the pPD-co-DABSA and pPD-co-2ABSA copolymers compared to the P(pPD) polymer could be due to the steric hindrances and the electro-withdrawing effects of the sulfonic groups (–SO3H) on the aromatic rings of pPD-co-DABSA and pPD-co-2ABSA copolymers (Mav-Golež et al., Citation2011). It seems that the attached sulfonic acid group makes oxidation of the phenylene ring in DABSA and 2ABSA difficult (Mav et al., Citation2000). As a result of the difficult oxidation of DABSA and 2ABSA phenylene rings; DABSA and 2ABSA do not homopolymerize under the experimental conditions used in this work, as shown in Table .
3.2. Solubility and degree of sulfonation of polymers
The solubility of pPD-co-DABSA and pPD-co-2ABSA copolymers was measured by adding 30 mL of the solvent to 300 mg of copolymer sample, followed by continuous stirring of the mixture for 2 h at room temperature; the solubility was measured quantitatively for all solvents. The sulfonation degrees (S/N molar ratios) of the pPD-co-DABSA and pPD-co-2ABSA copolymers were measured by elemental analysis (Table ). The P(pPD) polymer was mainly soluble in N-methylpyrrolidone (NMP), dimethyl sulfoxide (DMSO), dimethylformamide (DMF) and sulfuric acid (H2SO4) solvents, which are considered to be good solvents of PAN polymers as well (Li et al., Citation2002). The pPD-co-DABSA and pPD-co-2ABSA copolymers were relatively soluble in tetrahydrofuran (THF) and chloroform (CHCl3) solvents and slightly soluble in water, whereas the P(pPD) polymer was only partly soluble in THF and CHCl3 solvents and insoluble in water. This is in agreement with the results obtained by Li et al. for P(pPD) homopolymers (Li, Huang, Chen, Jin, & Yang, Citation2001; Li et al., Citation2002).
Table 2. Solubility data of P(pPD), pPD-co-DABSA and pPD-co-2ABSA copolymers in different solvents (at room temperature)
3.3. Molecular structure of polymers
3.3.1. FT-IR spectroscopy
FT-IR spectroscopy was used to verify whether or not pPD-co-DABSA and pPD-co-2ABSA copolymers were obtained. Figure shows the FT-IR spectra of P(pPD), pPD-co-DABSA 50/50 and pPD-co-2ABSA 50/50 copolymers. The FT-IR spectrum of the P(pPD) polymer was different compared to the FT-IR spectra of pPD-co-DABSA 50/50 and pPD-co-2ABSA 50/50 copolymers (Figure ). In general, FT-IR spectra of all polymers exhibited two or three broad peaks at 3,400–3,200 and 3,180–3,100 cm−1, suggesting N–H stretching vibrations of –NH and –NH2 groups, respectively (Cataldo, Citation1996; Li et al., Citation2001, 2002; Ramkumar & Minakshi, Citation2015). The polymers also showed strong peaks in the region between 1,620 and 1,700 cm−1, as well as between 1,500 and 1,540 cm−1, which were associated to the quinonoid and benzenoid rings repectively (Li et al., Citation2001). The two peaks at 1,400 and 1,140 cm−1 were attributed to the C–N stretching vibration in quinonoid imine units, whereas the peak positioned at 1,270–1,230 cm−1 was attributed to the C–N stretching in the benzenoid unit (Li et al., Citation2001, 2002). The peaks at 880 and 570 cm−1 were possibly due to the out-of-plane bending vibrations of the C–H of 1,2,4,5-tetrasubstituted benzene nuclei of phenazine units. The existence of –SO3H groups in pPD-co-DABSA and pPD-co-2ABSA copolymers was evident by the presence of peaks around 670, 750 and 1,040 cm−1 (Figure (b) and (c)). The peak at 1,040 cm−1 was caused by symmetric vibration of S=O stretching, confirming the presence of sulfonic acid groups in the copolymers (Huang et al., Citation2014; Lee, Lai, Wang, Jeng, & Lin, Citation2008; Mav-Golež et al., Citation2011; Pradeep et al., Citation2014). The peaks at 670 and 750 cm−1 were caused by the S–O and C–S aromatic stretching vibrations (Lee et al., Citation2008; Mav-Golež et al., Citation2011; Pradeep et al., Citation2014).
3.3.2. 1H NMR of the polymers
The 1H NMR spectra of the P(pPD), pPD-co-DABSA 50/50 and pPD-co-2ABSA 50/50 copolymers in deuterated DMSO are shown in Figure (a)–(c), respectively. The P(pPD), pPD-co-DABSA 50/50 and pPD-co-2ABSA 50/50 copolymers exhibited 1H NMR spectra relatively different from each other. The 1H NMR spectrum of the P(pPD) polymer (Figure (a)) was characterized by four major peaks, which correlates with the three types of protons on the polymer chains. The 1H NMR spectrum of the P(pPD) polymer displayed the strongest three peaks positioned at 7.1–7.3 ppm due to –NH– protons; and the peaks at 7.4 and 8.2 ppm due to the protons on 1,2,4,5-tetrasubstituted benzene rings (Li et al., Citation2001, 2002). The peaks attributed to the protons on 1,2,4,5-tetrasubstituted benzene rings were found at 7.0–7.5 and 7.3–8.3 ppm in the case of pPD-co-DABSA 50/50 and pPD-co-2ABSA 50/50 copolymers, respectively (Figure (b) and (c)).
3.3.3. Wide-angle X-ray diffractograms of polymers
Wide-angle X-ray diffractograms of P(pPD), pPD-co-DABSA 50/50 and pPD-co-2ABSA 50/50 copolymers are shown in Figure (a)–(c) respectively. There were clear differences between the XRD patterns of the P(pPD), pPD-co-DABSA 50/50 and pPD-co-2ABSA 50/50 copolymers. The P(pPD) polymer is amorphous and exhibited a major peak centered at 2θ = 26° and minor peak at 2θ = 6° (Figure (a)), which could be related to the presence of some large crystallite sizes (Li et al., Citation2001, 2001). Conversely, the peak at 2θ = 6° was not observed in the XRD patterns of pPD-co-DABSA 50/50 and pPD-co-2ABSA 50/50 copolymers (Figure (b), and (c)). However, pPD-co-DABSA 50/50 and pPD-co-2ABSA 50/50 copolymers were also amorphous and displayed additional small peaks at 2θ = 9°, 16° and 21° in comparison to P(pPD) polymer. These additional diffraction peaks were characteristic of the existence of –SO3H groups on the backbone chains of P(pPD) polymer (Lee et al., Citation2008).
3.4. Thermal analysis of polymers
3.4.1. TGA of the polymers
TGA was used to measure the thermal stability of P(pPD), pPD-co-DABSA and pPD-co-2ABSA copolymers in nitrogen gas atmosphere. The TGA curves of P(pPD), pPD-co-DABSA 50/50 and pPD-co-2ABSA 50/50 copolymers are shown in Figure . The P(pPD) displayed two weight loss stages, while pPD-co-DABSA and pPD-co-2ABSA copolymers showed three weight loss stages. The initial weight loss of 8–11 wt% at 100–120°C can be related to the evaporating of remaining water and/or the volatilization of solvent molecules trapped in the polymer chains (Lee et al., Citation2008). The second weight loss starting at about 180–250°C is possibly due to the cleavage of C–S bond (elimination of –SO3H groups) and the thermal degradation of polymer chains (Lee et al., Citation2008).
Figure 4. TGA curves of the polymer powders of P(pPD), pPD-co-DABSA 50/50 and pPD-co-2ABSA 50/50 in nitrogen, at a heating rate of 10°C/min.
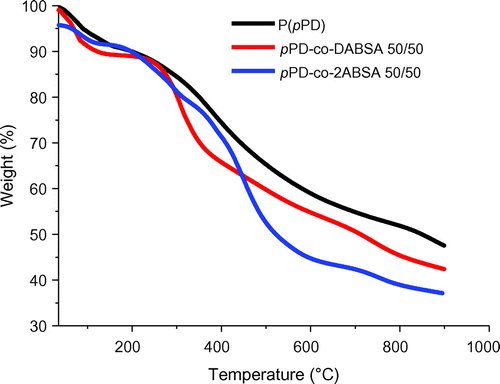
Scheme 1. The chemical reaction process of (A) the chemical oxidative polymerization reaction of pPD, (B) the copolymerization reaction of pPD with DABSA and (C) the copolymerization reaction of pPD with 2ABSA.
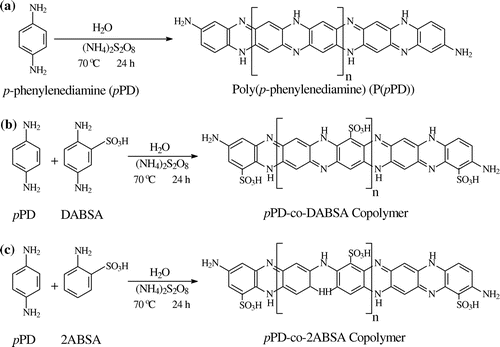
As can be seen from Figure , different thermal behaviors were observed for the P(pPD), pPD-co-DABSA and pPD-co-2ABSA copolymers, which is due to their difference in molecular structures. TGA results showed that P(pPD), pPD-co-DABSA and pPD-co-2ABSA copolymers have good heat resistance and thermal stability due to their rigid chain structure. However, no thermal degradation temperature below 180°C was observed for any of the P(pPD), pPD-co-DABSA and pPD-co-2ABSA copolymers, which means that the thermal stabilities of the copolymers are appropriate for application by the engineering industry, such as in the electrical field where higher temperature insulation is required, and in structural components in advanced high speed aircraft, weapon systems, energy storage applications, space vehicles and fuel cells even at high sulfonation levels (Rikukawa & Sanui, Citation2000).
4. Conclusion
pPD-co-DABSA and pPD-co-2ABSA copolymers with a degree of sulfonation of up to 19% were synthesized by chemical oxidative copolymerization of pPD with DABSA and 2ABSA monomers. In general, the pPD-co-DABSA and pPD-co-2ABSA copolymers exhibited a lower yield and molecular weight, but better solubility compared to the P(pPD) polymer. This indicates that the solubility of P(pPD) polymers can be improved by attaching sulfonic groups on the benzenoid rings of the P(pPD) polymers. The decrease in the molecular weight and yield of the pPD-co-DABSA and pPD-co-2ABSA copolymers is due to the steric effects and electron-withdrawing of sulfonic groups which cause the formation of cation radicals of DABSA and 2ABSA molecules difficult during the oxidative polymerization. Therefore, the steric effects and electron-withdrawing of sulfonic groups have a great impact on the structural features of pPD-co-DABSA and pPD-co-2ABSA copolymers and, consequently, on their properties. TGA showed that the P(pPD), pPD-co-DABSA and pPD-co-2ABSA copolymers displayed good thermal stability, but showed differences in their thermal behaviour due to their different molecular structures.
Funding
The authors received no direct funding for this research.
Acknowledgements
The authors wish to thank (1) University of South Africa for awarding a research fellowship to Ismael Amer, (2) Dr Damian Onwudiwe for TGA measurements, (3) Mr Andre Joubert for NMR measurements and (4) Mrs Sonja Brandt for English Editing.
Additional information
Notes on contributors
Ismael Amer
Ismael Amer received a PhD Degree in Polymer Science in 2011 from Stellenbosch University, South Africa. He is working as a senior lecturer and researcher at the Department of Chemical Engineering, University of South Africa. He has published more than 15 journal articles, conference proceedings and book chapters. He has given many national and international conference presentations for his research work in many countries. His research interest includes the synthesis and characterization of different types of polymers such as polyolefin and various kinds of conductive polymers. He is also interested in the field of fuel cell research, catalysis and polymeric membranes.
References
- Benavente, J., Garcı́a, J. M., Riley, R., Lozano, A. E., & de Abajo, J. (2000). Sulfonated poly(ether ether sulfones): Characterization and study of dielectrical properties by impedance spectroscopy. Journal of Membrane Science, 175, 43–52.10.1016/S0376-7388(00)00395-1
- Cataldo, F. (1996). On the polymerization of p-phenylenediamine. European Polymer Journal, 32, 43–50.10.1016/0014-3057(95)00118-2
- Drioli, E., Regina, A., Casciola, M., Oliveti, A., Trotta, F., & Massari, T. (2004). Sulfonated PEEK-WC membranes for possible fuel cell applications. Journal of Membrane Science, 228, 139–148.10.1016/j.memsci.2003.07.023
- Genies, C., Mercier, R., Sillion, B., Cornet, N., Gebel, G., & Pineri, M. (2001). Soluble sulfonated naphthalenic polyimides as materials for proton exchange membranes. Polymer, 42, 359–373.10.1016/S0032-3861(00)00384-0
- Gil, M., Ji, X., Li, X., Na, H., Hampsey, J. E., & Lu, Y. (2004). Direct synthesis of sulfonated aromatic poly(ether ether ketone) proton exchange membranes for fuel cell applications. Journal of Membrane Science, 234, 75–81.10.1016/j.memsci.2003.12.021
- Huang, M.-R., Ding, Y.-B., Li, X.-G., Liu, Y.-J., Xi, K., Gao, C.-L., & Kumar, R. V. (2014). Synthesis of semiconducting polymer microparticles as solid ionophore with abundant complexing sites for long-life Pb(II) sensors. ACS Applied Materials & Interfaces, 6, 22096–22107.10.1021/am505463f
- Huang, M.-R., Lu, H.-J., & Li, X.-G. (2012). Synthesis and strong heavy-metal ion sorption of copolymer microparticles from phenylenediamine and its sulfonate. Journal of Materials Chemistry, 22, 17685–17699.10.1039/c2jm32361c
- Lee, R.-H., Lai, H.-H., Wang, J.-J., Jeng, R.-J., & Lin, J.-J. (2008). Self-doping effects on the morphology, electrochemical and conductivity properties of self-assembled polyanilines. Thin Solid Films, 517, 500–505.10.1016/j.tsf.2008.06.079
- Li, X.-G., Huang, M.-R., & Yang, Y.-L. (2001). Synthesis and characterization of o-phenylenediamine and xylidine copolymers. Polymer, 42, 4099–4107.10.1016/S0032-3861(00)00661-3
- Li, X.-G., Huang, M.-R., Chen, R.-F., Jin, Y., & Yang, Y.-L. (2001). Preparation and characterization of poly(p-phenylenediamine-co-xylidine). Journal of Applied Polymer Science, 81, 3107–3116.10.1002/(ISSN)1097-4628
- Li, X.-G., Huang, M.-R., Duan, W., & Yang, Y.-L. (2002). Novel multifunctional polymers from aromatic diamines by oxidative polymerizations. Chemical Reviews, 102, 2925–3030.10.1021/cr010423z
- Mav, I., Zigon, M., Sebenik, A., & Vohlidal, J. (2000). Sulfonated polyanilines prepared by copolymerization of 3-aminobenzenesulfonic acid and aniline: The effect of reaction conditions on polymer properties. Journal of Polymer Science Part A: Polymer Chemistry, 38, 3390–3398.10.1002/(ISSN)1099-0518
- Mav-Golež, I., Pahovnik, D., Bláha, M., Žigon, M., & Vohlídal, J. (2011). Copolymers of 2-methoxyaniline with 2- and 3-aminobenzenesulfonic and 2- and 3-aminobenzoic acids: Relationships between the polymerization conditions, structure, spectroscopic characteristics and conductivity. Synthetic Metals, 161, 1845–1855.10.1016/j.synthmet.2011.06.023
- Nolte, R., Ledjeff, K., Bauer, M., & Mülhaupt, R. (1993). Partially sulfonated poly(arylene ether sulfone) – A versatile proton conducting membrane material for modern energy conversion technologies. Journal of Membrane Science, 83, 211–220.10.1016/0376-7388(93)85268-2
- Pradeep, S., Arunraj, L., & Dhatchinamurthy, L. (2014). Synthesis and characterization of poly m-amino benzene sulfonic acid for sensor applications. Journal of NanoScience and NanoTechnology, 2, 390–393.
- Qi, Z., & Pickup, P. G. (1998). High performance conducting polymer supported oxygen reduction catalysts. Chemical Communications, 21, 2299–2300.10.1039/a805322g
- Qi, Z., Lefebvre, M., & Pickup, P. G. (1998). Electron and proton transport in gas diffusion electrodes containing electronically conductive proton-exchange polymers. Journal of Electroanalytical Chemistry, 459, 9–14.10.1016/S0022-0728(98)00241-1
- Ramkumar, R., & Minakshi, M. (2015). Fabrication of ultrathin CoMoO4 nanosheets modified with chitosan and their improved performance in energy storage device. Dalton Transactions, 44, 6158–6168.10.1039/C5DT00622H
- Ramkumar, R., & Sundaram, M. M. (2016). A biopolymer gel-decorated cobalt molybdate nanowafer: Effective graft polymer cross-linked with an organic acid for better energy storage. New Journal of Chemistry, 40, 2863–2877.10.1039/C5NJ02799C
- Rikukawa, M., & Sanui, K. (2000). Proton-conducting polymer electrolyte membranes based on hydrocarbon polymers. Progress in Polymer Science, 25, 1463–1502.10.1016/S0079-6700(00)00032-0
- Tsuchida, E., Kaneko, M., & Kurimura, Y. (1970). Oxidative polymerization of aromatic amines in aqueous solution of iron chelate. Macromolecular Chemistry and Physics, 132, 209–213.10.1002/macp.1970.021320119