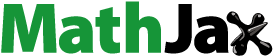
Abstract
This study aimed at the degradation of COD, BOD and Color present in palm oil mill effluent (POME) using UV/TiO2 system. A Statistical tool called face-centered central composite design has been run through response surface methodology by the use of design expert software to model and optimize the photodegradation process. The SEM average size of the synthesized TiO2 particle is 100 nm and this confirm that it is anatase. The prominent peak as reveal by XRD occur at 22.3° and this indicates that TiO2 formed is anatase. The FTIR results shows a peak value of 1,406.50 and 1,452.50 cm−1 indicating that the TiO2 anatase is formed while nonappearance of the peak at 2,900 cm−1 indicates that the all organic compounds have been removed after calcination. The three models developed for the three pollutants are non-significant as revealed from the Prob > F values of 0.0584 for COD, 0.0612 for BOD and 0.4239 for color and this confirms that proposed models fit the experimental data. The optimum conditions for the three parameters are 5.50 for initial pH, 4.84 mg for catalyst dosage and 42.86 min for time and the percentage removal of the COD, BOD, and color are within the range of 59.43–96.81%, 48.05–102.68% and 60.63–94.29% respectively. Regression analysis obtained through analysis of variance provided significant quadratic polynomial with the coefficient of determination R2 = 0.9646, 0.9496 and 0.9223 for COD, BOD, and Color removal respectively.
Public Interest Statement
Palm oil mill effluent (POME) is the wastewater generated when palm fruit is processed for oil production. This wastewater, when discharge into nearby rivers affects the survival of living organisms. Biological treatment, coagulation-flocculation, and membrane technology are often used for the POME treatment. These methods though effective are very expensive and usually generate secondary waste know as sludge. In addition, they do not remove organic pollutants present in POME.
This research aimed at using the energy of ultraviolet light in the presence titanium dioxide catalyst to completely degrade pre-treated by palm oil mill effluent through removal of organic pollutants such as chemical oxygen demand, biological oxygen demand, and color. This method is simple and can be operated under ambient conditions. The percentage degradation of these pollutants has been found to be above 90%. This study has also reveals that this technique can be upgraded for large scale treatment.
1. Introduction
Palm oil mill effluent (POME) contributes to serious environmental problems especially to aquatic life when disposed of nearby water bodies, and is thus often treated using anaerobic, aerobic bioreactors and membrane technology (Chong, Sen, Kayaalp, & Ang, Citation2012; Wang et al., Citation2015; Yoochatchaval et al., Citation2011). These schemes are ineffective in removing recalcitrant organic compounds present in POME (Rupani & Singh, Citation2010). They also produce a large volume of sludge which contributes to land pollution in addition to long retention time required for their operation (Igwe & Onyegbado, Citation2007). Similarly, membrane technology is very expensive because the membrane needs to be replaced over time and this incurs high maintenance costs (Azmi & Yunos, Citation2014).
Often than not, POME is pre-treated using coagulation-flocculation process (Alhaji et al., Citation2016, Citation2017) aimed at reducing a number of suspended solids before advanced treatment by anaerobic, aerobic or membrane technology. There is also need for further treatment to remove recalcitrant organic compound that is very difficult to remove by conventional means. Advanced oxidation processes employing heterogeneous photocatalysis and Fenton-like oxidations are promising technologies in this regard and have high potential to decompose recalcitrant organic pollutants in POME (Saeed, Azizli, Isa, & Bashir, Citation2015; Tan, Goh, Lau, & Ismail, Citation2014). This process can be operated under ambient conditions (Chong, Jin, Chow, & Saint, Citation2010) and is capable of mineralizing the organic content present in POME into environmentally friendly products.
Among the various photocatalysts (e.g. TiO2, ZnO, Fe2O3, WO3 etc.) employed in heterogeneous photocatalysis, TiO2 is the most promising because of its strong photocatalytic activity, non-toxicity, high chemical stability, low cost, high transparency to visible light (Schneider et al., Citation2014). As TiO2 is exposed to ultra-violet irradiation, it absorbs a photon of light that causes TiO2 to the excited state resulting in the formation of an electron-hole pair. The electron-hole thus formed migrate to titania surface and it further reacts with O2 and H2O to form reactive oxidation species (O2− and ·OH) (Fujishima, Rao, & Tryk, Citation2000) and these reactive species are capable of oxidizing organic molecules in contact with its surface.
Photocatalytic processes used in the treatment of organic effluents (POME inclusive) have been studied through the determination of reaction kinetics mechanisms involved in the process and the identification of various intermediates (Ng, Lee, Khan, & Cheng, Citation2016). In the kinetic studies, the most commonly used methods involve the study of one factor at a time while keeping the other factors constant with the assumption that they do not interact with each other. Examples of such factors include pH of the solution, catalyst dose, temperature, intensity of UV light, etc. Most of these factors interact independently with one another during the photocatalytic process, it is therefore very difficult to optimize the responses such as organic COD removal, BOD removal, or color removal when such method is used. Consequently, this approach is cumbersome and costly as more time and reagent are required leading to its overall inefficiency (Ahmadi, Vahabzadeh, Bonakdarpour, Mofarrah, & Mehranian, Citation2005). Therefore, this method has currently been replaced with an efficient method, which involves an interactive combination of independent variables, called response surface methodology (RSM). It gives the desired responses and effective means for optimizing various industrial processes as documented in the scientific literature (Aslan, Citation2008). Though photocatalytic technology employing titania have recently been utilized for POME treatment with emphasis mainly on photokinetics and scavenging studies (Ng et al., Citation2016), the application of RSM to the photocatalytic treatment of POME employing titanium dioxide as photocatalyst catalyst is still scarce.
Primarily, RSM is a statistical method applied for optimization of process variables using the design of experiments such as full three level factorial design, central composite design, Doehlert design, Box-Behnken design etc. RSM is normally used to predict the most efficient operations found majorly in chemical and process engineering (Ahmad, Low, Shukor, & Ismail, Citation2009). In the present study, a 23 full factorial, face-centered central composite design (FCCCD) with five center points will be used as a design basis with the aid of design expert® version 6.0.8 statistical software. A second-order response surface model obtained will be used to calculate the interaction effects of the most influential parameters namely pH, catalyst dose and reaction time on the efficient removal of COD by photocatalytic degradation of pre-treated POME using titanium particles. The regression models will also be used to predict the operating parameters for the optimum removal of COD, BOD and color removal operating parameters.
2. Material and methods
2.1. POME collection
The pre-treated POME used in this study was obtained from aerobic treatment pond of Salcra palm oil mill in Bau, state of Sarawak, Malaysia. It was collected in a 20-L plastic bottle and was kept in a refrigerator at a temperature of 4°C. The Standard method for examination of water and wastewater was followed for sample collection and storage (APHA, Citation2012). The initial characteristics of the pre-treated POME used in this study are shown in Table . The sample was first filtered using whatman® filter-UK filter paper (size 50 mm Ø) in order to reduce the total suspended solids.
Table 1. Characteristics of the pre-treated POME used
2.2. Synthesis and characterization of TiO2
Titanium dioxide particles were synthesized following the sol-gel method described by Khataee and Mansoori (Citation2012). Analytical grade titanium isopropoxide (Sigma, 99%), isopropanol (Across, 99%) and acetic acid (Sigma, 98%) have been used for the synthesis. The synthesized TiO2 particles were characterized using scanning electron microscope (JEOL JSM-6390LA), X-ray diffraction, XRD (Model D/MAX 2500, Rigaku Analytical devices Inc. U.S.A) and Fourier Transformed Infrared Spectrometer, FTIR (Thermo Scientific/Nicolet’s 10) in order to determine the surface morphology and chemical functional groups of the synthesized TiO2.
2.3. Photocatalytic degradation experiments and procedures
500 mL beaker was used as photoreactor and 400 mL POME was mixed with the desired quantity of TiO2. The initial pH of the suspension was adjusted using concentrated NaOH (0.1 mol/dm3). The UV lamp (UV Fluorescent tube 20 WATT/4 FT, USA) was placed in between a transparent water jacket (to maintain ambient temperature) (as shown in Figure ) to irradiate the POME in titanium suspension. The UV-irradiation is emitted at 250 nm wavelength. The whole content of the reactor was continuously stirred using magnetic stirrer (Lab tech. ES35 A-pro, UK) during throughout the irradiation process. COD was determined using the COD digester (Rocker COD reactor CR25, USA) with subsequent chemical titration as described in APHA standard methods for the examination of water and wastewater (APHA, Citation2012). Similarly, BOD has been determined using BOD analyzer (LH-BOD601, Thermofisher Scientific, Australia) with subsequent chemical titration using reagents as specified in the APHA standard methods for the examination of water and wastewater. The color was determined by UV Spectrophotometer (UV-1800, Shimadzu Scientific instruments, North America) with necessary calibrations as specified in the APHA standard methods for the examination of water and wastewater. The values of COD, BOD and Color that were obtained are presented as the average of three readings while the % efficiency of their removal is calculated using Equation 1:(1)
(1)
where Ci s the initial COD, BOD or Color in mg/l, the final concentration of COD, BOD, or Color in mg/l.
For each experiments, the chosen independent variables (Initial pH, Catalyst dosage and reaction time) were varied following standard practices reported in the past literature. For example, the initial pH used in this study has been chosen based on the work of Ng et al. (Citation2017), in which it has been confirmed that acidic medium with pH between 3 and 5 is the most suitable condition for photocatalytic processes involving POME. Similarly, preliminary experiments thus conducted have revealed that reaction time of 60 min is sufficient to allow for the photodegradation process to proceed to completion and no further degradation has been observed after 60 min while a very low degradation rate was observed at 20 min. Also, the catalyst loading of 6 mg is just sufficient to allow for highest degradation and it has been observed that any further increase in this value only increases the cloudiness of the effluent stream, which obstructs the passage of light thus leading to slower photodegradation process. Therefore, 2–6 mg of catalyst dosage has been chosen for this study. All the selected values for each of the experiments are shown in Tables and .
Table 2. Experimental range and levels of independent variables
Table 3. Full factorial central composite face-centered design (FCCCD) matrix for the three variables (coded and actual) and their units
2.4. Design of photocatalytic experiments and statistical analysis
The experimental design for this study has been built on three independent variables: pH (X1), catalyst dosage (X2), and Reaction time (X3). The ranges of the independent variables were selected through experiments obtained from open literature as described earlier. 23 full factorial FCCCD, employing design expert 6.0.8 software (Stat-Ease Inc., Minneapolis, USA), was used for the design, modeling, and optimization. The experiments were performed at ambient conditions (atmospheric pressure, room temperature of 25°C) while the agitation speed was kept constant. The three independent variables are converted to dimensionless code numbers using Equation 2 and presented in Table with their ranges and level of experimental design as used in the present study. It must be noted that the arrangement of the FCCCD (Table ) is configured to allow for the development of experimental (empirical) equations containing second order polynomial as reported in the literature (Bailey & Borwein, Citation2009). The efficiency of COD, BOD and Color removal are the required responses or the dependent variable.(2)
(2)
where xi, s the dimensionless coded value, XO is the value of Xi Centre point, ΔX is the step change (Khataee, Citation2010). The minimum, center and maximum levels (−1, 0 and + 1) of each variable are designated according to the FCCCD principle, which involves design of experiments and it thus also allows the building of a second order quadratic model for the response variable without the need to use a complete three-level factorial experiment (Khuri & Mukhopadhyay, Citation2010). The dimensionless numbers in the quadratic model allow comparison of variables of different natures, so as to adjust lack of fit, and to decrease the error in the polynomial quadratic statistical analysis. Coded variables are often used when constructing this design.
In this study, the FCCCD consists of 8 (23) full factorial points, 6 (2 × 3) axial points, and five replications at the center points. In whole, 19 experiments (8 + 6 + 5 = 19) were therefore designed. The response Equation (3) was used to correlate the dependent and independent variables. The equation represents the behavior of the system as an experimental second-order quadratic model.(3)
(3)
where, Y is the removal efficiency of COD, BOD and Color as a response variables, b0 is error b1, b2, and b3 are the coefficients for linear effects (also known as main effects describe the variation of the quadratic model due to one single factor) b11, b22, and b33 are quadratic coefficients while b12, b13, and b23 are the coefficients for interaction effects described by more than one factor (Oehlert, Citation2010). ANOVA as offered from design expert software has been used to interpret the complex relationship among the three independent variables and the response variable of the complete data-set-up. It is important to note that only two interactions effects (i.e. only interaction between two independent variables at one time) have been considered in this work because it is simple and easy to handle with reasonable predictions (Yuksel, Kanik, & Baykara, Citation2000). Similarly, that the interaction effects, center point, factor, coded value, significant interactions, and response are found out only by the use of statistical experimental design techniques (Fegade et al., Citation2013).
3. Results and discussion
3.1. Characterization of the synthesized TiO2
3.1.1. XRD analysis
The XRD analysis of titanium nanoparticles synthesized has been carried out at room temperature using Rigaku X-ray Diffraction D/MAX 2500, with Cu KR radiation (λ = 1.5406 Å) at a scan rate of 0.04°/0.15 s. An accelerating voltage of 40 kV and an emission current of 50 mA were used. It can be observed from Figure that the prominent peak in XRD occurs at 2θ (22.3°) and other well-defined peaks are obtained at 38, 48, and 54°. These peaks indicate anatase form of TiO2 and these values are similar to the ones obtained in the works of Liu et al. (Citation2003) and Khataee, Fathinia, and Aber (Citation2010).
3.1.2. SEM analysis
SEM image of the synthesized TiO2 particles prepared at a calcination temperature of 200°C for 3 h is shown in Figure . It can be seen from the image that the average size of the titanium nanoparticles is less than 100 nm, which further proves that the synthesized titanium nanoparticles are anatase. This assertion is as reported in previous studies (Hanaor & Sorrell, Citation2011; Reyes-Coronado et al., Citation2008).
3.1.3. FTIR analysis
Figure shows the FTIR spectra of a Titanium particles samples synthesized via the sol-gel method in the range of 1,000–4,000 cm−1. Peaks at 1,406.50 and 1,452.50 cm−1 are for O–Ti–O bonding in anatase morphology (Mertens et al., Citation2012). There is no peak at 2,900 cm−1 regarding C–H stretching band, which means all organic compounds were removed from the samples after calcinations.
3.2. Modelling and optimization of photocatalytic treatment of POME
The experimental and predicted values of all the responses for removal efficiency of COD, Y1, BOD, Y2 and Color Y3 are presented in Figure . The experimental measurements thus obtained were used to estimate the regression coefficients and to develop the regression quadratic model’s equations (Equations (4)–(6)) for the three responses and they all consist of linear, quadratic and interaction effects.(4)
(4)
(5)
(5)
(6)
(6)
where Y1, Y2 and Y3 are the responses while X1, X2 and X3 are the coded values of initial pH, catalyst dosage and reaction time respectively.
The statistical importance of the mean square ratio variation owing to regression and residual errors of the mean square were evaluated using the analysis of variance (ANOVA). As shown in Table , the three models were established within 95% confidence level and it has been reported in the literature that model “Lack of Fit” with ‘‘Prob. > F” > 0.05 is non-significant and the proposed model fit the experimental data (Cramer et al., Citation2016; McHugh, Citation2011). For this study, ‘‘Prob. > F’’ for three responses are 0.0584, 0.0612 and 0.4239 (Table ) therefore, the proposed models fit the experimental data and the independent variables have significant effects on all the three the output responses. Similarly, the value of adjusted R2 for the three responses are 0.9292 for COD, 0.9462 for BOD and 0.9206 for Color which are very close to the coefficient of determination, R2 are 0.9646, 0.9496 and 0.9223 respectively and this thus indicates that the reliability of experimental data used to estimate the COD, BOD and color removal. Adequate precision is used to measure the signal to noise ratio and a ratio greater than 4 has been proved to be desirable (Welvaert & Rosseel, Citation2013). For this study, a ratio of 14.601, 11.24 and 7.090 (Table ) have been obtained for COD, BOD and Color respectively and this thus indicates adequate signal strength for the models to navigate the design space.
Table 4. Statistical parameters obtained from ANOVA of quadratic models for photocatalytic removal of COD, BOD and Color from pre-treated POME
Figure (a)–(c) show the predicted values due to the model response for removal of COD, BOD and Color and they have been correlated with the experimental (actual) values. The data points for each of the response are reasonably distributed close to each other in a linear behavior. Thus, the plots indicate sufficient agreement between predicted and experimental data. Similarly, Figure (a)–(c) show the methods of least square being used to obtain residuals plots to judge the adequacy of the model for COD, BOD and Color removal. The normal plots of the residual plots and the relationship between the normal percentage probabilities vs. studentized residual formed show that the residual distributions are satisfactorily expressed by the normal distribution as clearly specified for the independent variables. In addition, the residual plots show linear behavior as such a straight line is obtained which clearly indicates that the predictions made by the proposed models are accurate.
Figure 6. Normal plot distribution of the residuals for (a) COD removal; (b) BOD removal; (c) Color removal.
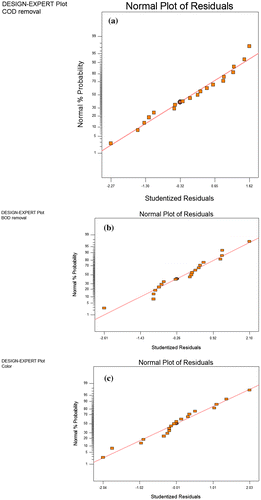
3.2.1. 3D response surface plots
Assessment of response surfaces (3D response surface plot) is a useful aspect of experimental design. The model equation obtained is usually used to generate a 3D response surface plots that offer a diverse and more useful visible outlook of the interaction effects (Montgomery, Citation2004). Therefore, the plots show a more useful pictorial view of the surface profile and are subsequently used to determine the optimum in a more precise and accurate manner. The interactions among the three independent variables and the response are illustrated based on the regression model equations (Equations (4)–(6)) and the relevant 3D surface plots are carried out with different relationships among the independent variables and different peaks and curves representing the response.
The 3D surface curvatures as shown in Figure (a)–(c) have been chosen for the axes of the response based on the variable that gives the quadratic and interaction terms with the largest absolute coefficients in the fitted models, this allow the curvatures to be accounted for easily. Initial pH and catalyst dosage were thus selected for RSM plots for removal of COD, BOD and Color while the reaction time was kept at the central level of 40 min (0.00 in coded unit). It shows that the highest removal of COD, BOD and Color occurs at the highest value of the initial pH and catalyst dosage. Therefore, initial pH and catalyst dosage has a significant effect on the removal of this effluent parameters. The shape of the 3D response surface plots shows a very strong curvature even as the there is significant interactions within the range of 4–6 mg catalyst dosage and Initial pH in the range of X1 = 4–5.50, imply that the acidic medium is the best for the removal of these effluents parameters from pre-treated POME. This assertion is in agreement with a previous study for COD removal from POME through Fenton oxidation process (Saeed et al., Citation2015).
Figure 7. 3D surface plot showing (a) COD removal (Y1) for the POME treatment by photodegradation. Dependence of Y1 on the initial pH (X1) and catalyst dosage (X2) (Reaction time, X3 = 0). (b) BOD removal (Y2) for the POME treatment by photodegradation. Dependence of Y2 on the initial pH (X1) and catalyst dosage (X2) (Reaction time, X3 = 0). (c) Color removal (Y3) for the POME treatment by photodegradation. Dependence of Y3 on the initial pH (X1) and catalyst dosage (X2) (Reaction time, X3 = 0).
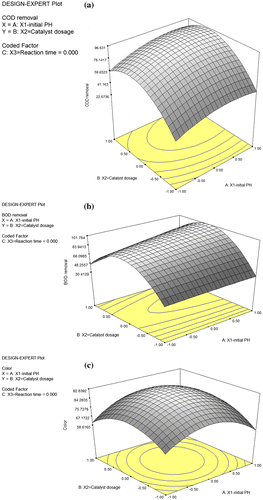
Similarly, as shown in Figure (a)–(c) the percentage removal of COD, BOD and Color are at highest level of 96.631, 101.784 and 92.839% respectively. It can be observed that the removal COD and BOD (above 100%) record the highest percentage, this can be attributed to the fact that the two of the three selected parameters (i.e. initial pH and catalyst dosage) contributes increasingly towards the mineralization of organic components present in the POME whereas removal of Color record low percentage because some degraded species within POME re-appear as electron scavengers which hinders the effective removal of color during the photodegradation process.
3.2.3. Numerical optimization analysis
Numerical optimization analysis has been performed to determine the optimum process parameters at the maximum removal of COD, BOD, and Color from pre-treated POME. Under the selected desirability conditions of between 0 and 1 as set in the design expert software, the removal efficiency of COD, BOD and Color falls within the range of 59.43–96.81%, 48.05–102.68% and 60.63–94.29% (Figure (a)–(c)), respectively as validated by the experiments when initial pH (X1) is 5.50, catalyst dosage X2, is 4.84 and the reaction time X3 is set as 42.86 min (0.143 in coded unit). It is important to note that the selection of desirability between 0 and 1 is not a condition that must be adhered to at all time because it depend largely on how close the lower and upper limit have been set in relation to the actual optimum value. In the overall, it can be noticed that there are only small differences between simulated values and experimental results and this thus confirms that RSM employing FCCCD is a useful tool to obtain good and reliable operating conditions for photocatalytic removal of COD, BOD and Color from pre-treated POME using titanium particles in a slurry photoreactor at laboratory scale.
Figure 8. 3D surface plot showing Numerical optimization of the (a) COD removal (Y1) for the POME treatment by photodegradation. Dependence of Y1 on the initial pH (X1) and catalyst dosage (X2) (Reaction time, X3 = 0.143) (b) BOD removal Y2for the POME treatment by photodegradation. Dependence of Y2 on the initial pH (X1) and catalyst dosage (X2) (Reaction time, X3 = 0.143) (c) Color removal Y3 for the POME treatment by photodegradation. Dependence of Y3 on the initial pH (X1) and catalyst dosage (X2) (Reaction time, X3 = 0.143).
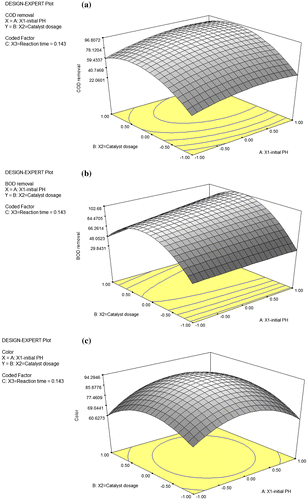
4. Conclusion
An experimental approach, through FCCCD/RSM offered by design expert software, has been exercised to optimize process parameters for photocatalytic degradation of pre-treated POME using synthesized titanium nanoparticles. The coefficient of determination otherwise known as regression model (R2) as obtained from the ANOVA and have been examined under three process parameters (i.e. initial pH, catalyst dosage and reaction time) for the three responses whose values are 0.9646, 0.9496 and 0.9223 for the removal of COD, BOD and Color respectively. These values are high and it shows that the data predicted by all the three models are in agreement with the experimental data and all values of responses attained in this study after photodegradation are within acceptable discharge limit for POME. The present optimum data obtained for the three process parameters have been aligned with the highest possible removal of COD, BOD and Color that can be set as benchmark values for the effective prototype design of photocatalytic reactor for POME treatment.
List of abbreviations | ||
ANOVA | = | Analysis of Variance |
APHA | = | American Public Health Association |
BOD | = | Biochemical Oxygen Demand |
COD | = | Chemical Oxygen Demand |
DOE | = | Design of Experiments |
FCCCD | = | Face-Centered Central Composite Design |
FTIR | = | Fourier Transform Infrared |
POME | = | Palm Oil Mill Effluent |
RSM | = | Response Surface Methodology |
SEM | = | Scanning Electron Microscope |
USA | = | United State of America |
Funding
This work was supported by Universiti Malaysia Sarawak for the financial support with [grant number F02/SpGS/1407/16/8/2016] offered to the present project.
OAEN_1382980_Supplementary_Materials.docx
Download MS Word (14.6 KB)Acknowledgement
The authors will like to acknowledge Universiti Malaysia Sarawak for the financial support with grant number: F02/SpGS/1407/16/8/2016 offered to the present project.
Additional information
Notes on contributors
Mohammed Haji Alhaji
Mohammed Haji Alhaji is a PhD candidate in the Department of Chemical Engineering and Energy Sustainability Universiti Malaysia Sarawak. His research interest is in development of photocatalytic technology for palm oil mill effluent treatment.
Khairuddin Sanaullah
Khairuddin Sanaullah is an associate professor in the Department of Chemical Engineering and Energy Sustainability Universiti Malaysia Sarawak. His research interest includes multiphase systems and transport processes.
Soh Fong Lim
Soh Fong Lim is a senior lecturer in the Department of Chemical Engineering and Energy Sustainability, Universiti Malaysia Sarawak. Her research interests include Environmental Chemical Engineering.
Andrew Ragai Henry Rigit
Andrew Ragai Henry Rigit is a professor in the Department of Mechanical and Manufacturing Engineering, Universiti Malaysia Sarawak.
Abdulhamid Hamza
Abdulhamid Hamza is a senior lecturer in the Department of Chemical Engineering, Ahmadu Bello University Zaria, Nigeria. His research interest includes photocatalysis for Environmental Application.
Afrasyab Khan
Afrasyab Khan is Research Fellow and post-doctorate student in the Department of Chemical Engineering and Energy Sustainability Universiti Malaysia Sarawak.
References
- Ahmad, A. L., Low, S. C., Shukor, S. R., & Ismail, A. (2009). Optimization of membrane performance by thermal-mechanical stretching process using responses surface methodology (RSM). Separation and Purification Technology, 66, 177–186.10.1016/j.seppur.2008.11.007
- Ahmadi, M., Vahabzadeh, F., Bonakdarpour, B., Mofarrah, E., & Mehranian, M. (2005). Application of the central composite design and response surface methodology to the advanced treatment of olive oil processing wastewater using Fenton’s peroxidation. Journal of Hazardous Materials, 123, 187–195.10.1016/j.jhazmat.2005.03.042
- Alhaji, M. H., Sanaullah, K., Lim, S.-F., Khan, A., Hipolito, C. N., Abdullah, M. O., … Jamil, T. (2016). Photocatalytic treatment technology for palm oil mill effluent (POME)—A review. Process Safety and Environmental Protection, 102, 673–686.10.1016/j.psep.2016.05.020
- Alhaji, M. H., Sanaullah, K., Khan, A., Hamza, A., Muhammad, A., Ishola, M. S., & Rigit, A. R. H. (2017). Recent developments in immobilizing titanium dioxide on supports for degradation of organic pollutants in waste water: A review. International Journal of Environmental Science and Technology, 9, 2039–2052.10.1007/s13762-017-1349-4
- APHA. (2012). Standard methods for the examination of water and wastewater (pp. 1–541). American Water Works Association, Water Environment Federation.
- Aslan, N. (2008). Application of response surface methodology and central composite rotatable design for modeling and optimization of a multi-gravity separator for chromite concentration. Powder Technology, 185, 80–86.10.1016/j.powtec.2007.10.002
- Azmi, N. S., & Yunos, K. F. M. (2014). Wastewater treatment of palm oil mill effluent (POME) by ultrafiltration membrane separation technique coupled with adsorption treatment as pre-treatment. Agriculture and Agricultural Science Procedia, 2, 257–264.10.1016/j.aaspro.2014.11.037
- Bailey, D. H., & Borwein, J. M. (2009). Experimental mathematics and computational statistics. Wiley Interdisciplinary Reviews: Computational Statistics, 1, 12–24.10.1002/wics.1
- Chong, M. N., Jin, B., Chow, C. W. K., & Saint, C. (2010). Recent developments in photocatalytic water treatment technology: A review. Water Research, 44, 2997–3027.10.1016/j.watres.2010.02.039
- Chong, S., Sen, T. K., Kayaalp, A., & Ang, H. M. (2012). The performance enhancements of upflow anaerobic sludge blanket (UASB) reactors for domestic sludge treatment–A State-of-the-art review. Water Research, 46, 3434–3470.10.1016/j.watres.2012.03.066
- Cramer, A. O. J., van Ravenzwaaij, D., Matzke, D., Steingroever, H., Wetzels, R., Grasman, R. P. P. P., … Wagenmakers, E.-J. (2016). Hidden Multiplicity in Multiway ANOVA: Prevalence, Consequences, and Remedies. Psychonomic Bulletin & Review, 23(2), 640–647.
- Fegade, S. L., Tande, B. M., Cho, H., Seames, W. S., Sakodynskaya, I., Muggli, D. S., & Kozliak, E. I. (2013). Aromatization of propylene over Hzsm-5: A design of experiments (DOE) approach. Chemical Engineering Communications, 200, 1039–1056.10.1080/00986445.2012.737385
- Fujishima, A., Rao, T. N., & Tryk, D. A. (2000). Titanium dioxide photocatalysis. Journal of Photochemistry and Photobiology C: Photochemistry Reviews, 1(1), 1–21.10.1016/S1389-5567(00)00002-2
- Hanaor, D. A. H., & Sorrell, C. C. (2011). Review of the anatase to rutile phase transformation. Journal of Materials Science, 46, 855–874.10.1007/s10853-010-5113-0
- Igwe, J. C., & Onyegbado, C. C. (2007). A review of palm oil mill effluent (Pome) water treatment. Global Journal of Environmental Research, 1, 54–62.
- Khataee, A. R. (2010). Optimization of UV-promoted peroxydisulphate oxidation of C.I. Basic Blue 3 using response surface methodology. Environmental Technology, 31, 73–86.10.1080/09593330903358302
- Khataee, A., & Mansoori, G. A. (2012). Nanostructured titanium dioxide materials: Properties, preparation and applications. World Scientific Publishers, 1–204.
- Khataee, A. R., Fathinia, M., & Aber, S. (2010). Kinetic modeling of liquid phase photocatalysis on supported TiO2 nanoparticles in a rectangular flat-plate photoreactor. Industrial and Engineering Chemistry Research, 49, 12358–12364.10.1021/ie101997u
- Khuri, A. I., & Mukhopadhyay, S. (2010). Response surface methodology. Wiley Interdisciplinary Reviews: Computational Statistics, 2, 128–149.10.1002/wics.v2:2
- Liu, H., Yang, W., Ma, Y., Cao, Y., Yao, J., Zhang, J., & Hu, T. (2003). Synthesis and characterization of titania prepared by using a photoassisted sol−gel method. Langmuir, 19, 3001–3005.10.1021/la026600o
- McHugh, M. L. (2011). Multiple comparison analysis testing in ANOVA. Biochemia Medica, 21, 203–209.10.11613/issn.1846-7482
- Mertens, T., Gammel, F. J., Kolb, M., Rohr, O., Kotte, L., Tschöcke, S., & Kaskel, S. (2012). Investigation of surface pre-treatments for the structural bonding of titanium. International Journal of Adhesion and Adhesives, 34, 46–54.10.1016/j.ijadhadh.2011.12.007
- Montgomery, D. (2004). Design and analysis of experiments (5th ed.). New York, NY: Wiley.
- Ng, K. H., Lee, C. H., Khan, M. R., & Cheng, C. K. (2016). Photocatalytic degradation of recalcitrant POME waste by using silver doped titania: Photokinetics and scavenging studies. Chemical Engineering Journal, 286, 282–290.10.1016/j.cej.2015.10.072
- Ng, K. H., Khan, M. R., Ng, Y. H., Hossain, S. S., & Cheng, C. K. (2017). Restoration of liquid effluent from oil palm agroindustry in Malaysia using UV/TiO2 and UV/ZnO Photocatalytic systems: A comparative study. Journal of Environmental Management, 196, 674–680.10.1016/j.jenvman.2017.03.078
- Oehlert, G. W. (2010). A first course in design and analysis of experiments (pp. 1–679). University of Minnesota USA.
- Reyes-Coronado, D., Rodríguez-Gattorno, G., Espinosa-Pesqueira, M. E., Cab, C., de Coss, R., & Oskam, G. (2008). Phase-pure TiO2 nanoparticles: Anatase, brookite and rutile. Nanotechnology, 19(14), 145605.10.1088/0957-4484/19/14/145605
- Rupani, P., & Singh, R. (2010). Review of current palm oil mill effluent (POME) treatment methods: Vermicomposting as a sustainable practice. World Applied Sciences, 11, 70–81.
- Saeed, M. O., Azizli, K., Isa, M. H., & Bashir, M. J. K. (2015). Application of CCD in RSM to obtain optimize treatment of POME using Fenton oxidation process. Journal of Water Process Engineering, 8, e7–e16.10.1016/j.jwpe.2014.11.001
- Schneider, J., Matsuoka, M., Takeuchi, M., Zhang, J., Horiuchi, Y., Anpo, M., Bahnemann, D. W. (2014). Understanding TiO2 Photocatalysis: Mechanisms and Materials. Chemical Reviews, 114, 9919–9986.10.1021/cr5001892
- Tan, Y. H., Goh, P., Lau, W. J., & Ismail, A. F. (2014). Treatment of aerobic treated palm oil mill effluent (AT-POME) by using TiO2 photocatalytic process. Journal Teknologi, 70(2), 61–63.
- Wang, J., Mahmood, Q., Qiu, J.-P., Li, Y.-S., Chang, Y.-S., & Li, X.-D. (2015). Anaerobic treatment of palm oil mill effluent in pilot-scale anaerobic EGSB reactor. BioMed Research International, 2015, 7.
- Welvaert, M., & Rosseel, Y. (2013). On the definition of signal-to-noise ratio and contrast-to-noise ratio for fMRI data. PLoS ONE, 8(11), e77089.
- Yoochatchaval, W., Kumakura, S., Tanikawa, D., Yamaguchi, T., Yunus, M. F. M., Chen, S. S., … Kubota, S. (2011). Anaerobic degradation of palm oil mill effluent (POME). Water Science and Technology, 64, 2001–2008.10.2166/wst.2011.782
- Yuksel, N., Kanik, A. E., & Baykara, T. (2000). Comparison of in vitro dissolution profiles by ANOVA-based, model-dependent and -independent methods. International Journal of Pharmaceutics, 209, 57–67.10.1016/S0378-5173(00)00554-8