Abstract
In the present research, nanocomposites based on copper (I) oxide poly(1-vinyl-2—pyrolidone-co-itaconic acid) nanostructures (Cu2O/p(NVP-co-AI)) were synthesized by the electrodeposition method using the copolymer p(NVP-co-AI) as a stabilizing agent in the reduction of Cu+2 to Cu+. The chemical and physical properties of the nanostructures were characterized by techniques such as scanning electron microscopy, infrared (IR) spectroscopy, Raman and ultraviolet-visible spectrophotometry. The obtained nanostructures are mainly a mixture of agglomerated nanospheres with a diameter of approximately 78 to 91 nm, with an alternation of nanolaminar structures, composed of copper (I) oxide species. In the case of the Cu2O/p(NVP-co-AI) nanocomposite, it was observed a decrease in the carbon-oxygen vibration links of the carbonyl groups in IR intensity for the polymer when it was dissolved in the electrolytic solution, which indicates that interactions are produced by the carbonyl groups with the Cu2O species. In addition, bandgap values of 1.80 and 1.63 eV were estimated by the Kubelka–Munk method for Cu2O and Cu2O/p(NVP-co-AI) samples, respectively.
Public interest statement
This work shows the approaches to prepare of copper oxide/polymer (Cu2O/p(NVP-co-AI)) nanocomposite films by the method of electrodeposition in aqueous solution of Cu2+ using p(NVP-co-AI) as stabilizer. In addition, the chemical and physical properties of the nanostructures were characterized by scanning electron microscopy (SEM), UV-Vis spectrometry, Fourier transformed infrared (FTIR) and Raman spectroscopy. In particular, we analyze the optical properties and how they vary with the different morphologies obtained via stabilization with the polymer.
1. Introduction
Nanostructured oxides have attracted the attention of scientific researchers due to their ease of obtaining and their morphological order at the nanoscale, generating a control in their chemical and physical properties, which gives rise to numerous potential applications (Mutalib et al., Citation2013), such as in areas of medicine, optoelectronics, acoustics, photonics, sensors and catalysis (Alvarado, Citation2017; Lara-juárez et al., Citation2018; Verdaguer, Citation2016). Among the nanostructured oxides, Cu2O is classified as a “p” type semiconductor material due to the copper electron vacancies in the crystalline system, which results in low bandgap values, between 1.9 and 2.17 eV (Osherov et al., Citation2013; Oyarzún et al., Citation2018). These characteristics classify it as a highly photoactive material in the visible region and, therefore, a good candidate for photoelectrochemical and photovoltaic applications (Stepniowski & Misiolk, Citation2018). In addition, it can be used for the design of solar cells, LEDs, and water photolysis due to the fact that the energies of conduction bands and valence are close to the reduction and oxidation potentials of water (Gattinoni & Michaelides, Citation2015; Musselman et al., Citation2017; Stepniowski & Misiolk, Citation2018). It can represent an extremely promising material to be used as a catalyst in the photodegradation of dyes or organic pollutant molecules, being a low-cost catalyst, easy to obtain, non-toxic nature and coming from the metal copper, which is obtained from the minerals that are exhaustively extracted in mining (Osherov et al., Citation2013).
Currently, there are several methods reported in the literature for obtaining Cu2O nanostructures, such as thermal oxidation of metallic copper at high temperatures (Rai, Citation1988), template-assisted growth (Prieto et al., Citation2003), colloidal methods (Wang et al., Citation2010) and by electrochemical techniques of anodization or electrodeposition (Kar et al., Citation2017; Oyarzún et al., Citation2018). However, high temperature processes generally limit the control of the interfacial characteristics of Cu2O thin films, which significantly affects the optical and photoelectrochemical properties of the resulting oxide (Read et al., Citation2009). As an alternative, electrodeposition is a simple, versatile and low-cost method to obtain ordered nanostructures, allowing a better control of their growth (Oyarzún et al., Citation2018). In this context, the appropriate selection of the chelating or stabilizing agent can promote the growth of diverse morphologies, such as wires, bars, points, pores and tubes, among other nanostructures (Allam and Grimes, Citation2011; Kar et al., Citation2017; Read et al., Citation2009). In particular, the use of lactic acid, tartaric acid, ammonium nitrate, amino acids or polymers as chelating agents or stabilizers, have attracted great attention for different technological applications (Oyarzún et al., Citation2018; Zoolfakar et al., Citation2014), due to the improvement that nanostructures present in their physical and optical properties, caused by the incorporation of the chelating agent in the synthesis process (Oyarzún, Pizarro et al., Citation2017; Oyarzún, Tello et al., Citation2017). In this sense, it has been previously reported the synthesis of zinc oxide nanoparticles with poly (N-PhMI-co-HEMA) as stabilizing agent. The function that this polymer fulfills is that zinc is coordinated by poly(N-PhMI-co-HEMA), which allows the speed at which the metal oxidation occurs to be slower, obtaining zinc oxide surfaces with more uniform nanostructured morphologies (Oyarzún, Tello et al., Citation2017). Therefore, it is expected that in similar way the polymer p(NVP-co-AI) coordinates the metal Cu+2 in solution during the electrodeposition process, being the stabilizing agent of this reaction (Oyarzún, Tello et al., Citation2017). Precisely the use of a water-soluble polymeric material as chelating agent during the electrodeposition of Cu2O nanostructures has not been previously reported in scientific literature (Ait et al., Citation2020; Anastasiadou et al., Citation2019; Anower et al., Citation2017; Bao et al., Citation2012; Das et al., Citation2018; Gu et al., Citation2010; Kim et al., Citation2014; Lee et al., Citation2004; Y. Liu et al., Citation2016; R. Liu et al., Citation2003; Messaoudi et al., Citation2016; Nian et al., Citation2009; Paracchino et al., Citation2012; Rahal et al., Citation2018; Ravichandiran et al., Citation2019; Shahbazi & Kiani, Citation2016; Xua et al., Citation2012; Yang et al., Citation2016; Zhao et al., Citation2011; Zhu et al., Citation2013), therefore, the develop this simple, low-cost synthesis method under novel chemical conditions is novel.
In the present work, it is reported the synthesis of Cu2O/p(NVP-co-AI) based nanocomposite films by the method of electrodeposition in aqueous solution of Cu2+ using p(NVP-co-AI) as stabilizer. In addition, the chemical and physical properties of the nanostructures were characterized by scanning electron microscopy (SEM), UV-Vis spectrometry, Fourier transformed infrared (FTIR) and Raman spectroscopy.
2. Materials and methods
The synthesis of p(NVP-co-AI) was carried out by radical radical polymerization at 70°C during 120 min, using benzoyl peroxide as initiator, in addition to the monomers 1-vinyl-2-pyrolidone (NVP) and itaconic acid (AI) in ratios 1:1, 1:3, 3:1, dissolved in distilled water (Pizarro et al., Citation2009).
The electrochemical synthesis was performed in a conventional electrochemical cell containing an Ag/AgCl/KCl(sat) reference electrode, as a counter electrode a spiral shaped platinum wire and as a working electrode a glass plate coated with indium tin-doped oxide (ITO). The working electrode was rinsed with MILLI-Q water and then cleaned with an ultrasound bath. The electrode was immersed in an acetone/ethanol solution in a ratio of 1:1 for 15 min at room temperature to improve film adhesion.
The deposition of Cu2O in the absence of the copolymer was carried out by cyclic voltammetry in the potential range (−0.8; 0) V in an electrolyte composed of 5 mL of aqueous solutions of 0.005 M CuSO4 + 5 mL of 0.05 M NaNO3 (this solution will be called “C”). This electrodeposition range was selected by performing electrodeposition in a range of (−1; 1) V depending on the chemical composition of the electrolyte solution (see , and of the supplemental material).
Figure 1. (A) Photographs of the ITO surface and (B) Cyclic voltamograms obtained in the electrodeposition of the Cu2O/p(NVP-co-AI) nanocomposite, using electrolytic solutions composed by 0.05 M NaNO3 + 0.005 M CuSO4 + the p(NVP-co-AI) copolymer in proportion 1:1, concentration 0%; 0.1% (A and B) and 1.0% (B) at a scanning speed of 0.1 V s−1
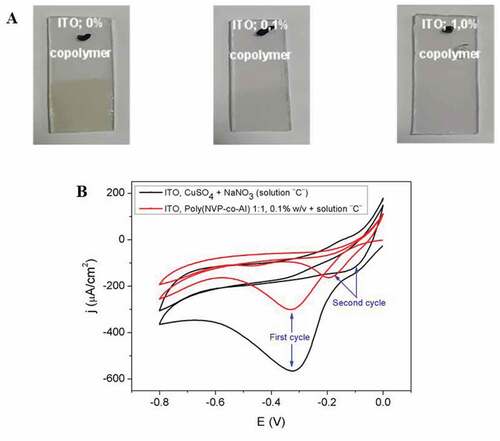
Figure 2. A) Raman spectra of Cu2O nanostructures obtained by electrodeposition in ITO using a concentration “x” of copolymer (1:1). x %w/v: 0.1 (Red) and 0 (Black). B-C) FT-IR spectra for Cu2O/polymer compounds obtained in proportion (1:3) and z %w/v + 0.005 M CuSO4 + 0.05 M NaNO3, z: 0.1 (black); 0.5 (red); 1.0 (blue); 2.0 (green) and without (black) (B), or 0.1 %w/v + 0.005 M CuSO4 + 0.05 M NaNO3 (blue) (C)
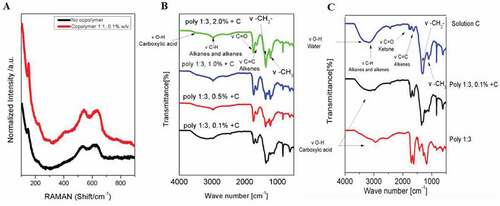
Figure 3. SEM micrographs of the ITO surface with Cu2O/poly (NVP-co-AI) nanocomposite, synthesized with the copolymer in proportion (1:1) and in concentration I: 0.1 % w/v, II: 0.5%w/v, III: 2.0%w/v (IA: Mag 10KX, IB: Mag 20KX, IC: Mag 50KX, ID: Mag 50KX)
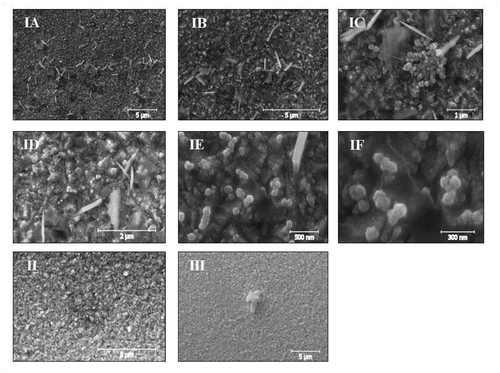
For the preparation of the nanocomposite, the same procedure was followed but in the presence of x % w/v p(NVP-co-AI) (0.1 < x < 2), using copolymer ratios of 1:1, 3:1, 1:3.
Potential and current were monitored on an Autolab 100 potentiostat/galvanostat. The surface morphology of the nanocomposite electrodes was characterized with scanning electron microscopy (SEM), using a Zeiss EVO MA10 model microscope, and the analysis of the micrographs obtained was performed with Gwyddion imaging software 2.53, the characteristic band vibrations were characterized by attenuated total infrared spectroscopy (ATR-FTIR), using an FT-IR spectrometer, Spectrum Two, (UATR Two), PerkinElmer. Raman spectroscopy was carried out using a Horiba LabRAM HR spectrometer and employing a He/Ne laser (wavelength 632.8 nm). While in Uv-Vis spectroscopy a LAMBDA 35, PerkinElmer, spectrometer was used.
3. Results and discussion
shows pictures of the ITO surface after electrodeposition. It can be seen that the surfaces of the ITO are covered with a brown film, which decreases in opacity as the concentration of the copolymer increases. Also, due to the higher concentration of the copolymer, the electrodeposited oxide film is expected to be thinner than that obtained in a synthesis without the copolymer (Niveditha et al., Citation2016; Oyarzún, Pizarro et al., Citation2017; Oyarzún, Tello et al., Citation2017; Pizarro et al., Citation2009; Riveros et al., Citation2012; Talib et al., Citation2016). If we look at the curve representing the synthesis of nanostructures without copolymer (black line, ), in the first electrodeposition cycle, the signal at −0.3 V corresponds to the reduction of Cu2+ to Cu+, this is much more intense than those obtained in the synthesis with copolymer (red line). This indicates that the polymer material decreases its electrodeposition speed at the ITO surface. Furthermore, in the absence of polymer, a higher current is obtained for the second cycle, which indicates that the ITO surface is more conductive than the film deposited in the presence of the copolymer in the first cycle. While when the polymer is present, a decrease in current intensity is caused during the second cycle, indicating that the ITO surface is partially covered by oxide (Niveditha et al., Citation2016; Riveros et al., Citation2012).
The chemical composition of the nanostructure was analyzed by Raman and ATR-FTIR spectroscopies. shows the Raman spectra for the films obtained by electrodeposition in the presence and absence of p(NVP-co-AI) copolymer. The appearance of the signals at 149 cm−1, 542 cm−1 and 623 cm−1, and the absence of the bands at 289 cm−1, 296 cm−1 and 345 cm−1, show the presence mainly of copper (I) oxide species on the ITO surfaces (Oyarzún, López et al., Citation2017).
When analyzing the ATR-FTIR spectra (see ), a weak band at approximately 3000 cm−1 is observed in each spectrum, which is characteristic of C-H bending—type vibrations for alkanes. In addition, a very wide band between 2400 cm−1 and 3600 cm−1 is observed, which is characteristic of stress type vibrations of O-H bonds for the carboxylic acid functional group from the AI monomer. A very wide band between 1725 and 1705 cm−1 is also observed which corresponds to a stress type vibration of the C = O bonds of the ketone functional groups and carboxylic acid of the NVP and AI monomers. Between 1680 and 1620 cm−1 another strong band is observed, corresponding to a C = C stretching type vibration of the alkene functional group. Finally, two medium bands are observed at 1450 and 1375 cm−1, which are characteristic for bending vibrations of—CH2—and—CH3 respectively. When analyzing the difference in intensity of each band obtained, only differences are obtained between the bands at 1725–1705 cm−1 and 1680–1620 cm−1 corresponding to ketones (C = O), carboxylic acid and alkenes (C = C), respectively. As the concentration of copolymers increases, the intensity of these two bands is greater, although for the C = O band the intensity increases significantly more, due to the greater presence of this functional group, since a greater amount of asymmetric vibrations of the carbon-oxygen bond is produced, which is represented in the spectrum with a more intense band (Oyarzún, Pizarro et al., Citation2017; Oyarzún, Tello et al., Citation2017; Pizarro et al., Citation2009).
On the other hand, when only the synthesis with solution “C” is carried out (see ), the bands corresponding to ketones, carboxylic acid and alkenes are absent at 1725–1705 cm−1 and 1680–1620 cm−1, respectively, since there is no presence in the electrolytic solution of the copolymer, which is what provides this type of vibration. If we compare the corresponding band of ketones or carboxylic acid at 1705 cm−1 we observe that in the copolymer without copper sulfate solution the band is very intense, while in the copolymer + “C” solution the intensity of the band decreases. This is because the oxygen atoms are coordinated with the Cu+2 ions in solution, causing a decrease in the amount of C = O vibrations that were initially present (Oyarzún, Pizarro et al., Citation2017; Oyarzún, Tello et al., Citation2017; Pizarro et al., Citation2009).
By performing the electrodeposition in the presence of p(NVP-co-AI) copolymer at 0.1 %w/v (1:1), nanostructures were obtained along the surface of the ITO in the form of nanospheres, accompanied by nanosheets ( “IA” and “IB”). In addition, a uniform morphology is observed with a higher concentration of nanospheres above the nanosheets, which are deposited at random on the surface of the substrate (see “ID”). By observing the SEM micrographs with the highest magnification, it was determined that the nanoshells tend to agglomerate with each other, while the nanoshells are deposited on the surface individually ( “IC” and “ID”). Finally, it was determined that the average diameter of the nanoshells ranges from 78 nm to 91 nm ( “IE” and “IF”).
SEM micrographies of the nanocomposite obtained in the syntheses with 0.5% w/v and 2% w/v copolymer (1:1), nanoscales were obtained. The sample synthesized with 2% w/v copolymer presents a more uniform morphology, but the copolymer tends to agglomerate on the surface of the ITO more than the one obtained with 0.5% w/v copolymer ( II and III) (Niveditha et al., Citation2016; Oyarzún et al., Citation2018; Oyarzún, Tello et al., Citation2017).
The influence of p(NVP-co-AI) on the semiconductor properties was studied by UV-Vis spectrometry (see ). The absorbance spectrum of Cu2O nanostructures shows a weak absorption below 300 nm (black curve) compared to Cu2O/p(NVP-co-AI) nanosheets, which also shows an absorption only below 300 nm but significantly more intense. In addition, it can be determined by observing the UV-Vis spectrum that both samples were completely transparent films in the visible region. In the direct transition semiconductors as Cu2O it is possible to make an estimation of the band gap by the Kubelka–Munk method. It is plotting (-Ln(T) * hv)2 against the photon energy (hv), being T the % of transmittance of the sample, h the Planck constant, v the frequency of the energy and then extrapolating the linear adjustment of the highest energy to (Ln (T) = 0) () (Meyer et al., Citation2012). With this method, bandgap values of 1.63 eV for Cu2O (black line) and 1.80 eV for Cu2O/p(NVP-co-AI) (red line) were estimated. The values obtained for this material are much lower than those previously reported for various types of Cu2O nanostructures obtained by electrodeposition (1.92–2.47 eV), using various substrates, chelating agents and chemical composition of the electrolytic solution (see ). This corroborates that the use of soluble polymeric materials have not been previously studied to stabilize the electrodeposition reaction of Cu2O nanostructures, besides that in these conditions the semiconductor properties of Cu2O nanostructures are considerably benefited, generating a material with potential applications (Ait et al., Citation2020; Anastasiadou et al., Citation2019; Anower et al., Citation2017; Bao et al., Citation2012; Das et al., Citation2018; Gu et al., Citation2010; Kim et al., Citation2014; Lee et al., Citation2004; Y. Liu et al., Citation2016; R. Liu et al., Citation2003; Messaoudi et al., Citation2016; Nian et al., Citation2009; Paracchino et al., Citation2012; Rahal et al., Citation2018; Ravichandiran et al., Citation2019; Shahbazi & Kiani, Citation2016; Xua et al., Citation2012; Yang et al., Citation2016; Zhao et al., Citation2011; Zhu et al., Citation2013). The band separation value obtained in the presence of the copolymer is higher than without it, probably due to the presence of the polymeric material adhered to the Cu2O nanostructures, which increases the energy between the conduction and valence bands of the semiconductor, generating a higher quantum confinement of the nanostructures (Tello et al., Citation2012).
Figure 4. UV-Vis absorbance spectrum (A), energy band gap (B) of Cu2O nanoparticles (black line) and Cu2O/Poly (NVP-co-AI) nanocomposite (red line)
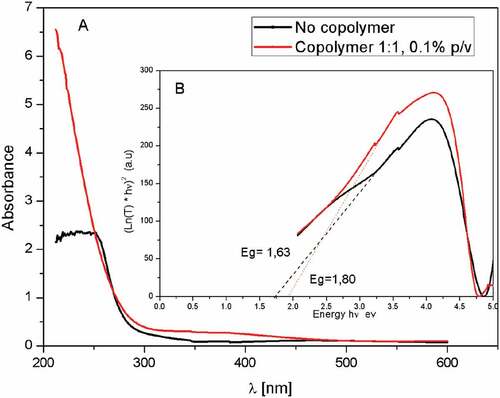
Table 1. Chelating agent type, electrolyte, substrate and bandgap values of films with Cu2O nanostructures obtained by electrodeposition
4. Conclusions
The results of this research show that it is possible to obtain Cu2O nanostructures in ITO by electrodeposition in the potential range of 0 V to −0.8 V from an aqueous solution of Cu2+ in the presence of p(NVP-co-AI). The morphology obtained shows a uniform film, composed of a mixture of agglomerated nano-grains with a diameter of approximately 78 nm to 91 nm and an alternation of nanoscales for a polymer concentration of 0.1% w/v. In addition, similar nanostructures are obtained by increasing the polymer concentration, where the surface uniformity increases, but in turn the polymer material is agglomerated on the surface of the ITO.
From the ATR-FTIR spectra obtained, it is confirmed that Cu2+ is effectively coordinated with the oxygen atoms of the carboxylic and carbonyl groups of the polymer. Besides, the decrease of the bandgap of Cu2O nanostructures obtained in comparison to those previously reported using the same synthesis technique indicates that the growth of Cu2O nanostructures takes place inside the polymer, which causes an important improvement in the semiconductor properties of Cu2O.
Acknowledgements
The authors thank to Master Program in Chemistry mention of Materials Technology of the Metropolitan Technological University, Internal Project (L217-15) and Fondecyt project n° 1191336. OELP thanks the financial support from SECYT-UNC. RAMAN equipment at LANN, INFIQC-UNC/CONICET, Sistema Nacional de Microscopía- MINCyT, Argentina, are gratefully acknowledged.
Additional information
Funding
Notes on contributors
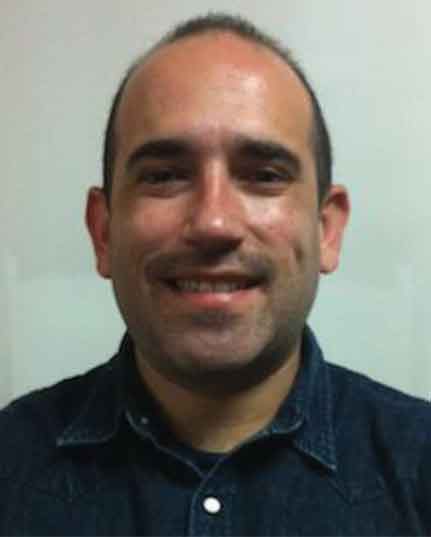
Diego P. Oyarzún
Diego P. Oyarzún Jerez obtained his PhD. in Chemistry (2014) from Pontifical Catholic University of Valparaíso (Valparaíso, Chile). He did a postdoctoral training at the Center of Applied Nanosciences of Faculty of Exacts Sciences of Andrés Bello University (Santiago, Chile) and in the Department of Physical Chemistry, Faculty of Chemical Sciences Córdoba National University (Córdoba, Argentina) focusing his research on the synthesis and characterization of polymers materials and anodic metal oxide films. Currently, he is professor and researcher at the Laboratory of Applied Nanosciences of Atacama University (Copiapó, Chile). Dr. Oyarzún Jerez research interests are focused on the preparation, characterization and different applications of nanocomposite materials based on anodic metal oxide and polymeric matrix. This research provides new synthesis conditions of a fundamental component to generate these new nanocomposites with potential applications in areas such as photoelectrochemistry, photovoltaics, solar cells, LEDs, water photolysis and photocatalysis.
References
- Ait, A., Atourki, L., Labchir, N., Abouabassi, K., Ouafi, M., Mouhib, H., Ihlal, A., Elfanaoui, A., Benmokhtar, S., & Bouabid, K. (2020). Structural and optical properties of electrodeposited Cu2O thin films. Mater. Today, 22(1), 89–10. https://doi.org/10.1016/j.matpr.2019.08.100
- Allam, N., & Grimes, C. (2011). Electrochemical fabrication of complex copper oxide nanoarchitectures via copper anodization in aqueous and non-aqueous electrolytes. Mater. Lett, 65(12), 1949–1955. https://doi.org/10.1016/j.matlet.2011.03.105
- Alvarado, J. (2017). Analysis of the perovskite structure LaxSr1-xCryMn1-yO3-δ with potential application as an anode for solid oxide fuel cells. Bol. Soc. Esp. Ceram, 56(2), 73–82. https://doi.org/10.1016/j.bsecv.2016.09.003
- Anastasiadou, D., Schellekens, M., de Heer, M., Verma, S., & Negro, E. (2019). Electrodeposited Cu2 O films on gas diffusion layers for selective CO2 electroreduction to ethylene in an alkaline flow electrolyzer. ChemElectroChem, 6(15), 3928–3932. https://doi.org/10.1002/celc.201900971
- Anower, M., Al-Gaashani, R., Hamoudi, H., Al Marri, M., Hussein, I., Belaidi, A., Merzougui, B., Alharbi, F., & Tabet, N. (2017). Controlled growth of Cu2O thin films by electrodeposition approach. Mat. Sci. Semicon. Proc, 63(1), 203–211. https://doi.org/10.1016/j.mssp.2017.02.012
- Bao, M., Wang, D., Liu, S., Kuang, L., Sun, J., Wang, F., & Wen, Y. (2012). Electrodeposition and electrocatalytic activity of Cu2O film on stainless steel substrate. Appl. Surf. Sci, 258(20), 8008–8014. https://doi.org/10.1016/j.apsusc.2012.04.156
- Das, C., Singh, A., Heo, Y., Aggarwal, G., Maurya, S., Seidel, J., & Kavaipatti, B. (2018). Effect of grain boundary cross-section on the performance of electrodeposited Cu 2 O photocathodes. The Journal of Physical Chemistry C, 122(3), 1466–1476. https://doi.org/10.1021/acs.jpcc.7b10103
- Gattinoni, C., Michaelides, A. (2015). Atomistic details of oxide surfaces and surface oxidation: The example of copper and its oxides. Surf. Sci. Rep, 70(3), 424-447. https://doi.org/10.1016/j.surfrep.2015.07.001
- Gu, Y., Su, X., Du, Y., & Wang, C. (2010). Preparation of flower-like Cu2O nanoparticles by pulse electrodeposition and their electrocatalytic application. Appl. Surf. Sci, 256(20), 5862–5866. https://doi.org/10.1016/j.apsusc.2010.03.065
- Kar, P., El-tahlawy, M., & Zhang, Y. (2017). Anodic copper oxide nanowire and nanopore arrays with mixed phase content: Synthesis, characterization and optical limiting response. J. Phys. Commun, 1(4), 1–9. https://doi.org/10.1088/2399/6528/aa93a4
- Kim, T., Oh, H., Ryu, H., & Lee, W. (2014). The study of post annealing effect on Cu2O thin-films by electrochemical deposition for photoelectrochemical applications. J. Alloys Compd, 612(1), 74–79. https://doi.org/10.1016/j.jallcom.2014.05.158.
- Lara-juárez, D., García-contreras, R., & Concepción, M. (2018). Sutures functionalised with nanomaterials for oral surgery. A systematic review. Rev. Esp. Cir. Oral Maxilofac, 40(1), 33–40. https://doi.org/10.1016/j.maxilo.2017.01.001
- Lee, Y., Leu, I., Chang, S., Liao, C., & Fung, K. (2004). The electrochemical capacities and cycle retention of electrochemically deposited Cu2O thin film toward lithium. Electrochimica Acta, 50(2-3), 4553–4559. https://doi.org/10.1016/j.electacta.2003.12.072
- Liu, R., Oba, F., Bohannan, E., Ernst, F., & Switzer, J. (2003). Shape control in epitaxial electrodeposition: Cu 2 O nanocubes on InP(001). Chem. Mater, 15(26), 4882–4885. https://doi.org/10.1021/cm034807c
- Liu, Y., Liu, Y., Mu, R., Yang, H., Shao, C., Zhang, J., Lu, Y., Shen, D., & Fan, X. (2016). The structural and optical properties of Cu2O films electrodeposited on different substrates. Semicond. Sci. Technol, 20(1), 44–49. https://doi.org/10.1088/0268-1242/20/1/007
- Messaoudi, O., Ben Assaker, I., Gannouni, M., Souissi, A., Makhlouf, H., Bardaoui, A., & Chtourou, R. (2016). Structural, morphological and electrical characteristics of electrodeposited Cu2O Effect of deposition time. Appl. Surf. Sci. 366(1) 383–388. https://doi.org/10.1016/j.apsusc.2016.01.035.
- Meyer, B., Polity, A., Reppin, D., Becker, M., Hering, P., Klar, P. J., Sander, T. H., Reindl, C., Benz, J., Eickhoff, M., Heiliger, C., Heinemann, M., Blasing, J., Krost, A., Shokovets, S., Muller, C., & Ronning, C. (2012). Binary copper oxide semiconductors: From materials towards devices. Phys. Status. Solid. B, 249(8), 1487–1509. https://doi.org/10.1002/pssb.201248128
- Musselman, K., Wisnet, A., Iza, D., Hesse, H., Scheu, C., MacManus-Driscoll, J., & Schmidt-Mende, L. (2017). Strong efficiency improvements in ultra‐low‐cost inorganic nanowire solar cells. Adv. Mater, 22(35), 254–258. https://doi.org/10.1002/adma.201001455
- Mutalib, A., Losic, D., & Voelcker, N. H. (2013). Nanoporous anodic aluminium oxide: Advances in surface engineering and emerging applications. Prog. Mater. Sci, 58(5), 636–704. https://doi.org/10.1016/j.pmatsci.2013.01.002
- Nian, J., Tsai, C., Lin, P., & Teng, H. (2009). Elucidating the conductivity-type transition mechanism of p-type Cu[sub 2]O films from electrodeposition. J. Electrochem. Soc, 156(7), H567–H573. https://doi.org/10.1149/1.3125800
- Niveditha, C., Fatima, M., & Sindhu, S. (2016). Comprehensive interfacial study of potentio-dynamically synthesized copper oxide thin films for photoelectrochemical applications. J. Electrochem. Soc, 163(6), 426–433. https://doi.org/10.1149/2.0971606jes
- Osherov, A., Zhu, C., & Panzer, M. (2013). Role of solution chemistry in determining the morphology and photoconductivity of electrodeposited cuprous oxide films. Chem. Mater, 25(5), 692–698. https://doi.org/10.1021/cm303287g
- Oyarzún, D., Broens, M., Linarez, O., López, M., Islas, R., & Arratia-Perez, R. (2018). Simple and rapid one-step electrochemical synthesis of nanogranular Cu2O films. ChemistrySelect, 3(30), 8610–8614. https://doi.org/10.1002/slct.201703128
- Oyarzún, D., López, M., Ramos, W., Pérez, O. L., Sánchez, J., Pizarro, G., Acosta, G., Flores, M., & Arratia-Perez, R. (2017). Nanostructuring of anodic copper oxides in fluoride-containing ethylene glycol media. J. Electroanal. Chem, 807(1), 181–186. https://doi.org/10.1016/j.jelechem.2017.11.047
- Oyarzún, D., Pizarro, G., Asenjo, A., Tello, A., Martin-Trasanco, R., Zúñiga, C., Sánchez, J., & Arratia-Pérez, R. (2017). Synthesis and morphological characterization of nanocomposite based on anodic tio2 nanotubes and poly(n-maleoyl glycine-co-acrylic acid). J. Chil. Chem. Soc, 62(3), 3634–3636. https://dx.doi.org/10.4067/s0717-97072017000303634
- Oyarzún, D., Tello, A., Pizarro, G., Martin-Transaco, R., Zúñiga, C., Perez-Donoso, J., & Arratia-Perez, R. (2017). Electrochemical synthesis, optical properties and morphological characterization of ZnO/Poly(N-PhMI-co-HEMA) nanocomposite. J. Electroanal. Chem, 799(1), 358–362. https://doi.org/10.1016/j.jelechem.2017.06.039
- Paracchino, A., Brauer, J., Moser, J., Thimsen, E., & Graetzel, M. (2012). Synthesis and characterization of high-photoactivity electrodeposited Cu2O solar absorber by Photoelectrochemistry and Ultrafast Spectroscopy. The Journal of Physical Chemistry C, 116(3), 7341–7350. https://doi.org/10.1021/jp301176y
- Pizarro, G., Marambio, O., Jeria-Orell, M., Oyarzún, D., Rivas, B., & Habicher, W. (2009). Synthesis and characterization of hydrophilic copolymers of maleimide derivatives with 2-hydroxyethyl methacrylate: Electrochemical and thermal behavior. Polym. Int, 58(10), 1160–1166. https://doi.org/10.1002/pi.2644
- Prieto, A., Martín-González, M., Keyani, J., Gronsky, R., Sands, T., & Stacy, A. (2003). The electrodeposition of high-density, ordered arrays of Bi1xSbxNanowires. J. Am. Chem. Soc, 125(9), 2388–2389. https://doi.org/10.1021/ja029394f
- Rahal, H., Kihal, R., Affoune, A., & Rahal, S. (2018). Electrodeposition and characterization of Cu2O thin films using sodium thiosulfate as an additive for photovoltaic solar cells. J. Chem. Eng, 26(2), 421–427. https://doi.org/10.1016/j.cjche.2017.06.023
- Rai, B. (1988). Cu2O solar cells: A review. Sol. Cells, 25(3), 265–272. https://doi.org/10.1016/0379-6787(88)90065-8
- Ravichandiran, C., Sakthivelu, A., Davidprabu, R., Valanarasu, S., Kathalingam, A., Ganesh, V., Shkir, M., Algarni, H., & AlFaify, S. (2019). In-depth study on structural, optical, photoluminescence and electrical properties of electrodeposited Cu2O thin films for optoelectronics: An effect of solution pH. Microelectron. Eng, 210(1), 27–34. https://doi.org/10.1016/j.mee.2019.03.013
- Read, C., Steinmiller, E., & Choi, K. (2009). Atomic plane-selective deposition of gold nanoparticles on metal oxide crystals exploiting preferential adsorption of additives. J. Am. Chem. Soc, 131(34), 12040–12041. https://doi.org/10.1021/ja9036884
- Riveros, G., Garmendia, A., Ramírez, D., Tejos, M., Grez, P., Gómez, H., & Dalchielec, E. A. (2012). Study of the electrodeposition of Cu2O thin films from DMSO solution. J. Electrochem. Soc, 160(1), 28–33. https://doi.org/10.1149/2.029302jes
- Shahbazi, P., & Kiani, A. (2016). Fabricated Cu2O porous foam using electrodeposition and thermal oxidation as a photocatalyst under visible light toward hydrogen evolution from water. Int. J. Hydrog. Energy, 41(39), 17247–17256. https://doi.org/10.1016/j.ijhydene.2016.07.080
- Stepniowski, W., & Misiolk, W. (2018). Review of fabrication methods, physical properties, and applications of nanostructured copper oxides formed via electrochemical oxidation. Nanomaterials, 8(6), 379–398. https://doi.org/10.3390/nano8060379
- Talib, R., Abdullah, M., Al-salman, H., Mohammad, S., & Allam, N. (2016). ZnO nanorods/polyaniline-based inorganic/organic heterojunctions for enhanced light sensing applications. J. Solid State Sci. Tech, 5(3), 142–147. https://doi.org/10.1149/2.0031603jss
- Tello, A., Gómez, H., Muñoz, E., Riveros, G., Pereyra, C., Dalchiele, E., & Marotti, R. E. (2012). Electrodeposition of nanostructured ZnO thin films from dimethylsulfoxide solution: Effect of temperatures on the morphological and optical properties. J. Electrochem. Soc, 159(12), 750–755. https://doi.org/10.1149/2.017301jes
- Verdaguer, J. (2016). The specific immunotherapy of autoimmune diseases through the nanomedicine. Endocrinol Y Nutr, 63(9), 437–439. https://doi.org/10.1016/j.endonu.2016.05.003
- Wang, D., Peng, Q., & Li, Y. (2010). Nanocrystalline intermetallics and alloys. Nano. Res, 3(8), 574–580. https://doi.org/10.1007/s12274-010-0018-4
- Xua, L., Xua, H., Wua, S., & Zhang, X. (2012). Synergy effect over electrodeposited submicron Cu2O films in photocatalytic degradation of methylene blue. Appl. Surf. Sci, 258(11), 4934–4938. https://doi.org/10.1016/j.apsusc.2012.01.122
- Yang, Y., Li, Y., & Pritzker, M. (2016). Control of Cu2O film morphology using potentiostatic pulsed electrodeposition. Electrochim. Acta, 213(1), 225–235. https://doi.org/10.1016/j.electacta.2016.07.116
- Zhao, W., Fu, W., Yang, H., Tian, C., Li, M., Li, Y., Zhang, L., Sui, Y., Zhou, X., Chen, H., Zou, G. (2011). Electrodeposition of Cu2O film and their photoelectrochemical properties. CrystEngComm, 13(8), 2871–2877. https://doi.org/10.1039/C0CE00829J
- Zhu, C., Osherov, A., & Panzer, M. (2013). Surface chemistry of electrodeposited Cu2O films studied by XPS. Electrochimica Acta, 111(1), 771–778. https://doi.org/10.1016/j.electacta.2013.08.038
- Zoolfakar, A., Rani, R., Morfa, A., O’Mullane, A., & Kalantar-zadeh, K. (2014). Nanostructured copper oxide semiconductors: A perspective on materials, synthesis methods and applications. J. Mater. Chem, 27(2), 5247–5270. https://doi.org/10.1039/C4TC00345D