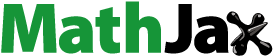
Abstract
The aim of this study is to evaluate the potential of cashew nutshell activated with H2SO4 as adsorbent for the removal of Cr and Mn (II) ions from wastewater. In this study, cashew nutshell (CNS) adsorbent was prepared using shells of cashew nut (Anacardium occidentale) by chemical activation. Cashew nutshells were crushed for size reduction and the resulting crushed shells were immersed in 1.0 mol L−1 H2SO4 at a rate of 1:10 (m/v) with a constant stirring at 60°C for 4 h. Proximate analysis was carried out according to standard analytical methods and determination of pH, bulk density, and iodine number was conducted. The morphology and functional groups present were investigated by Scanning Electron Microscopy (SEM) and Fourier Transform Infrared (FT-IR) spectroscopy. The surface area, pore volume, and pore size distribution were also characterized by N2 adsorption at 77 K using BET. Continuous adsorption study in a fixed-bed column was carried out by using CNS as an adsorbent for the removal of chromium and manganese ions from electroplating wastewater. The effect of flow rate, influent concentration, and bed depth were investigated on the adsorption characteristics of the CNS adsorbent. Three dynamic model Clark, Yoon-Nelson, and Adams-Bohart were applied using the experimental data to predict the breakthrough curves using linear regression to determine the characteristic parameters of the column that are useful for process design. Significant values in the moisture, ash content, volatile matter, iodine number, and zero-point of charge were obtained on the adsorbent and compared to literature. SEM images revealed irregular pore structure before adsorption and pores of the CNS adsorbent were filled with chromium and manganese ions after adsorption. The FT-IR adsorption bands observed in the CNS sample confirmed the presence of hydroxyl (-OH), carbonyl, and carboxylic (-COOH) groups involved in sorption of chromium and manganese ions onto surface of CNS adsorbent. The result showed highest BET specific surface area of the adsorbent sample obtained as 608.2 m2/g, pore volume and diameter of 0.2209 cm3/g and 2.116 nm, respectively. The column parameters calculated showed the effectiveness of the column at a flow rate of 5.0 ml/min, bed height of 10 cm and concentration of 20.3 mg/l Mn ion and 21.05 mg/l Cr ion. The percentage removal of Cr ions was 56.40% at an adsorption capacity of 10.79 mg/g and the percentage removal of Mn (II) ions was 53.09%, at an adsorption capacity of 9.82 mg/g. The Yoon-Nelson and Clark models were found suitable for the description of the breakthrough curve. Results reveal that quality cashew nutshell (CNS) adsorbent can be produced from Cashew nutshell thereby serving as an alternative to commercial adsorbent for heavy metal removal from wastewater.
PUBLIC INTEREST STATEMENT
Water contamination has been a major concern in developing countries through industrial activities from small and medium scale enterprises. Their technological processes generate a lot of wastewater which are discharged without treatment into the waterbodies and soil. Consequences of this are severe health challenges to human such as blindness in children, kidney failure, cancer, lungs related diseases, mutation, and even death. Aquatic habitats are also not left out. Bio-accumulation of heavy metals in the bodies of aquatic animals can be transferred to human through consumption. Cashew nutshell is an agricultural waste generated from cashew nuts processing plants. Recently, Nigeria witnessed increased demand of cashew from international market. The rinds of the cashew nuts collected from the boiler during the heating and shelling process are disposed indiscriminately, causing environmental problems. This study established that chemical activation of cashew nutshell adsorbed chromium and manganese ions in electroplating wastewater.
1. Introduction
The importance of water to the survival of the human race and aquatic life is vast. This is because it is a resource for sustaining the ecosystem, providing life-supporting services to people, animals, and plants. However, the discharge of heavy metals into aquatic ecosystems has become a matter of concern in Nigeria over the last few decades. Various reports indicate that water contamination can bring about low profitability, poor living conditions, and even death of living things (Garba et al., Citation2010). These pollutants are introduced into the aquatic systems significantly as a result of various industrial operations in the form of petroleum refining waste, mining waste, refining ores, sludge disposal, processing of radioactive materials, metal plating, or manufacturing of electrical equipment, paints, alloys, batteries, and pesticides or wood preservatives, among others (Ekubo & Abowei, Citation2011; Obayomi & Auta, Citation2019a).
Heavy metals such as lead, chromium, mercury, manganese, zinc, arsenic, cadmium, gold, silver, copper, and nickel are harmful to human health and other living organisms (Fu & Wang, Citation2011; Naser, Citation2013). A high concentration of chromium, for example, is released into the environment from metal ceramics, metal plating, stainless steel, chemicals, and tanning industries which is harmful to human health (Adeleken & Abegunde, Citation2011). Chromium has two main oxidation states: Cr (III) and Cr (VI) ions. Chromium (III) serves as an essential nutrient in balanced diet for both humans and animals. Its deficiency causes disturbance to the glucose and lipid metabolism in humans. However, chromium (VI) form is known to be toxic, non-biodegradable and carcinogenic (Devendra et al., Citation2017). It is highly mobile and soluble in water capable of causing skin allergy, stomach upset and ulcer, respiratory issues, immune system damage, kidney and liver diseases, lung cancer, and sometimes even death (Zhong et al., Citation2016).
Manganese is an essential metal ion for normal physiological functioning of human beings and animals. Exposure to low levels in the diet is nutritionally beneficial to healthy human life; however, chronic exposure to higher doses is detrimental to human health. It is toxic and its toxicity varies with route of exposure, chemical species, age, sex, and animal species (Kohl & Medlar, Citation2007). Syndrome called “manganism” result from chronic exposure to higher dosage and it is characterized by weakness, tremors, a mask-like face, and psychological disturbance among other symptoms (Dandang et al., Citation2017).
Some of the conventional technologies currently in use for adsorbing heavy metals from electroplating wastewater include; chemical precipitation/neutralization, ion exchange, extraction, membrane processes, evaporation, and adsorption. Their limitations have motivated the search for more efficient and cleaner technologies to overcome the ever-increasing threat to future water security. The search for new technologies involving the removal of toxic metals from wastewaters has directed attention to adsorption based on its cost and regenerating ability (Liang et al., Citation2010; Obayomi et al., Citation2020).
Adsorption is the most efficient and effective method, which has been used to adsorb heavy metals from wastewater especially when using commercial activated carbon (Jang et al., Citation2008; Obayomi et al., Citation2019). It is widely applied due to its simplicity, cost-effectiveness, and environmental friendliness (Karami, Citation2013). Adsorbent produced from activated carbon is often efficient because it has high porosity properties and high surface area, which makes it useful in batch and continuous mode operation. However, fast depletion of the sources of commercial activated carbon made the production of its raw material expensive and in addition, its regeneration produces additional effluents, resulting in considerable loss of the adsorbents (Zhao et al., Citation2011). Batch adsorption study has been used in previous studies for the removal of pollutants from wastewater using the cashew nutshell (Coelho et al., Citation2014; Kumar et al., Citation2012). Results indicated that it is a promising adsorbent for the substitution of activated carbon. Fewer studies are, however, available for its utilization for commercial treatment of large volumes of wastewater. The novelty of this study is in the use of the column adsorption technology. In addition, a nutshell makes 20% of the cashew nut and, according to data by FAO (Citation2013), world production capacity of cashew is 4.28 million tons, which invariably gives yearly production of the nutshells at approximately 856 thousand tonnes (Coelho et al., Citation2014). The environmental pollution generated by the high demands of cashew kernel, its liquid and oil has beamed searchlight for the setting up of small and medium enterprises that now engage in the manufacturing process.
Thus, the use of natural adsorbents can represent an excellent alternative, providing the same efficiency that activated carbon can give with lower costs. Adsorbents can be considered to be cheap or low-cost if it is abundant in nature, requires little processing, and is a by-product of industrial waste. Various waste materials, industrial by-products, agricultural wastes, and other natural waste materials are cheap and available in nature (Chu et al., Citation2015).
Mostly, agricultural wastes are used as bio-sorbents for wastewater treatment, because they are very efficient and promising in the bio-sorption technique. There are generally three types based on the sources: (i) non-living biomass, such as bark, lignin, shrimp, krill, squid, and crab shell; (ii) algal biomass; (iii) microbial biomass, such as algae, bacteria, fungi, and yeast. Agricultural wastes such as potato peel, sawdust, sugarcane beet (Yao et al., Citation2011), mango peel, corn cob, rice husk, tree fern, wheat bran, grape bagasse, coconut copra meal, orange waste, walnut, hazelnut, almond shell (Atmani et al., Citation2009), tea waste, sugarcane bagasse, peanut shell, tamarind seeds, cashew nutshell (Kumar et al., Citation2010), sunflower stalk and black gram husk (Feng et al., Citation2010; Hossain et al., Citation2012), in the preparation of bio-sorbents are very promising. Therefore, alternative materials from agricultural wastes, industrial by-products, and natural substances can provide the basis for the development of a new approach for heavy metal removal which are suitable for use in large quantities and have the ability to accumulate large amount of these heavy metals namely, Cu+2, Ni+2, Cr+6, Pb+2, Mn+2, Cd+2 (Coelho et al., Citation2014; Kumar et al., Citation2011).
Cashew nutshell is an agricultural waste, generated from the cashew nut processing industries. It is not suitable for consumption because of its low nutritional content. It is an abundant agricultural waste with good stability, wide availability, low cost and high metal sorption uptake from aqueous solutions and industrial waste effluents (Kumar et al., Citation2011).
Recently, Nigeria witnessed increased demand of cashew from international communities, which is attributed to the commercial importance of its main products; cashew nut (kernel), cashew apple, and cashew nutshell liquid (CNSL) (Azam-Ali & Judge, Citation2001). The shell is separated from the kernel by the Testa, which is a thin skin surrounding the kernel. The commercial method of removing the kernel from the shell was to subject the nuts to very low temperature by pan roasting causing the shell to become brittle. Thereafter, the nuts were mechanically cut to remove the kernel from the shell and further processed to obtain the cashew nutshell liquid. The rinds of the cashew nut (shells of the cashew nuts) are collected from a boiler during the heating and shelling process. After obtaining the cashew nuts and its oil, the solid wastes are disposed inappropriately in the soil, causing environmental problems. Studies have shown that simple chemical activation of the cashew nutshell adsorbent may increase its capacity to adsorb industrial pollutant with little cost. There is a paucity of literature that has reported the efficiency of the adsorbent to remove pollutants. As such, this agricultural waste will be investigated for its potential by using a fixed-bed column in treating electroplating wastewater containing chromium and manganese ions. The use of the column adsorption technology is relevant in the treatment of wastewater in large quantities. The batch adsorption could only predict behaviours of the adsorbent and its removal of heavy metals at micro level. To solve the emergence of CNS wastes from the cashew manufacturing company, a pilot plant would be desirable for a healthy ecosystem.
2. Materials and methods
2.1. Materials
All the reagents (sulphuric acid, distilled water) used were purchased from a commercial chemical laboratory and are of analytical grade with percentage purity in the range of 98.0–99.0%. The agricultural waste used in preparation of the adsorbent is rinds of cashew nutshells (CNS), which was obtained from the recovery unit of a local processing factory located in Ilorin, Kwara State, Nigeria.
2.2. Sampling and preparation of adsorbent
The shells of cashew (CNS) were cleaned thoroughly with distilled water to remove earthy impurities, and sun dried for 7 days. The shells were crushed for size reduction using mortar and pestle. The resulting material was sieved in a standard sieve mesh for particle size between 0.035 and 0.25 mm followed by chemical pre-treatment of the crushed shells. This was carried out by immersing the crushed shells in 1.0 mol L−1 H2SO4 at a rate of 1:10 (m/v) with constant stirring for 4 h at 60°C. Thereafter, the dehydrated cashew nutshell adsorbent was continuously washed with distilled water in order to remove the excess chemicals. The washing continued until the pH value of supernatants reaches 7.0. The resulting pre-treated material was dried at 50°C for 6 h to remove moisture content. The sample was stored as cashew nutshell (CNS) adsorbent prior to use as bio-sorbent for the column adsorption experiments.
2.3. Characterization of adsorbent materials
The concentration of chromium and manganese ions in the electroplating wastewater was determined in a chemical laboratory using Atomic Absorption Spectrophotometer (AAS). Proximate analysis such as bulk density, moisture content, ash content, volatile matter, and fixed carbon content were determined by applying standards analytical procedures. The adsorbent (CNS) prepared was characterized using the Fourier transform infrared spectroscopy (FTIR) for its functional group identification, scanning electron microscope (SEM) for its surface morphology and, determination of its surface area and pore volume analysis by Brunauer-Emmett-Teller (BET).
2.4. Fixed-bed column adsorption experiment
Column adsorption experiments were carried out in a glass column of 1.6 cm internal diameter and height of 30 cm. Each bed of the adsorbent was underlain by 0.5 mm of glass wool and 2 cm of sandstones. The essence of the glass wool and sandstones is to improve the flow distribution and prevent loss of adsorbent. The column was parked to a height of 4 cm, 8 cm, and 10 cm with known weight of CNS adsorbent on layer of glass wool placed at the bottom of the column. Metal ion solution having an initial concentration of Cr ions (21.05 mg/l) and Mn (II) (20.36 mg/l) at pH (2.0) was pumped through the column at a desired varying flow rate. Metal ions solution was collected from the exit of the column at different time interval of 2 h (120 min) for residual metal ions concentration and was analyzed using an atomic absorption spectrophotometer (AAS). Experiment continued until the concentration at the outlet of the column almost equals the concentration at the inlet. All experiment was carried out under room temperature and data obtained was used to plot the breakthrough curves of residual Cr ions and Mn (II) ions. The breakthrough curves of bio-sorption will be derived as function of equilibrium time, bed height, concentration, and flow rate.
The effect of feed flow rate on adsorption was investigated by varying feed flow rate at 5, 7, and 10 ml/min, while maintaining a constant bed height of 8 cm and initial metal ions concentration of Cr ions and Mn (II) ions (21.05 and 20.36 mg/l), respectively.
In the first stage, the bed height was changed (4.0, 8.0 and 10.0 cm) corresponding to 3.7, 4.9, and 6.6 g dry weight of CNS, respectively. This is to assess the height required for optimal removal, while the inlet concentration Cr ions and Mn (II) ions in the feed and the flow rate were held constants at (21.05 and 20.36 mg/l) and (5.0 ml/min) respectively.
The effect of the inlet concentration on adsorption was studied by varying the inlet concentration while maintaining a constant adsorbent bed height of 8 cm and feed flow rate of 5.0 ml/min. The removal efficiency is calculated on the inlet and outlet effluent concentration using EquationEquation 1(1)
(1) .
where Cin (mg/l) and Cout (mg/l) are the influent and effluent metal ion concentrations, respectively. The adsorbent capacity of the target metal species was determined by the concentration before and after adsorption using EquationEquation 2(2)
(2) .
where t is treatment time (h), Q is the flow rate (ml/min), m is the total mass of adsorbent in the column (g).
2.5. Adsorption column performance
The volume of effluent treated, Veff (ml) is calculated using EquationEquation 3(3)
(3) .
where Q is the volumetric flow rate (ml/min) and ttotal is the total flow time in minutes.
The total adsorbed metal quantity (qtotal, mg) in the column is represented by the area under the plot of the adsorbed metal ion concentration, which is calculated through numerical integration, given b EquationEquation 4(4)
(4) ;
where Q corresponds to the solution flow rate (mL/min), C0 is the influent concentration (mg/L), Ct is the effluent concentration (mg/l) at any time t (min), and A refers to the area under the breakthrough curve from C0 to Ct(mg min/l) from time 0 to any time t.
The total metal ion sent to the column mtotal in (mg) can be deduced from EquationEquation 5(5)
(5) (Yahya & Odigure, Citation2015).
The percentage removal of the metal ion as given by EquationEquation 7(7)
(7) can be evaluated as the ratio of mass of metal adsorbed (qtotal) to the total mass of metal ion sent to the column (mtotal)
The total metal uptake at equilibrium, (mg/g), is computed using EquationEquation 7(7)
(7) .
where m (g) corresponds to the mass of adsorbent.
2.6. Adsorption mathematical models
The dynamic behavior of the columns was predicted using Clark, Yoon-Nelson and Adams-Bohart models. The models are important when designing an efficient fixed-bed adsorption system.
3. Results and discussion
3.1. Adsorbent characterization
The initial concentration of the metal ions present in the wastewater (Table ) was found to be higher compared to maximum permissible limit set by the World Health Organization (WHO, World Health Organization, Citation2015).
Table 1. Initial metal ion concentration and maximum permissible limit
Higher bulk densities serve better filterability of the adsorbent as adsorbent with a bulk density of 0.47 g/cm3 is adequate to retain substantial amount of liquid when compared to activated carbons with bulk density of 0.5 g/cm3 (Okieimen et al., Citation2007). Adsorbent prepared by chemical activation exhibited higher iodine adsorption capacity (492.3 mg/g) indicating improved porosity. The prepared CNS has low mineral matter content as indicated by the value of ash content (1.15%) which is lower compared to result of Kumar et al. (Citation2011) who reported ash content value of (2.75%). It can affect adsorbent as it reduces the overall activity of bio-materials, the lower the ash content the better the overall activity of the adsorbent. The lower carbon yield could be due to method of preparation applied. The CNS volatile matter of 71.05% was in close agreement with the ASTM D-3175 standard of 72% when compared. Therefore, the proximate analysis results obtained shows that CNS can serve as a good adsorbent.
The FTIR analysis illustrated in (Figure ) shows that before and after adsorption the CNS have a variety of functional groups, such as hydroxyl, and carboxyl groups, which may be involved in the potential binding of metal ions onto the adsorbents. The FTIR spectrum of CNS before adsorption as compared to that of CNS after adsorption is different. There is also a change in the spectrum observed, which confirms the modification. Based on the result obtained, there was an apparent shift in transmittance from 3446.01 cm−1 to 3446.66 cm−1 which correspond to a stretch vibration of O-H groups indicating physisorbed moisture on the adsorbent surface (Olutoye & Hameed, Citation2013). High carbonization due to sulphuric acid treatment is also very clear by the decrease in the intensity of the peak from 2924.37 to 2301.35 cm−1 due to the—CH2—stretching vibrations. The medium peaks 1635.66 cm−1 which shifted to 1636.95 cm−1 can be attributed to the binding between Cr ions and Mn (II) ions with C = O bond which confirms the presence of carbonyl groups (Saka et al., Citation2012). The conversion of the alcoholic groups into ethers is also clearly evident by the well-resolved—C–O–C—asymmetric vibrations peaks obtained at 1123.80 and 1036.71 cm−1.
The surface morphology of the CNS before (Figure ) metal ion adsorption was observed to have created irregular pores on the surface of the CNS due to effect of the sulphuric acid solution and after metal ion adsorption; the CNS surface becomes agglomerated and packed (Figure ). The spaces showed no pores, demonstrating that the pores had been filled with Cr6+ and Mn2+ ions trapped (Kumar et al., Citation2011). This also confirms that the sulphuric acid-treated CNS adsorbent has a more desired surface morphology for metal ion adsorption than the untreated CNS adsorbent.
The presence of C (73.61%), O (23.27%), Al (0.92%), and N (2.2%) peaks on CNS adsorbent before adsorption were observed and after adsorption CNS loaded with metal ions were shown to have the following elements; C (41.26%), O (22.29%), Mn (12.59%), Cr (4.13%), Na (1.44%), Al (0.90%), and Pb (10.84%) with additional peaks such as N (2.08%), I (1.68%), S (1.44), F (1.36%) were observed to be present as shown in Figure (a,b).
The test conducted on the CNS adsorbent material showed that the surface area is 608.2 m2/g and a pore volume of 0.221 cm3/g at a correlation coefficient (R2) of 0.74216. The BET surface area indicates large number of active sites and this value is high compared to Coelho et al. (Citation2014) who reported 0.5092 m2/g and 0.00052 cm3/g. According to International Union of Applied Chemistry (IUPAC), pores are classified into three namely; micropores (<2 nm diameter), mesopores (2–50 nm diameter), and macropores (>50 nm). The CNS adsorbent prepared exhibited mainly mesopores with a pore size of 2.116 nm, which makes it suitable for liquid-phase adsorption (wastewater treatment). The diameter of Chromium and Manganese ions was observed to be 0.256 nm and 0.322 nm. This indicates that Chromium has the highest affinity to be adsorbed onto the pores of the prepared CNS adsorbent. The adsorption process has been reported to be either surface area or functional group dependent.
3.2. Effects of flow rate
Figure ,b) depicts the breakthrough curves for adsorption of Mn ion onto CNS adsorbent for different flow rates at bed height of 8.0 cm and concentration of 20.36 mg/l and breakthrough curves for adsorption of Cr ion onto CNS adsorbent for different flow rate at bed height of 8.0 cm and concentration of 21.05 mg/l. The impact of the solution flow rates on the shape and parameters of the breakthrough curves were examined in this sub-section. Results showed that the metal ions uptake onto CNS adsorbent decreases with an increase in flow rate through the adsorbent bed. The volume of effluent (Veff) treated efficiently also reduces as the flow rate increases. This occurs due to the decrease in contact time between the metal ions and the sorbent, which do not allow full saturation of the active sites by the metal ions and eventually leads to decrease in the amount of metal ions adsorbed (Chen et al., Citation2012). The result indicates that adsorption of metal ions is strongly dependent on the flow rate. At lower flow rate of 5 ml/min, the sorption of Mn2+ and Cr6+ increases `steadily and there is delay in the saturation of the sorbent (Figures and ). At higher flow rates, saturation of the bed is easily attained. To attain saturation at lower flow rate, the contact time must be extended. As the influent flow continues, there is a gradual saturation of the sites and less effectiveness in the metal ions uptake is reached at a point where the influent concentration equals effluent concentration, implying that the bed is saturated (Yahya & Odigure, Citation2015).
3.3. Effects of bed height
Figure (a,b) depicts the breakthrough curves for adsorption of Mn ion onto CNS adsorbent for different bed height at flow rate of 5.0 ml/min and concentration of 20.36 mg/and breakthrough curves for adsorption of Cr ion onto CNS adsorbent for different bed height at flow rate of 5.0 ml/min concentration of 21.05 mg/l. The increase in bed depth leads to increase in the service time to breakthrough and exhaustion of the sorption column. From Figure (a), with increase in bed depth from 4 cm to 10 cm, the service time at breakthrough increased from 120 min (2 h) to 600 min (10 h), while the service time at exhaustion (saturation) increased from 360 min (6 h) to 1080 min (18 h). The increasing trend in the service time to breakthrough and exhaustion could be explained by the fact that as the amount of the CNS adsorbent increases in the column, the bed depth gets longer and more active sites of the adsorbent are available for the sorption process (Sarma et al., Citation2015). As such, longer time is required to get the metal ions fully saturated on the CNS. It is also observed that both the breakthrough curve and the saturation time increased with increase in bed height as there exist a large amount of sorbent for the solute to travel before exit from the column.
3.4. Effects of initial metal ion concentration
The study of the effect of the initial metal ion concentration on metal ions removal in Figure (a,b) shows, that there is a decrease in metal ion removal with an increase in its initial metal ion concentrations. But it is also observed that there is an increase in the adsorption capacity with an increase in the metal ion concentration. The decrease in the percentage of metal ion removal can be attributed to the saturation of the available active sites on the adsorbent beyond a certain initial metal ion concentration. The increase in the adsorption capacity may be due to the higher adsorption rate and the utilization of all the available active sites for adsorption at a higher initial metal ion concentration.
Figure 7. Effects of initial metal ion concentration (a) for adsorption of Mn (II) ion onto CNS adsorbent for different concentration at bed depth of 4 cm and flow rate of 5.0 ml/min. (b) for adsorption of Cr ion onto CNS adsorbent for different concentration at bed depth of 4 cm and flow rate of 5.0 ml/min
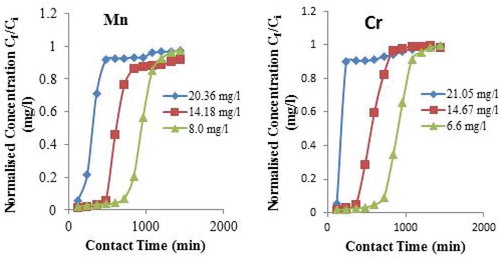
3.5. Evaluation of column performance
The total Mn2+ fed to the column at a flow rate of 5 ml/min was 122.19 mg, out of which 64.97 mg was adsorbed as shown in Table . At flow rate of 7 ml/min, total Mn2+ sent was 153.96 mg and 43.68 mg was absorbed, while at flow rate of 10 ml/min, total Mn2+ sent was 195.51 mg and 19.60 mg was adsorbed. The percentage removal of Mn2+ at flow rate of 5 ml/min was higher at 53.09% than the percentage removal at 7 ml/min and 10 ml/min, which was 28.37% and 10.30%. Similarly, total Cr ion sent to the column at a flow rate of 5 ml/min was 126.32 mg out of which 71.25 mg was adsorbed. At a flow rate of 7 ml/min, total Cr6+ sent was 159.17 mg out of which 43.96 mg was adsorbed, while at a flow rate of 10 ml/min, total Cr sent was 202.12 mg out of which 20.29 mg was adsorbed. The percentage removal of Cr at a flow rate of 5 ml/min was much higher at 56.40% than that at 7 and 10 ml/min, which was 27.60% and 10.04%. The total metal ion (mtotal) sent to the column is a function of the flow rate (Q) and time (t), though the total metal ion sent are much more at higher flow rate, the amount of metals adsorbed is smaller compared to the amount adsorbed at lower flow rate as observed by (Yahya & Odigure, Citation2015). This is because at higher flow rates, the contact time gets shorter; thus, disallowing the full saturation of the active sites by the metal ions, leading to a decrease in the amount of metal ions adsorbed (Chen et al., Citation2012). The contact time at lower flow rates increased enabling more interaction of metal ions with the sorbent, allowing inter-particle diffusion to take place. In other words, allowing diffusion of a larger number of ions into the pores of the sorbent resulting in more metal ion being adsorbed.
Table 2. Column data parameter obtained on sorption characteristics of CNS adsorbent at varying flow rates
The study of the effect of the bed height (adsorbent dosage) on the adsorption of metal ions shows that the percentage of metal ions removed increases with an increase in the adsorbent dosage due to an increase in the surface area.
The amount of metal ion adsorbed and percentage removal increases with increase in bed height as presented in Table . For Mn (II) ion adsorption, there is an increase in the amount of Mn (II) ion adsorbed from 10.52 to 50.26 mg as the bed depth increase from 4 to 10 cm, while percentage removal increases from 10.77% at 4.0 cm to 41.13% at 10 cm bed depth. Also, for Cr ions ion adsorption, the amount adsorbed increased from 11.54 to 59.38 mg as the bed depth increases from 4 cm to 10 cm, while the percentage removal increases from 11.42% at 4 cm to 47.0% at 10 cm bed depth. The increase is because of increase in the amount of sorbent in the column, which provided more binding sites for the metal ion.
Table 3. Column data parameters obtained on sorption characteristics of CNS adsorbent at varying bed heights
Results depicted in Table , showed that the mass of Mn2+ and Cr6+ ion adsorbed, qtotal(in mg) and the percentage removal decreased with increasing influent concentration (Ci) of Mn2+ and Cr6+. For Mn2+ adsorption, the amount adsorbed decreases from 26.7 to 11.52 mg as the concentration increases from 8.0 to 20.3 mg/l, while the percentage removal decreases from (56%) at 8.0 mg/l to (12%) at 20.4 mg/l. Also, for Cr6+ adsorption, the amount adsorbed decreases from 21.6 mg to 11.9 mg as the concentration increases from 6.6 mg/l to 21.05 mg/l, while the percentage removal decreases from (55%) at 6.6 mg to (12%) at 21.05 mg/l. This could be due to the shorter service time of the column as Mn2+ and Cr6+ concentration increases. Most often, percentage metal uptake (qtotal) decreases with increasing metal ion concentration (Wong et al., Citation2003). Again, diffusion process of the metal ions into the sorbent is concentration-dependent as the results indicated that increase in concentration changes the rate of adsorption through adsorbent bed and increases the bed capacity. Meanwhile, breakthrough is reached before the binding sites of the adsorbent get saturated by the metal ions (Yahya & Odigure, Citation2015).
3.6. Column adsorption isotherms
It is observed that as the flow rate (Q) increases from 5 to 10 ml/min at constant bed depth (L) of 8 cm, the rate constant (KYN) increases with little significance while the time required for 50% breakthrough () decreases from 1178 min (20 h) to 410 min (7 h) (see Table ). This shows that earlier breakthrough is reached at higher flow rate and less residence time of metal ion to move through the length of the sorption column (Yahya & Odigure, Citation2015). However, as the bed depth increases from 4 to 10 cm, the rate constant increases and the time required for 50% breakthrough (
) increases from 421 min (7 h) to 823 min (14 h). Also, from Table , as flow rate (Q) increases from 5 to 10 ml/min at constant bed depth (L) of 8 cm, the rate constant (KYN) increases with little significance while the time required for 50% breakthrough (
) decreases from 1129 min (19 h) to 410 min (0.8 h). As the bed depth increases from 4 to 10 cm, the rate constant increases and the time required for 50% breakthrough (
) increases from 415 min (7 h) to 1080 min (18 h). This is in agreement with some literature findings (Chowdhury et al., Citation2013).
Table 4. Column data obtained on sorption characteristics of CNS adsorbent at varying concentration
Table 5. Yoon-Nelson model parameters for Mn2+sorption at different operating conditions
Table 6. Yoon-Nelson model parameters for Cr6+ sorption at different operating conditions
Adams-Bohart was applied to test the accuracy and reliability of experimental data, as well as to calculate the kinetic constants of the adsorption process of both metals (Tables and ). The values of No at all conditions have significant difference and, this is as a result of more saturation sites, adsorption capacity of the adsorbent decreased with increasing flow rate. The values of KAB increased with both initial influent concentration and flow rate increase, but however, decreased with increase in bed depth. This showed that overall system kinetic is controlled by external mass transfer in the initial part of the adsorption in the column.
Table 7. Adams-Bohart model parameters for Mn2+ sorption at different operating conditions
Table 8. Adams-Bohart model parameters for Cr6+ sorption at different operating conditions
Among the Clark, Yoon-Nelson and Adams-Bohart models studied, the value of error (SSE) for Yoon-Nelson model was least for a given experimental condition, while it was highest for Clark model. Thus, it is concluded that the Yoon-Nelson model is better in describing the process of Mn2+ and Cr ions adsorption in the fixed-bed column. In comparison of values of SSE of the predicted and the experimental data, both the Yoon-Nelson and Adams-Bohart models can be used to describe behavior of the adsorption process.
3.7. Adsorption mechanism
The mechanism of Cr and Mn (II) ion uptake by cashew nutshell is proposed by the participating hydroxyl and carbonyl groups in the reaction as indicated by the FTIR before and after adsorption. The HCrO4− and Mn2+is attracted to the positively charged surface and donates protons per each mol of its monovalent and divalent ions into the wastewater. The hydrated metallic ion loses its water of hydration. Finally, CNS carbonyl and hydroxyl groups bind with the non-solvated metallic ion. At lower pH value, the adsorption process is effective in eliminating metallic ions of Cr and Mn (II) ions. Comparison of adsorption capacity of metal ions onto cashew nutshell adsorbent and with other adsorbents is depicted in Tables and 1 respectively.
Table 9. Comparison of adsorption capacity of metal ions onto cashew nutshell adsorbent
Table 10. Comparison of adsorption capacity of cashew nutshell with other adsorbents
4. Conclusion
This work investigated the sorption of Chromium and Manganese ions by cashew nutshell (CNS) adsorbent from electroplating effluents under various parameters. The major findings of the study are:
Cashew nutshell (CNS) adsorbent was successfully prepared by sulphuric acid activation. The characterization results revealed presence of functional groups such as hydroxyl (-OH), carbonyl (-CO), carboxyl (-COOH), and aliphatic (-CH2) groups, which are involved in the binding of the metal ions onto the adsorbents. The SEM-EDX image of the CNS indicated an irregular pattern and porosity of the CNS. The EDX spectrum confirmed the appearance of the adsorbed metallic ions. The pore spaces were agglomerated and packed after the adsorption. The BET test revealed that the surface area and pore volume are large enough to allow deposition of Mn2+ and Cr6+ ions on the molecular sites of the adsorbent.
Continuous column adsorption studies on chromium and manganese ions removal showed significant effects of the variables and that sorption capacity strongly depends on flow rate, bed height, and initial metal ion concentration. Breakthrough curves were derived under varying flow rate, bed height, and initial metal concentration. Results indicated that the breakthrough time, exhaustion time, and adsorption capacity at breakthrough, increased with the decreasing flow rate and initial concentration and increasing bed height. The adsorption preference of CNS could be arranged in the order of Cr(VI)>Mn(II). Experimental breakthrough curves were observed to be in good agreement with theoretical values by Yoon-Nelson and Adams-Bohart models, which were validated by high R2 and low SSE values.
Additional information
Funding
Notes on contributors
K. S. Obayomi
Muibat Diekola Yahya is a PhD holder in Chemical Engineering and lectures at the Federal University of Technology, Minna, Nigeria. Her area of specialization is in waste management, environmental engineering, pollution and control. The indiscriminate release of toxic compounds in the form of heavy metals, contaminants and pollutants from small, medium scale enterprise has been a major challenge in developing nation and had spurred continuous research in waste managements. Agricultural wastes such as shea butter husk/cake, almond husk, kola nuts pods, maize tassel, cashew nuts shell, melon husk, to mention a few are among successful biomaterials that have been used for the decontaminations of wastewater. The application of column adsorption process at optimum conditions will reduce the heavy metals below threshold levels before their disposal to the water bodies. This will remediate the environment and increase in job opportunity and revenue generation to the cashew processing company.
References
- Adeleken, B., & Abegunde, K. (2011). Heavy metal contamination of soil and ground water at automobile mechanic village in Ibadan, Nigeria. International Journal of the Physical Sciences, 6(5), 1045–18. http://doi.org/10.2897/IJPS10.495
- Albadarin, A. B., Mangwandi, C., Walker, G. M., Allen, S. J., Ahmad, M. N. M., & Khraisheh, M. (2013). Influence of solution chemistry on Cr(VI) reduction and complexation. Journal of Environmental Management, 114, 190–201. https://doi.org/10.1016/j.jenvman.2012.09.017
- Atamani, F, Bensmaili, A, & Mezenner, N.Y. (2009). Synthetic textile effluent removal by skin almonds waste. Journal Of Environmental Sciences and Technology, 2(4), 153-169. https://doi.10.3923/jest.2009.153.169
- Azam-Ali, S. H., & Judge, E. C. (2001). Small scale cashew nut processing. Intermediate Technology Development Group. Schumacher Center for Technology Development, Bourton Dunsmore, Rugby, Wickshire, CV39QZ, UK.
- Basci, N., Kocadagistan, E., & Kocadagistan, B. (2004). Biosorption of copper (II) from aqueous solutions by wheat shell. Desalination, 164(2), 135–140. https://doi.org/10.1016/S0011-9164(04)00172-9
- Blanesa, P. S., Bordoni, M. E., Gonzáleza, J. C., Garcíaa, S. I., Atriac, A. M., Sala, L. F., & Bellú, S. E. (2016). Application of soy hull biomass in removal of Cr(VI) from contaminated waters. Kinetic, thermodynamic and continuous sorption studies. Journal of Environmental Chemical Engineering, 4(1), 516–526. https://doi.org/10.1016/j.jece.2015.12.008
- Chen, S., Qinyan, Y., Baoyu, G., Li., Q., Xing, X., & Kaifang, F. (2012). Adsorption of hexavalent chromium from aqueous solution by modified corn stalk: A fixed-bed column study. Bioresource Technology, 113 (2012), 114–120. Elsevier. https://doi.org/10.1016/j.biortech.2011.11.110
- Chowdhury, Z. Z., Zain, S. M., Rashid, A. K., Rafique, R. F., & Khalid, K. (2013). Breakthrough curve analysis for column dynamic sorption of Mn(II) ions from wastewater by using mango stanagarcinia peel-based granular-activated carbon. Journal of Chemistry, 959761, 1–8. https://doi.org/10.1155/2013/959761
- Chu, L., Liu, C., Zhou, G., Xu, R., Tang, Y., Zeng, Z., & Luo, S. (2015). A double network gel as low cost and easy recycle adsorbent: Highly efficient removal of Cd (II) and Pb (II) pollutants from wastewater. Journal of Hazardous Materials, 300, 153–160. https://doi.org/10.1016/j.jhazmat.2015.06.070
- Coelho, G. F., JrAC, C., Tarley, C. R. T., Casarin, J., Nacke, N., & Francziskowski, M. A. (2014). Removal of metal ions Cd(II), Pb(II) and Cr(III) from water by the cashew nut shell (anarcadiumoccdentale L). Ecological Engineering, 73, 514–525. https://doi.org/10.1016/j.ecoleng.2014.09.103
- Dandang, H., Guangcai, W., Zheming, S., Zhihong, L., Fei, K., & Fei, L. (2017). Removal of Hexavalent Chromium in Natural 1 Groundwater using Activated Carbon and Cast Iron Combined System. Journals of Cleaner Energy Production, 165, 667-676. https://doi.org/10.1016/j.jclepro.2017.07.152
- Devendra, K. S., Vijay, K., Sweta, M., Daraksha, B., & Syed, H. (2017). Breakthrough curve modeling of graphene oxide aerogel packed fixed bed column for the removal of Cr(VI) from water. Journal of Water Process Engineering, 18, 150–158. https://doi.org/10.1016/j.jwpe.2017.06.011
- Ekubo, A. T., & Abowei, J. F. N. (2011). Aspects of aquatic pollution in Nigeria. Research Journal of Environmental and Earth Sciences, 3(6), 673–693.hhtps://maxwellsci.com/print/rjees/v3-673-693.pdf.
- FAO - Food and Agriculture Organization of the United Nations. (2013, August 15). Statitics (FAOSTATS) 2013. http://faostat.fao.org/site/291/default.aspx
- Feng, Y., Gong, J. L., Zeng, G. M., Niu, Q. Y., Zhang, H. Y., Niu, C. G.,. D., . J. H., & Yan, M. (2010). Adsorption of Cd (II) and Zn (II) from aqueous solutions using magnetic hydroxyapatite nanoparticles as adsorbents. Chemical Engineering Journal, 162(2), 487–494. https://doi.org/10.1016/j.cej.2010.05.049
- Fu, F., & Wang, Q. (2011). Removal of heavy metal ions from wastewaters: A review. Journal of Environmental Management, 92(3), 407–418. https//doi.org/10.1016/j.jenvman.2010.11.011
- Garba, Z. N., Hamza, S. A., & Galadima, A. (2010). Arsenic level speciation in fresh water from Karaye Local Government Area, Kano State, Nigeria. International Journal of Chemistry, 20(2), 113–117.
- Hossain, M. A, Ngo, H. H, Guo, W. S, & Nguyen, T. V. (2012). Palm oil fruit shells as bio-sorbent for copper removal from water and wastewater: experiments and sorption models. Bioresource Technology, 113, 97–101. https://doi.org/10.1016/j.biortech.2011.11.111
- Jang, M., Chen, W., & Cannon, F. S. (2008). Preloading hydrous ferric oxide into granular activated carbon for arsenic removal. Environmental Science & Technology, 42(9), 3369–3374. https://doi.org/10.1021/es7025399
- Karami, H. (2013). Heavy metal removal from water by magnetite nanorods. Chemical Engineering Journal (Amsterdam, Neth.), 219, 209–216. http://dx.doi.org/10.1016/j.cej.2013.01.022
- Kohl, P., & Medlar, S. (2007). Occurrence of Mn(II) in drinking water and Mn(II) control. IWA Publishing, 3, 16–18.
- Kumar, P. S., Ramalingam, S., Sathyaselvabala, V., Kirupha, S. D., & Sivanesan, S. (2011). Removal of copper (II) ions from aqueous solution by adsorption using cashew nut shell. Desalination, 266(1-3), 63-71.
- Kumar, P. S., Ramalingam, S., Sathyyaselvabala, V., Kirupha, S. D., Murugesa, A., & Sivaanesan, S. (2012). Removal of cadmium (II) from aqueous solution by agricultural waste cashew nutshell. Korean Journal of Chemical Engineering, 29(6), 756–768. https://doi.org/10.1007/s11814-011-0259-2
- Kumar, P. S., Ramalingam, S., Senthamarai, C., Niranjanaa, M., Vijayalakshmi, P., & Sivanesan, S. (2010). Adsorption of dye from aqueous solution by cashew nut shell: Studies on equilibrium isotherm, kinetics and thermodynamics of interactions. Desalination, 261(1–2), 52–60. https://doi.org/10.1016/j.desal.2010.05.032
- Li, X., Zhou Yang, L., & Joo-Youp, L. (2013). Adsorption kinetic and equilibrium study for removal of mercuric chloride by CuCl2-impregnated activated carbon sorbent. Journal of Hazardous Materials, 252– 253, 419–427. https://doi.org/10.1016/j.jhazmat.2013.02.049
- Liang, S., Guo, X., Feng, N., & Tian, Q. (2010). Isotherms, kinetics and thermodynamic studies of adsorption of Cu2+ from aqueous solutions by Mg2+/K+ type orange peel adsorbents. Journal of Hazardous Materials, 174(1–3), 756–762. https://doi.org/10.1016/j.jhazmat.2009.09.116
- Naiya, T. K., Chowdhury, P., Bhattacharya, A. K., & Das, S. K. (2009). Saw dust and Neem bark as low-cost natural biosorbent for adsorptive removal of Zn(II) and Cd(II) ions from aqueous solutions. Chemical Engineering Journal, 148(1), 68–79. https://doi.org/10.1016/j.cej.2008.08.002
- Naser, H. A. (2013). Assessment and management of heavy metal pollution in the marine environment of the Arabian Gulf: A review. Marine Pollution Bulletin, 72(1), 6–13. https://doi.org/10.1016/j.marpolbul.2013.04.030
- Nassar, M. M. (2006). Adsorption of Fe+3 and Mn+2 from ground water onto maize cobs using batch adsorber and fixed bed column. Separation Science and Technology, 41(5), 943–959. https://doi.org/10.1080/01496390600588796
- Obayomi, K. S., & Auta, M. (2019). Development of microporous activated Aloji clay for adsorption of Pb(II) from aqueous solution. Heliyon, 5(11), e02799. https://doi.org/10.1016/j.heliyon.2019.e02799
- Obayomi, K. S., Auta, M., & Kovo, A. S. (2020). Isotherm, kinetic and thermodynamic studies for adsorption of lead (II) onto modified Aloji clay. Desalination and Water Treatment, 181, 376–384. https://doi:10.5004/dwt.2020.25142
- Obayomi, K. S, Bello, J. O, Nnoruka, J. S, Adediran, A. A, & Olajide, P. O. (2019). Development of low- cost bio-adsorbent from agricultural waste composite for pb(ii) and as(iii) sorption from aqueous solution. Cogent Engineering, 6(1), 1687274. https://doi.org/10.1080/23311916.2019.1687274
- Okieimen, F. E., Okieimen, C. O., & Wuana, R. A. (2007). Preparation and characterization of activated carbon from rice husks. Journal of Chemical Society, 32, 126–136.
- Olutoye, M. A., & Hameed, B. H. (2013). A highly active clay-based catalyst for the synthesis of fatty acid methyl ester from waste cooking palm oil. Applied Catalysis A: General, 450, 57–62. https://doi.org/10.1016/j.apcata.2012.09.049
- Saka, C., Şahin, Ö., & Küçük, M. M. (2012). Applications on agricultural and forest waste adsorbents for the removal of lead (II) from contaminated waters. International Journal of Environmental Science and Technology, 9(2), 379–394. https://doi.org/10.1007/s13762-012-0041-y
- Sarma, P. J., Kumar, R., & Pakshirajan, K. (2015). Batch and continuous removal of copper and lead from aqueous solution using cheaply available agricultural waste materials. International Journal of Environmental. Resources, 9(2), 635–648. https://ijer.ut.ac.ir/article_938_5057bc945d86bea9b9bdba8d55c6baa8.pdf
- Singha, B., & Das, S. K. (2012). Removal of Pb (II) ions from aqueous solution and industrial effluent using natural biosorbents. Environmental Science and Pollution Research, 19(6), 2212–2226. https://doi.org/10.1007/s11356-011-0725-8
- Tangjuank, S., Insuk, N., Tontrakoon, J., & Udey, V. (2009). Adsorption of lead (II) and cadmium (II) ions from aqueous solutions by adsorption on activated carbon prepared from cashew nutshell. World Academy of Science, Engineering and Technology, 3(4), 1098–1104. https://pdfs.semanticscholar.org/f7ef/126d172934abbedcf98c6cf57c8559fe0a64.pdf
- WHO, World Health Organization. (2015). Guidelines for drinking-water quality (4th ed.). World Health Organization. http://whqlibdoc.who.int/publications/2015/9782415481_eng.pdf
- Wong, K. K, Lee, C. K, Low, K. S, & Haron, M. J. (2003). Removal of cu and pb from electroplating wastewater using tartaric acid modified rice husk. Process Biochemistry, 39(4), 437–445. https://doi.org/10.1016/S0032-9592(03)00094-3
- Yahya, M. D., & Odigure, J. O. (2015). Fixed bed column study for Pb(II) adsorption using calcium-alginate Shea butter husk (TSBH). 5th International Conference on Industrial Engineering and Operations Management.
- Yao, Y, Gao, B, Inyanga, M, Zimmerman, A.R, Cao, X, Pullammanappallil, P, & Yang, L. (2011). Journal of hazardous materials, 190. Removal Of Phosphate from Aqueous Solution by Biochar Derived from Anaerobically Digested Sugar Beet Tailings. https//doi.org/ 10.1016/2011.03.083
- Zhao, G., Wu, X., Tan, X., & Wang, X. (2011). Sorption of heavy metals ions from aqueous solution: A review. Open Colloid Science Journal, 4(1), 19–31. https://doi.org/10.2174/1876530001104010019
- Zhong, W. S., Ren, T., & Zhao, L. J. (2016). Determination of Pb (lead), Cd (cadmium), Cr (chromium), Cu (copper), and Ni (nickel) in Chinese tea with high-resolution continuum source graphite furnace atomic absorption spectrometry. Journal of Food Drug Analysis, 24(1), 46–55. https://doi.org/10.1016/j.jfda.2015.04.010
- Zhou, H., & Smith, D. W. (2002). Advanced technologies in water and wastewater treatment. Journal of Environmental Engineering Science, 1(4), 247–264. https://doi.org/10.1139/s02-020-