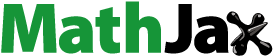
Abstract
The corrosion inhibition effect of non-toxic calcium gluconate at 0.75%, 1.25%, 1.75% and 2.25% concentration on mild steel in artificial seawater (3.5% NaCl) was studied by potentiodynamic polarization, coupon measurement, optical microscopy characterization and open circuit potential measurement. Results obtained showed the inhibiting compound effectively suppressed the corrosion of mild steel with inhibition efficiency value generally above 80% from polarization test and 90% from coupon measurement after 0.75% concentration of calcium gluconate. Calcium gluconate exhibited mixed type inhibition property with dominant anodic inhibition effect. The inhibitor adsorbed onto the steel surface through physisorption mechanism according to Langmuir and Frumkin isotherm models. Calcium gluconate shifted open circuit corrosion potential plot of mild steel to electropositive values compared to the plot of the non-inhibited steel. Corrosion pits in addition to severe worn out morphology on the image of the non-inhibited steel were absent on the inhibited steel with relatively improved surface morphology.
Keywords:
PUBLIC INTEREST STATEMENTS
The economic impact and problems resulting from corrosion has drawn strong attention from scientists and engineers worldwide. Corrosion of mild steel in industrial environments is a major concern in chemical processing plants, oil and gas industry, manufacturing, automobile industry, marine operations, boiler plants and power generation plants due to the considerable cost involved in the replacement of metallic parts in their various applications. The consequence often leads to plant shutdowns, breakdown of industrial equipment, reduced efficiency, industrial downtime, high maintenance cost due to replacement of damaged part, wastage of valuable resources and expensive overdesign. Corrosion inhibition is of great practical importance, being extensively employed in curtailing wastage of engineering materials and minimizing costs of corrosion control. A great number of studies have being devoted to the subject of corrosion inhibitors. Identification of the functional groups in organic compounds responsible for corrosion inhibition is important in the development of organic corrosion inhibitors of mild steels.
1. Introduction
Corrosion of metallic structures has been a major challenge in architectural design, ancient artifacts petrochemical, automobile, shipping, mining and energy generation industries, causing loss of revenue due to repair, replacement of damaged parts and maintenance overheads (Lorenzo, Rizzo, Formisano, Landolfo, & Guastaferro, Citation2019; Loto, Citation2016; Loto & Babalola, Citation2017; Rizzo, Di Lorenzo, Formisano, & Landolfo, Citation2019). Carbon steels are the most commonly used materials as metallic structures for industrial applications due to their relatively low cost, easy fabrication and excellent mechanical and physical properties (Loto & Loto, Citation2018). Despite these advantages, carbon steel corrosion limits their versatility and application (Ahamad, Prasad, & Quraishi, Citation2010; Ju, Kai, & Li, Citation2008; Yurt, Balaban, Kandemir, Bereket, & Erk, Citation2004). The corrosion phenomenon on carbon steels results from redox electrochemical reaction on metallic surfaces in environments consisting mostly of Cl−, SO42−, NO3− anions, etc., which gradually degrades the metallic alloy (Fajobi, Loto, & Oluwole, Citation2019). Previous research has shown that corrosion of metallic alloys can be controlled or minimized by the use of chemical compounds known as corrosion inhibitors (Loto et al., Citation2011, Citation2011; Loto & Loto, Citation2012; Loto, Citation2018; Zee et al., Citation2019). Corrosion inhibitors are chemical substances that modify and suppress the electrochemical properties and reaction mechanisms of corrosive environments in addition to their reaction with metallic surfaces exposed to the environment (Obot, Obi-Egbedi, & Umoren, Citation2009; Papavinasam, Citation2013; Popoola, Grema, Latinwo, Gutti, & Balogun, Citation2013). Research has shown that green, non-toxic organic derivatives are suitable alternatives to toxic chromates, nitrates and some organic compounds that have dominated the corrosion inhibitor market (Bao, Furumoto, Fukunaga, & Nakao, Citation2001; Furtado et al., Citation2019; Hossain et al., Citation2019; Loto, Citation2016; Loto, Loto, Joseph, & Olanrewaju, Citation2016; Rosli, Yusuf, Sauki, & Razali, Citation2019). Calcium gluconate (CGN) obtained from gluconic acid is a whitish crystalline needle compound with fine texture and low rate of solubility in water (Rajendran, Apparao, & Palaniswamy, Citation1998). The compound is used as medication and health mineral supplements. Application of CGN in acidic media, low chloride solutions and cooling has given mixed results necessitating further research (Akanji, Loto, Abdulwahab, & Kolesnikov, Citation2016; Gunasekaran, Palaniswamy, Apparao, & Muralidharan, Citation1996; Saremi, Benehkohal, Dehghanian, & Zebardast, Citation2009). This research is focused on study of the inhibition effect of CGN on mild steel (MS) in artificial seawater.
2. Experimental procedure
MS of cylindrical dimension (0.7 cm diameter) was manually cut into five test coupons. Each coupon was mounted in acrylic paste which hardened after 5 min, grinded with emery abrasive papers (80, 120, 240, 320, 400, 600, 800 and 1,000 grits), polished with diamond polishing paste and subsequently washed with distilled H2O and acetone for potentiodynamic polarization technique and open circuit potential (OCP) measurement. Seven grams of recrystallized NaCl was added to 200 ml of distilled H2O to formulate to simulate artificial seawater. CGN obtained from Sigma Aldrich, USA was prepared in volumetric concentrations of 0.75%, 1.25%, 1.75% and 2.25%% per 2,000 ml of artificial seawater. The compound is non-toxic with molecular weight of 430.373 g/mol with molecular formular of C12H22CaO14. Its molecular structure is shown in Figure . Potentiodynamic polarization test was done with Digi-Ivy 2300 potentiostat at 25°C ambient temperature. Resin mounted MS electrodes (exposed surface area of 0.38 cm2), Pt counter electrode and silver chloride reference electrode (Ag/AgCl) were immersed in artificial seawater solution, and connected to the Potentiostat–computer interface. Potentiodynamic measurement was performed between potentials of −1.5 V to 0.5 V at a scan rate of 0.0015 V/s. OCP measurement was performed for 9,000 s at 0.2 V step potential. Omax trinocular metallurgical microscope was used to study and capture images of MS surface before corrosion and after corrosion with and without CGN inhibitor.
3. Theory
System performance is limited by the precision and accuracy of the measuring instrument. The electrochemical system was checked for possible causes of systematic errors. Calibration of the instrument and hardware test was performed with the results shown in Table . Test for reproducibility of consistent results was also performed. Based on this assertion, the expected error in the experimentation from systemic hysteresis is less than 1%.
Table 1. Results from calibration and hardware test
Corrosion current density (CI) and corrosion potential (CJ) were calculated from the Tafel plots of potential versus log current. The corrosion rate (CR) was determined from EquationEquation (1)(1)
(1) .
where CI is the current density (µA/cm2), D is the density (g/cm3), Eq is the specimen equivalent weight (g). 0.00327 is a constant for corrosion rate calculation. The percentage inhibition efficiency (η) was calculated from corrosion rate values using the equation below;
where CR1 and CR2 are the corrosion rates with and without CGN inhibitor. Polarization resistance (Rp, Ω) was calculated from EquationEquation (3)(3)
(3) below;
where Ba is the anodic Tafel slope and Bc is the cathodic Tafel slope, both are measured as (V/dec). Optical microscopic images of control, non-inhibited and CGN inhibited MS surface were studied from captured images of MS surface before and after corrosion tests.
4. Results and discussion
4.1. Potentiodynamic polarization and microscopy characterization
Figure shows the potentiodynamic polarization plots of MS corrosion in artificial seawater (3.5% NaCl) at 0% CGN to 2.25% CGN. Table shows the data obtained from the polarization test. The optical microscopic images for MS surface before corrosion and after corrosion test in artificial seawater without CGN compound are shown in Figure . Figure shows the optical microscopic images of MS surface after corrosion in artificial seawater at 0.75% CGN and 2.25% CGN concentration. The corrosion rate of MS at 0% CGN is 1.56 mm/y which corresponds to corrosion current density of 1.37 × 104 A/cm2 due to the electrochemical action of Cl− anions within the electrolyte responsible for the oxidation of MS surface as shown in Figure ). This mechanism results in increased anodic dissolution and hydrogen evolution reactions on MS surface. The image shows the presence of corrosion pits on MS surface in addition to general wear due to oxidation of the substrate Fe2+. The presence of chlorides significantly aggravates the conditions for formation and growth of the pits on MS through an autocatalytic oxidation process. The small size of Cl− ions enables localized deterioration of MS surface (Loto, Citation2013). Migration of Cl− ions into micro-pits is enhanced to maintain electrical neutrality and hydrolysis of the corrosion products inside pits causing acidification, and hence pit enlargement and penetration. The mechanism is autocatalytic because the increased acidity accelerates the dissolution rate inside pits. It is well known that Cl− ions are aggressive enough to attack steel and initiate pitting (Abd El Meguid, Mahmoud, & Abd El Rehim, Citation2000; Ameer, Fekry, El-Taib, & Heakel, Citation2004; El-egamy & Badway, Citation2004; Phanis, Satpati, Muthe, Vyas, & Sundaresan., Citation2003; Yang & Luo, Citation2001). Oxidation of MS takes place according to the equation below leading to the formation of FeCl3 and further on Fe(OH)2.
Table 2. Potentiodynamic polarization data for MS corrosion inhibition in artificial seawater at 0–2.25% CGN concentration
The Fe3+ and Cl− ions combine to form FeCl3 and the overall balanced equation is
FeCl3 further dissociates according to the equations below
Fe3+ cation interacts with water shown below
The reaction mechanisms lead to the continuous degradation of MS surface in the electrolyte. The corrosion rate results for MS in the presence of CGN significantly differed from the control solution due to the effective inhibition effect of CGN. Corrosion rate of 0.92 mm/y was obtained at 0.75% CGN concentration, though this value is significantly lower than the value obtained at 0% CGN, its corresponding inhibition efficiency and corrosion current density are 40.72% and 8.10 × 105 A/cm2. In the presence of CGN molecules at 0.75% concentration, Cl− ions from the electrolyte migrate through the partially CGN protected MS surface. There is the possibility of the Cl− ion complexing with protonated CGN molecules. However, the rate of corrosion is only partially and insufficiently inhibited resulting in the diffusion of dissolving metal cations from the pit interior. The optical image of MS (Figure )) is a slight improvement of the image at Figure ) with fewer corrosion pits and less surface wear. Beyond 0.75% CGN concentration, inhibition efficiency of CGN (1.25–2.25% CGN) compound was generally above 80%. CGN inhibited the electrochemical action of Cl− ions due to strong attraction and coordination reactions to MS surface. The chelating ability of the carboxylic group of CGN caused the formation of stable complex CGN–Ca2+ in the neutral chloride solution according to the equation below:
Fe2+-gluconate reaction product precipitates on the anodic portions of MS and Ca2+ is released according to the equation below;
The released Ca2+ forms Ca(OH)2 on the cathodic portion of MS according to the equation below:
The protective reaction product of Fe2+-gluconate precipitate and Ca(OH)2 passivates on MS as shown in Figure ) and the extended passivated region of the inhibited polarization plots in Figure . The passivated regions of the polarization plots in the presence of specific concentrations of CGN are much wider than the plot without CGN, which confirms anodic inhibition effect of CGN on MS surface. The passivated region confirms surface coverage of MS surface by CGN molecules. The extent of morphological wear in Figure ) is significantly lower, the serrated edges are due to machining are quite visible and corrosion pits are completely absent. Observation of the corrosion potential in Table shows the maximum potential shift between the inhibited steel and control specimen is 75 mV in the anodic direction. This confirms the inhibitor to be mixed type with dominant anodic inhibition property (Amel Gharbi, Himour, Abderrahmane, & Abderrahim, Citation2018). This assertion is further confirmed from the variation of the anodic Tafel slope values, which shows the mechanism of anodic inhibition is through surface coverage and precipitation on anodic reaction sites on MS. This is further proven as earlier mentioned from the passivated region of the polarization plot following anodic polarization. The general similarity of the cathodic Tafel slope values and the cathodic portion of the polarization plot show the cathodic inhibition reaction involving H2 evolution and O2 reduction is under activation control (Dariva and Galio).
Figure 2. Potentiodynamic polarization plots for MS corrosion inhibition in artificial seawater at 0–2.25% CGN concentration
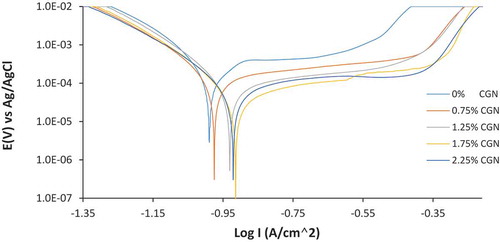
4.2. Coupon measurement
Graphical plots of MS corrosion rate versus exposure time are shown in Figure ) while Figure ) shows the plot of CGN inhibition efficiency versus exposure time. Table shows the results obtained from coupon measurement at 216 h. The corrosion rate of MS at 0% CGN concentration initiated at 0.0045 mm/y (48 h) due to oxidation of MS and progressively decreased to 0.00104 mm/y at 216 h due to weakening of the electrolyte. However, the corrosion rate value of MS obtained at this concentration (0% CGN) contrast the general corrosion rate value of MS obtained at 0.75% CGN concentration which initiated at 0.0008 mm/y (48 h) and peaked at 0.0007 mm/y (216 h). The inhibition efficiency of CGN at 0.75% concentration is quite poor at 33% and is unable to make any significant difference in protecting MS from the corrosive Cl− anions in artificial seawater. Further increase in CGN concentration (1.25% CGN −2.25% CGN) Decreased the corrosion rate values to MS to 0.00009 mm/y, 0.00002 mm/y and 0.00006 mm/y at 216 h. These values correspond to inhibition efficiency of final inhibition efficiencies of 90.97%, 97.87% and 94.58% that signifies effective inhibition of MS surface in artificial seawater. It must be noted that the presence of CGN compound in the electrolyte stabilized the thermodynamic properties of MS throughout the exposure period as shown in Figure . The plots from 0.75% to 2.25% CGN were generally constant. The corrosion rate and inhibition efficiency plots at 0% CGN varied with exposure time due to the electrochemical action of Cl− ion. The effect of Cl− decreased with exposure time.
Figure 5. Graphical plot of (a) MS corrosion rate versus exposure time and (b) CGN inhibition efficiency versus exposure time
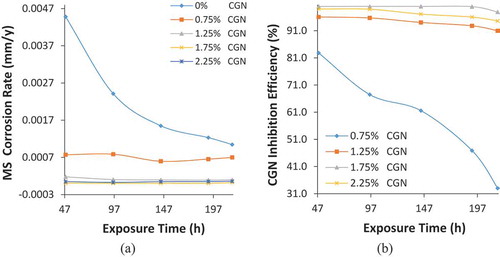
Table 3. Data obtained from coupon measurement at 216 h
4.3. Adsorption isotherm studies
The mechanism of CGN interaction on MS surface was further studied through adsorption isotherms models, which shows the extent to which inhibitor molecules adsorb on metallic surfaces in relation to its concentration at constant temperature (Karthikaiselvi & Subhashini, Citation2014). Langmuir and Frumkin isotherm models produced the most significant plots with correlation coefficients close to unity. Langmuir isotherm states metallic surfaces fixed region for adsorption of adsorbates with uniform Gibbs free energy values irrespective of differences in surface coverage value and the lateral interaction effect (Karimi, Danaee, Eskandari, & RashvanAvei, Citation2016). Figures and depict the plot of versus CCGN with correlation coefficient of 0.9898 with respect to the Langmuir equation below.
θ is the sum of inhibitor molecules adsorbed per unit gram on MS surface at equilibrium. Cinhibitor is inhibitor concentration and Kinhibitor is the equilibrium constant of adsorption. Frumkin isotherm states that molecular coverage of metallic surface depends on electrode potential due to variation in energy of double-layer capacitor which itself results from replacement of H2O molecules by molecules of chemical compounds with a lower dielectric constant (El-Aila, Elsousy, & Hartany, Citation2016). The metallic surfaces are heterogeneous and the lateral interaction effect is not negligible according to the equation below:
α is the lateral synergism parameter determined from the slope of the Frumkin isotherm plot. Plots of log versus θ (Figure ) results in correlation coefficient of 0.9780. The
4.4. Thermodynamics of inhibitor adsorption
Results for Gibbs free energy presented in Table were calculated from EquationEquation (11)(11)
(11) below.
Table 4. Results for Gibbs free energy (∆Gads), surface coverage (θ) and equilibrium constant of adsorption (Kads) for CGN adsorption on artificial seawater solution
55.5 is the molar concentration of H2O in the acid solution, R is the universal gas constant, T is the absolute temperature and Kinhibitor is the equilibrium constant of inhibitor adsorption on MS. The negative values of ΔGoads results show the spontaneous nature of adsorption (Bobina, Kellenbergera, Millet, Muntean, & Vaszilcsin, Citation2013). The highest ΔGoads value obtained for CG is −27.37 kJmol−1 while the lowest ΔGoads value is −18.23 kJmol−1. The highest and lowest ΔGoads values obtained show the adsorption mechanism of CGN inhibitor compound is by physical adsorption whose attraction is through weak Van der waals forces. The non-linear relationship between the Gibbs free energy values and CGN concentration shows lateral interaction effect between CGN molecules is negligible.
4.5. Open circuit potential measurement
The OCP plots of MS corrosion in artificial seawater solution at 0%, 0.75% and 2.25% CGN is shown in Figure . The plot at 0% CGN is the most electronegative due to active corrosion reactions on MS surface resulting in the formation of porous oxides. At this concentration, the OCP plot initiated at −0.580 V and sharply decreased to values associated with increased corrosion reactions before achieving relative stability at −0.657 V (600 s). There was a gradual decrease in corrosion potential to −0.683 V at 9,000 s. At 0.75% CGN concentration, the corrosion potential plot shifted significantly to electropositive values due to suppression of the redox electrochemical processes on MS. The OCP plot initiated at −0.450 V (0 s) and sharply decreased to −0.612 V at 1000.01 s. However, between this potential and the final potential at −0.663 V (9,000 s), miniature potential transients are visible on the OCP plot at 0.75% CGN. These transients are due to thermodynamic instability on MS surface resulting from the active-passive reaction between Cl− anions and protonated CGN molecules. The insufficient CGN molecules at 0.75% CGN were not able to completely hinder the diffusion of some Cl− anions unto the steel surface. At 2.25% CGN, the OCP plot shifted further to positive values signifying effective corrosion inhibition of MS surface. The OCP plot initiated at −0.421 V (0 s) and peaked at −0.630 V (9,000 s)
5. Conclusion
CGN effectively inhibited MS corrosion in artificial seawater with average inhibition efficiency above 80% and 90% from potentiodynamic polarization method and coupon measurement. The observation significantly contrasts the corrosion of MS in the electrolyte without inhibitor due to the presence of chlorides which significantly aggravates the conditions for formation and growth of the pits on the steel. At 0.75% inhibitor concentration, Cl− ions partially migrated to the steel surface resulting in the diffusion of dissolving metal cations and weak corrosion inhibition. The strong attraction and coordinated reaction of CGN onto the steel is responsible for its effective performance. The passivated regions of the polarization plots in the presence of specific concentrations of CGN are much wider than the plot without CGN. The presence of CGN stabilized the thermodynamic properties of MS compared to the non-inhibited steel. The thermodynamic tendency of MS to corrode decreased, with its corrosion potential shifting to electropositive values. CGN displayed mixed type inhibition with dominant anodic inhibiting effect. Thermodynamic calculations show the compound adsorbed through chemisorption mechanism according to Langmuir and Frumkin adsorption isotherms. Corrosion pits visible on the non-inhibited steel were absent on the inhibited steel.
Conflict of Interest
Authors declare no conflict of interest
Correction
This article has been republished with minor changes. These changes do not impact the academic content of the article.
Acknowledgements
The authors are grateful to Covenant University for their support of the research and funding for publication
Additional information
Funding
Notes on contributors
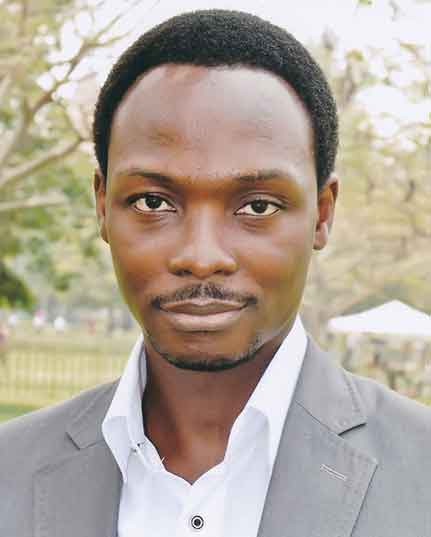
Roland Tolulope Loto
Loto Roland Tolulope is a Professor at the Department of Mechanical Engineering, Covenant University, Ogun State, Nigeria. He is the principal investigator of the corrosion and materials research cluster. Roland has over 155 research publications including reviews in top international journals and has consistently served as reviewer in respectable journals due to his in-depth knowledge and technical expertise. He has a lot of experience in research experimentation basically aimed at proffering solutions to the current depreciating effect of metallic degradation and failure in various engineering applications. He is a registered member of the Council for the Regulation of Engineering in Nigeria, South African Institute of Mining and Metallurgy and the Corrosion Institute of Southern Africa.
References
- Abd El Meguid, E. A., Mahmoud, N. A., & Abd El Rehim, S. S. (2000). The effect of some sulphur compounds on the pitting corrosion of type 304 stainless steel. Materials Chemistry and Physics, 63(1), 67–12. doi:10.1016/S0254-0584(99)00206-0
- Ahamad, I., Prasad, R., & Quraishi, M. A. (2010). Thermodynamic, electrochemical and quantum chemical investigation of some Schiff bases as corrosion inhibitors for mild steel in hydrochloric acid solutions. Corrosion Science, 52(3), 933–942. doi:10.1016/j.corsci.2009.11.016
- Akanji, O. L., Loto, C. A., Abdulwahab, M., & Kolesnikov, A. V. (2016). Anti-corrosion and computational study of mild steel in hydrochloric acid using calcium gluconate as inhibitor. Asian Journal of Chemistry, 28(7), 1417–1423.
- Ameer, M. A., Fekry, A. M., El-Taib, F., & Heakel, E. (2004). Electrochemical behaviour of passive films on molybdenum-containing austenitic stainless steels in aqueous solutions. Electrochimica Acta, 50(1), 43–49. doi:10.1016/j.electacta.2004.07.011
- Amel Gharbi, A., Himour, A., Abderrahmane, S., & Abderrahim, K. (2018). Inhibition effect of 2,2ʹ-Bipyridyl on the corrosion of austenitic stainless steel in 0.5M H2SO4. Oriental Journal of Chemistry, 34(1), 314–325. doi:10.13005/ojc/340134
- Bao, J., Furumoto, K., Fukunaga, K., & Nakao, K. (2001). A kinetic study on air oxidation of glucose catalyzed by immobilized glucose oxidase for production of calcium gluconate. Biochemical Engineering Journal, 8(2), 91–102. doi:10.1016/S1369-703X(00)00140-6
- Bobina, M., Kellenbergera, A., Millet, J.-P., Muntean, C., & Vaszilcsin, N. (2013). Corrosion resistance of carbon steel in weak acid solutions in the presence of l-histidine as corrosion inhibitor. Corrosion Science, 69, 389–395. doi:10.1016/j.corsci.2012.12.020
- El-Aila, H. J., Elsousy, K. M., & Hartany, K. A. (2016). Kinetics, equilibrium, and isotherm of the adsorption of cyanide by MDFSD. Arabian Journal of Chemistry, 9(1), S198–S203. doi:10.1016/j.arabjc.2011.03.002
- El-egamy, S. S., & Badway, W. A. (2004). Passivity and passivity breakdown of 304 stainless steel in alkaline sodium sulphate solutions. Journal of Applied Electrochemistry, 34(11), 1153–1158. doi:10.1007/s10800-004-1709-x
- Fajobi, M. A., Loto, R. T., & Oluwole, O. O. (2019). Corrosion in crude distillation overhead system: A review. Journal of Bio-and Tribo-Corrosion, 5(3), 67. doi:10.1007/s40735-019-0262-4
- Furtado, L. B., Nascimento, R. C., Seidl, P. R., Guimarães, M. J. O., Costa, L. M., Rocha, J. C., & Ponciano, J. A. C. P. (2019). Eco-friendly corrosion inhibitors based on Cashew nut shell liquid (CNSL) for acidizing fluids. Journal of Molecular Liquids, 284, 393–404. doi:10.1016/j.molliq.2019.02.083
- Gunasekaran, G., Palaniswamy, N., Apparao, B. V., & Muralidharan, V. S. (1996). Enhanced synergistic inhibition by calcium gluconate in low chloride media. Part I. Kinetics of corrosion. Proceedings of the Indian Academy of Sciences-Chemical Sciences, 108(4), 399–405. Springer India.
- Hossain, S. Z., Al-Shater, A., Kareem, S. A., Salman, A., Ali, R. A., Ezuber, H., … Razzak, S. A. (2019). Cinnamaldehyde as a green inhibitor in mitigating AISI 1015 carbon steel corrosion in HCl. Arabian Journal for Science and Engineering, 44(6), 5489–5499.
- Ju, H., Kai, Z. P., & Li, Y. (2008). Aminic nitrogen-bearing polydentate Schiff base compounds as corrosion inhibitors for iron in acidic media: A quantum chemical calculation. Corrosion Science, 50(3), 865–871. doi:10.1016/j.corsci.2007.10.009
- Karimi, A., Danaee, I., Eskandari, H., & RashvanAvei, M. (2016). Adsorption isotherm and inhibition effect of a synthesized di-(m-Formylphenol)-1,2-cyclohexandiimine on corrosion of steel X52 in HCl solution. Journal of Central South University, 23(2), 249–257. doi:10.1007/s11771-016-3068-2
- Karthikaiselvi, R., & Subhashini, S. (2014). Study of adsorption properties and inhibition of mild steel corrosion in hydrochloric acid media by water soluble composite poly (vinyl alcohol-o-methoxy aniline). Journal of the Association of Arab Universities for Basic and Applied Sciences, 16, 74–82. doi:10.1016/j.jaubas.2013.06.002
- Lorenzo, G. D., Rizzo, F., Formisano, A., Landolfo, R., & Guastaferro, A. (2019). Corrosion wastage models for steel structures: Literature review and a new interpretative formulation for wrought iron alloys. Key Engineering Materials, 813, 209–214. doi:10.4028/www.scientific.net/KEM.813
- Loto, C. A., Loto, R. T., & Popoola, A. P. I. (2011). Electrode potential monitoring of effect of plants extracts addition on the electrochemical corrosion behaviour of mild steel reinforcement in concrete. International Journal of Electrochemical Science, 6(8), 3452–3465.
- Loto, R. T. (2013). Pitting corrosion evaluation of austenitic stainless steel type 304 in acid chloride media. Journal of Materials and Environmental Science, 4(4), 448–459.
- Loto, R. T. (2016). Electrochemical analysis of the corrosion inhibition properties of 4-hydroxy-3-methoxybenzaldehyde on low carbon steel in dilute acid media. Cogent Engineering, 3(1), 1242107. doi:10.1080/23311916.2016.1242107
- Loto, R. T. (2018). Surface coverage and corrosion inhibition effect of Rosmarinus officinalis and zinc oxide on the electrochemical performance of low carbon steel in dilute acid solutions. Results in Physics, 8, 172–179. doi:10.1016/j.rinp.2017.12.003
- Loto, R. T., & Babalola, P. (2017). Corrosion polarization behavior and microstructural analysis of AA1070 aluminium silicon carbide matrix composites in acid chloride concentrations. Cogent Engineering, 4(1), 1422229. doi:10.1080/23311916.2017.1422229
- Loto, R. T., & Loto, C. A. (2012). Effect of P-phenylediamine on the corrosion of austenitic stainless steel type 304 in hydrochloric acid. International Journal of Electrochemical Science, 7(10), 9423–9440.
- Loto, R. T., & Loto, C. A. (2018). Corrosion behaviour of S43035 ferritic stainless steel in hot sulphate/chloride solution. Journal of Materials Research and Technology, 7(3), 231–239. doi:10.1016/j.jmrt.2017.07.004
- Loto, R. T., Loto, C. A., Joseph, O., & Olanrewaju, G. (2016). Adsorption and corrosion inhibition properties of thiocarbanilide on the electrochemical behavior of high carbon steel in dilute acid solutions. Results in Physics, 6, 305–314. doi:10.1016/j.rinp.2016.05.013
- Obot, I. B., Obi-Egbedi, N. O., & Umoren, S. A. (2009). Antifungal drugs as corrosion inhibitors for aluminium in 0.1 M HCl. Corrosion Science, 51(8), 1868–1875. doi:10.1016/j.corsci.2009.05.017
- Papavinasam, S. (2013). Corrosion control in the oil and gas industry. Amsterdam: Elsevier.
- Phanis, S. K., Satpati, A. K., Muthe, K. P., Vyas, J. C., & Sundaresan., R. I. (2003). Comparison of rolled and heat treated SS304 in chloride solution using electrochemical and XPS techniques. Corrosion Science, 45(2003), 2467–2483. doi:10.1016/S0010-938X(03)00099-4
- Popoola, L. T., Grema, A. S., Latinwo, G. K., Gutti, B., & Balogun, A. S. (2013). Corrosion problems during oil and gas production and its mitigation. International Journal of Industrial Chemistry, 4(1), 35. doi:10.1186/2228-5547-4-35
- Rajendran, S., Apparao, B. V., & Palaniswamy, N. (1998). Technical note Calcium gluconate as corrosion inhibitor for mild steel in low chloride media. British Corrosion Journal, 33(4), 315–317. doi:10.1179/bcj.1998.33.4.315
- Rizzo, F., Di Lorenzo, G., Formisano, A., & Landolfo, R. (2019). Time-dependent corrosion wastage model for wrought iron structures. Journal of Materials in Civil Engineering, 31(8), 4019165. doi:10.1061/(ASCE)MT.1943-5533.0002710
- Rosli, N. R., Yusuf, S. M., Sauki, A., & Razali, W. M. R. W. (2019). Musa Sapientum (Banana) peels as green corrosion inhibitor for mild steel. Key Engineering Materials, 797, 230–239. doi:10.4028/www.scientific.net/KEM.797
- Saremi, M., Benehkohal, N. P., Dehghanian, C., & Zebardast, H. R. (2009). Effect of calcium gluconate concentration and hydrodynamic effect on mild steel corrosion inhibition in simulated cooling water. Corrosion, 65(12), 778–784. doi:10.5006/1.3319104
- Yang, Q., & Luo, J. L. (2001). Effects of hydrogen and tensile stress on the breakdown of passive films on type 304 stainless steel. Electrochimica Acta, 46(6), 851–859. doi:10.1016/S0013-4686(00)00661-7
- Yurt, A., Balaban, A., Kandemir, S. U., Bereket, G., & Erk, B. (2004). Investigation on some Schiff bases as HCl corrosion inhibitors for carbon steel. Materials Chemistry and Physics, 85(2–3), 420–426. doi:10.1016/j.matchemphys.2004.01.033
- Zee, M., Chikkam, A. K., Larkin, E., Taheri, P., Rezaie, A., & Campbell, A. (2019). Corrosion risk assessment, failure analysis and corrosion mitigation for aboveground storage tanks and case histories. Corrosion 2019. Nashville, Tennessee, USA: NACE International.