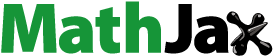
Abstract
Removal of cephalexin residues from wastewater is one of the most concerned issues in treatment plants due to the growing of the antibiotics consumption level around the world. In this study, the impact of the initial concentration of cephalexin residues on its removability by lemna minor was investigated by developing a grau model accounting for the cephalexin initial pH value. In the model, the second-order kinetic constants of adsorption were linked linearly with the influent pH values. Results showed that using lemna minor along with phytoremediation process increased the removal efficiency value to 86.5% and dropped chemical oxygen demand (COD) to 0.012 mg/L in effluent, making the removability of cephalexin from the polluted water easier. In addition, it was found that changing the grau model kinetic constants linearly (positively or negatively) with pH affects the removal efficiency. Based on the results, the best removability can be noticed at the pH level of 7 with a value of 71%.
PUBLIC INTEREST STATEMENT
Cephalexin residue is considered as one of the pollutants that can accumulate in wetland system due to the untreated effluent from typical wastewater treatment plants. This residue has been a concern in wastewater treatment technology because of its removal difficultly by the traditional treatment. In this work, the grau model based on the adsorption mechanisms was modified and simulated in order to improve the removal efficiency of cephalexin residue by using lemna minor technique. Using the proposed treatment helps researchers to explain the essential mechanisms of transformation, degradation, and mineralization of cephalexin residue in wastewater, increasing the residue removal efficiency by lemna minor.
1. Introduction
Cephalexin is antibiotics that are therapeutic organic compounds produced by particular microorganisms as secondary metabolites or generated in artificial or semi-artificial ways (Diyanati et al., Citation2013; Phillips, Citation2003). Antibiotics exert antibacterial activity by altering the basic metabolic bacterial paths (Alquzweeni & Alkizwini, Citation2020; Girardi et al., Citation2011). These antibiotics are commonly used in human drugs to prevent the bacterial disease, in the agricultural field to fight the pathogens, and in the veterinary industry and in aquaculture to control of pathogenic agents. Antibiotics, however, are also applied to promote growth (Dyanati-Tilaki et al., Citation2013; Naji et al., Citation2020a; Nunes et al., Citation2019). The wide use of medical products has contributed in increasing their concentrations in the ecosystem. Low levels of these compounds existed, but nowadays they are widespread in the aquatic environments particularly. Typically, these compounds reach the environment by releasing them from prescription drugs in feces and urine. Eventually, cephalexin residues enter wastewater with or without treatment (Balarak & Mostafapour, Citation2016; Nunes et al., Citation2019). In certain countries, wastewater, which contains cephalexin metabolic waste is processed at wastewater treatment facilities since the residues could be removed by absorption in the sludge treatment (Faisal et al., Citation2020f; Naji et al., Citation2020a; Rahi et al., Citation2020; Saad et al., Citation2019). However, in the absence of remediation procedures or when the treatment is not efficient, these cephalexin residues are accumulated directly into water bodies (Alshammari et al., Citation2020; Karthikeyan & Meyer, Citation2006).
The incomplete removal of pollutants in wastewater is an issue (Alkizwini, Citation2021; Pawęska et al., Citation2017). This downside poses a potential danger to aquatic species that live in water bodies. Many wastewater treatment technology, including coagulation, floatation, oxidation, chemical treatment, photochemical degradation, and microbial treatment have been developed to solve such an issue by eliminating antibiotics from habitats (Ahmed et al., Citation2020b; Abd Ali et al., Citation2020; Mahvi et al., Citation2018; Naji et al., Citation2020c). The application of plants in this treatment has grown over the years in the biological remediation of pollutants. Many plant species with the ability to eliminate a wide range of environmental pollutants have been reported (Ahmed et al., Citation2020a; Naji et al., Citation2020c; Turk Sekulic et al., Citation2019).
Phytoremediation was suggested as an efficient, cheap, and eco-friendly method for sites with a accepted level of pollutant concentration (Alquzweeni et al., Citation2021; Türker & Yakar, Citation2017). Plants may engage directly in detoxification processes through the absorption of pollutants and posterior metabolizing or immobilizing inside the plant, or in an indirect way through the promoting of rhizospheric microorganisms, which are efficiently detoxified. Phytofiltration is one of those processes. Mainly phytofiltration requires the use of floating, submerged or developed aquatic plants to extract contaminants from the solution, predominantly through their root system, while fronds are also engaged in a direct way (Alquzweeni et al., Citation2021; Olguín & Sánchez-Galván, Citation2012). Because of their action as “nutrient pumps”, this technique has a particular significance in the treatment of wastewater. Taking this role of phytoremediation into account, it can be argued that using aquatic macrophyte (e.g., lemna minor) in some remediation processes has great importance, particularly in the final step of tertiary treatment where fully purified wastewater is disposed into the environment. Ultimately, these plants that are utilized in phytoremediation processes could be either disposed to landfills or incinerated. In addition, the incineration process has been suggested as a method to downsize the plants and to produce the thermal energy (Moreira et al., Citation2020). Furthermore, plants can be applied in industries of ceramic, integrating the biomass applied in the remediation of ceramic blocks. The ability of plants such as lemna minor to absorb and metabolize significant quantities of pharmaceuticals under realistic conditions, similar to what happens in environment, has been reported and approved (J.-L. Zhao et al., Citation2010a; Murchie & Niyogi, Citation2011; Nunes et al., Citation2019; L. Zhao et al., Citation2010b). However, there is a lack of toxicological studies that are evaluating the performance of plants at the biochemical level after the exposure to toxicants.
These photosystems have very effective light-harvesting processes, simultaneously preventing photosynthetic and photo-oxidative damage. This may occur during periods of intense light, and photosynthetic organisms develope different defensive mechanisms for adaption purposes (Faisal et al., Citation2020d; Jahns & Holzwarth, Citation2012). There are two primary paths for chloroplasts: (a) Electron transference to molecular oxygen, producing O2, H2O2, and OH (Halliwell, Citation2007); (b) Energy transfer from a triplet chlorophyll to molecular oxygen form, resulting in the production of the radical superoxide (Nunes et al., Citation2019). However, these processes could not be used to evaluate the risks on the environment because they do not accurately measure the toxic impacts of pollutant on the aquatic ecosystems (Zeng et al., Citation2009). Hence, toxicity and concentration of antibiotic need to be taken into account analytically to understand the quality of wastewater. This can lead to accurate indices to the toxic effects of wastewaters on the aquatic environments. Appropriate assessment methods must be used for determining the effectiveness of the applied wastewater treatment. In this regard, the effluent quality has been focused by monitoring the physical, chemical, and biochemical properties in addition to the identifying particular pollutants (Petrović et al., Citation2005). Therefore, this paper aims to investigate the toxicity of cephalexin on lemna minor for different cephalexin concentrations and pH level by developing a grau model to simulate the governing adsorption processes.
2. Materials and methods
2.1. The experimental work
Lemna minor was obtained from laboratorial cultures and kept under well-controlled conditions (temperatures 25 ± 2 °C). The cultures adaption to the new environment was performed by keeping the plant in a continuous lit place for 5 days to allow plant acclimatization to the conditions required by the approved test guidelines. The lemna minor was subsequently exposed to a set of three cephalexin concentrations for five days. Standard stock solutions were prepared by dissolving 10 mg of cephalexin in water and storing in amber glass vials with a cap for a period of 7 days at 20 °C (Faisal et al., Citation2020b) and in a dark place. The chosen concentrations were 0.01, 0.05, 0.1, and 0.15 mg/L with a purity percentage of 99.7%. For the period of 6 weeks (Jun/1/2020 to September/15/2020), the operation phase was based on a constructed wetland (CW) as batch reactors for different concentrations. The batch tests were performed with a detention period equivalent to 5 days. Samples were collected and tested at the Ministry of Science and Technology, Iraq to determine cephalexin concentrations by using High-Performance Liquid Chromatography (HPLC) test (Argekar et al., Citation1997).
Furthermore, a Hach-Lang commercial kit (2,125,915-COD HR) was utilized to measure the COD in this study according to the technique 410.4 of US EPA (USEPA Iris, Citation1993) in addition to use a spectrophotometer (model HI-83,214) to measure the concentrations of COD (mg/L).
2.2. Kinetic modeling
The operation of the constructed wetland was very complex due to the occurrence of biological, chemical, and physical processes during the same time to eliminate the contaminants from wastewater. Thus, a unique mathematical representation of predominant processes was done to show the treatment method influence in improving the wetlands water quality. Although different models have been developed, the majority of CW designs remains is based on the researcher’s knowledge (DWA-A 262, 2006) or the simple first-order decay models. However, the first-order model is insufficient for designing constructed wetlands. Various models were utilized to design CW. Some models comprise first-order degradation kinetics, others forecast that the pollutant concentrations to be remediated will become zero when the detention time goes toward infinity, which is not always the case in CW.
The kinetic model is considered a beneficial tool to understand the biological and basic transference mechanisms within the reactor. Constants that are calculated from the kinetic formula are named bio-kinetic coefficients or constants of growth (Kröger et al., Citation2007). These kinetic constants characterize and expect the system performance. Bio-kinetic constants are based on the conditions of the environment and kind of microbial species such as temperature, pH, dissolved oxygen, substances of inhibition, nutrients, and organic matters decay in wastewater. Kinetic modelling has been utilized in a simple shape, and few variables are included for easy monitoring and applying in industrial purposes and for simple defining the coefficients of kinetic. The literature showed several mathematical models that define the processes occurred with systems identical to CW, such as the first-order model, the grau second-order model, Sundstrom model, the Stover-Kincannon model, the Chen model, the Contois model, and the Michaelis-Menten type kinetic model (Basibuyuk & Kalat, Citation2004). The grau second-order model was applied in this study to simulate the removability of antibiotic at different conditions.
The grau model has a second-order kinetic reaction, which can be expressed as follows: (Andreo-Martínez et al., Citation2016; Giraldi & Iannelli, Citation2009):
Where is the influent contaminants concentrations (mg/L),
represents the effluent contaminants concentrations (mg/L),
(X is the concentrations of the biomasses in the reactor; and k2 is the second-order contaminant removal rate constant (per day), and b is a constant greater than one.
This equation was simplified to become:
The kinetic constants () and (b) were calculated by fitting EquationEq. 2
(2)
(2) with the experimental measurements using s nonlinear regression. Statistical analysis was performed by using the one-way analysis of variance (ANOVA), and the differences between the measured and the predicted values were evaluated by using the t-test with an acceptable significance level (P-value of less than 0.05) (Al-Zubaidi et al., Citation2021).
To incorporate the influent pH effect, the kinetic constants will be plotted against pH to display the constants as a function of pH level. Eventually, EquationEq. (2)(2)
(2) can be expressed in terms of pH level.
3. Results and discussion
3.1. Modeling pH effects on the grau model
shows the experiment results that were determined at pH values from 3 to 10. Each set of results was correlated with pH values fitted to the grau model (EquationEq. (2(2)
(2) )), and the constants were predicted by nonlinear regression in SPSS Statistics version 20 (). Clearly, the values of a and b change with the initial pH of the influent solutions.
Table 1. Values of kinetic constants () and (b) as a function with the initial pH of the feed solution
To clarify the relationship between the constants (a and b) and the pH values, the constants were fitted with pH values as shown in . The values of a and b reveal a linear relationship as the pH level increases toward a value of 7. After that, the constants increase a linearly with the pH level. Because of the protonations and deprotonations of the acidic and alkaline groups of the root, cephalexin adsorptions behavior is affected by the value of pH that in turn influenced the surface structures of the root. This can be attributed to the presence of various cephalexin species at various values of pH. Cephalexin is a zwitterionic molecule with pKa values of 2.56 and 6.88 (Legnoverde et al., Citation2013; Naji et al., Citation2020a) . It was found that a charge of anion was existed on cephalexin at a pH value of 6.88 and above, while at pH values below 2.56, a charge of cation was existed as zwitterion species between both pKa values. Hence, the electrostatic attraction between cephalexin, and the surface of the root is preferable at the pH values. This indicated that the growth or reduction in the pH values from its best value (7) lead to a decrease in the values of a and b for surface sites. This may occur because of the competition between the protons and cations to uptake the binding sites when pH values are low. These linear relationships result in the following general linear models:
Figure 2. Effects of initial pH value on the constants (a and b) predicted by the grau model for cephalexin removability (Standard Deviation = 0.1)
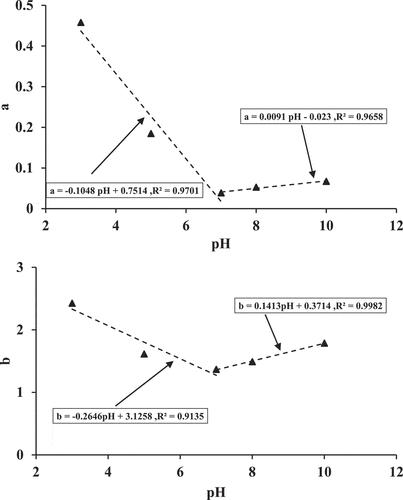
Where Ɣ1,Ɣ2,Ɣ3 and, Ɣ4 are constants in which the linear relationship is negative for the pH values of less than 7 and positive for the pH values of greater than 7.
Substituting EquationEq. (3)(3)
(3) and (Equation4
(4)
(4) ) in EquationEq. (2)
(2)
(2) yields:
EquationEquation (5)(5)
(5) is a wide applicable formula that could be utilized to express the initial concentration variation at various pH values, see .
3.2. Cephalexin initial concentration effect
The removal efficiencies based on different initial concentrations (0.01, 0.05, 0.1, and 0.15) mg/L and detention periods (1–5) day in the lemna minor CW are shown in . For initial concentration of 0.01 mg/L, the removal efficiency values were 84.66, 85.36, 85.76, 85.96, and 86.46 (Jun/2020) and 71.16, 71.96, 72.36, 72.46, and 72.96 (July/2020) for the last period during the detention periods. These values decreased as the initial concentration increases from 0.05 to 0.15 mg/L. For instance, the removal efficiency decreased from 86.46 (corresponding to initial concentration of 0.01 and detention period of 5 days on Jun) to 61.16 (corresponding to initial concentration of 0.15 and detention period of 5 days on the same month). Similar behavior exists during the other months. The results indicated that the content of the cephalexin medium continues to increase in the plant. High dosages of cephalexin in duckweed reduce the probability of forming the metabolites (starch, protein, polypeptices, etc.), which can lead to excessive amounts of biomass in such installations. A few previous studies reported physiological changes and restraining paths of enzymes in plants that are subjected to high cephalexin concentration, indicating to the adverse effect of high cephalexin content on the plant functionality (Białk-Bielińska et al., Citation2018). Thus, the content in the duckweed-depended phytoremediation system has to be optimized for the best operation efficiency (Faisal et al., Citation2020a; Verma & Suthar, Citation2015). In addition, the results confirmed that the presence of lemna minor would increase the reduction of cephalexin by adopting suitable initial concentrations since lemna minor will provide the required environment for phytoremediation growth within the root zone and other parts of lemna minor (Kouki et al., Citation2009). Light-harvesting and energy transfer in the plant, the photosystem is responsible for the active core (Baker, Citation2008; Faisal et al., Citation2020c).
Figure 4. Removal efficiencies of cephalexin with a constant initial concentration of 0.01 mg/L in the CW
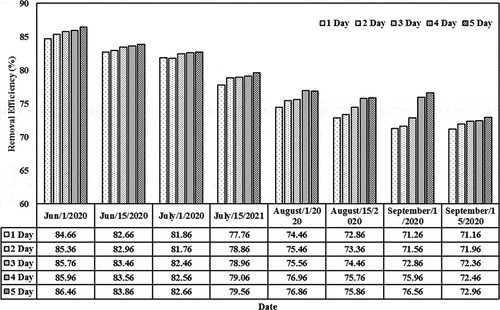
Figure 5. Removal efficiencies of cephalexin with a constant initial concentration of 0.05 mg/L in the CW
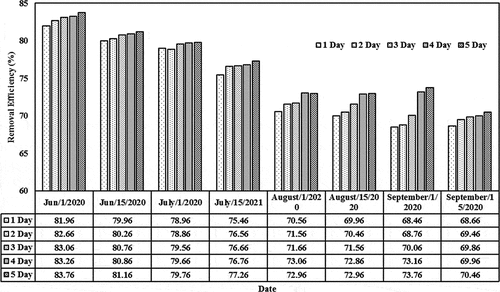
Figure 6. Removal efficiencies of cephalexin with a constant initial concentration of 0.1 mg/L in the CW
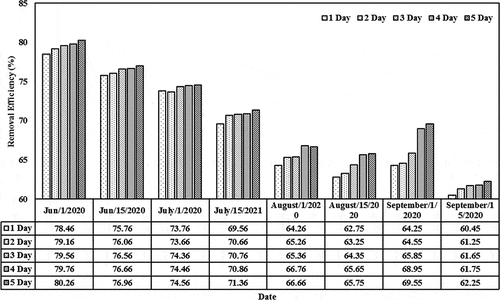
Figure 7. Removal efficiencies of cephalexin with a constant initial concentration of 0.15 mg/L in the CW
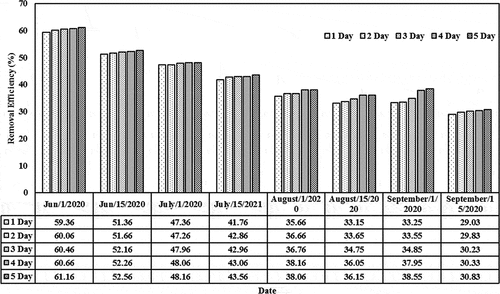
In plants, radical superoxide (O−2) is naturally generated during photosynthesis in chloroplasts, especially when plants develop under conditions of environmental stress. This prevents the photosynthetic processes and leads to absorb the light excessively (Faisal et al., Citation2020e; Nunes et al., Citation2019). Cephalexin can interact with the chain of electron transport, resulting in the production of oxidized chlorophyll triplets that directly transmit the energy to the oxygen molecules, which in turn have a double impact on the plant cells, oxidizing the biomolecules including lipids (structural components of the cellular membrane) (Faisal & Naji, Citation2019; Ramel et al., Citation2012). However, it can ultimately impact the normal physiological properties of plants. Catalase is the primary enzyme that degrades the hydrogen peroxide, and it protects the organisms from the deleterious impacts of oxidation that can be caused by chemicals (Nunes et al., Citation2015). The permanent activity of catalase in cells makes it able to prevent the damages of H2O2 during the natural aerobic process, which is one of the physiological functions of cells. However, the amounts of hydrogen peroxide may grow due to the presence of oxidative stress (following the metabolism process of some chemical compounds). H2O2 growth is directly followed by increasing activities of catalase, that is considered as a physiological reparation during the conditions of oxidative stresses (Nunes et al., Citation2019). Gomes et al. (Citation2017) revealed that when lemna minor plant was subjected to ciprofloxacin concentration equals to 0.75 mg/ L for a week, it showed remarkable growth in the concentration of peroxide and activity of catalase.
3.3. Catalase determination (CAT)
Due to the Catalase importance in preventing the oxidative damages which occur because of the accumulating species of reactive oxygen, catalase determination (CAT) has been selected as a biochemical marker enzyme. Catalase could compromise the physiological properties of plant (Gill & Tuteja, Citation2010). A 96-well microplate was used for determining the activity of CAT. The previously described microplate reader was employed to perform spectrophotometric readings. Basing on the rate of degradation of the substrate H2O2, the activity was quantified and monitored at 240 nm for 5 minutes. The outcomes have been represented based on the consideration of the activity of CAT equivalents to the degraded amounts of hydrogen peroxide per minute per milligram of proteins. Proteins quantity was determined using the Bradford technique at 595 nm (Bradford, Citation1976), adapted to micro-plate with bovine γ-globulin as a standard, for representing the enzymatic activity per milligram of proteins on the analyzed sample.
shows the Catalase activities of lemna minor subjected to effluents and Steinberg’s solution (control) for a period of 5 days. The determined quantities of CAT activity were equal to the lemna minor used plants in phytoremediation process. It was clear that the enzymatic activity of the plants diminished noticeably before and after the remediation process (p < 0.05). The occurrence of chemicals and the chemical exposing of biota may result in an environmental stress as the metabolism of these chemicals produces oxygen derivatives with high instability named reactive oxygen species (ROS). When these species accumulate in the plants’ cells, it results in serious oxidative damage, which inhibits the growing and yielding of the plants (Caverzan et al., Citation2016), and for shielding purposes against the harmful influence of ROS, the plant develops antioxidant mechanism (Racchi, Citation2013). One of the most important strategies to avoid the oxidative damage is to enhance the CAT activity, an enzyme which effectively induces the transfer of H2O2 to water and oxygen (Valko et al., Citation2006). Based on the present results, the activity of this enzyme declines when the exposure occurs after the remediation process, establishing the pro-oxidative scenarios. These patterns have been highlighted in many studies (de Alkimin et al., Citation2020). Singh et al. (Citation2008) demonstrated that the activities of CAT grew in lemna minor when it was subjected to various concentrations of industrial effluents, which are highly loaded with metals for a period of 7 days. Radić et al. (Citation2010) noticed that after subjecting lemna minor to industrial effluents for a week, the amounts of peroxidase activity (POD) grew significantly and an engagement was observed between the plant defense mechanisms and its enzymatic forms. In general, there is a possibility to activate the antioxidant defense mechanism of plant which is connected to oxidative stress that happens after the subjecting process to effluents of industry; nevertheless, the patterns of species response relay on their permissibility levels, pollutants concentration, and the stage of the plant growth (Gill & Tuteja, Citation2010).
3.4. Chemical oxygen demand (COD) role
shows COD values before and after the treatment compared to controls. it can be noticed that COD concentrations in controls were almost equal to 0.05 mg/L, while higher concentrations can be observed in the raw effluent. On the other hand, COD in effluent dropped to 0.012 mg/L generally after the phytoremediation process. Lemna minor is capable of reducing the organic matter load in effluent remarkably. This reduction was very significant because the COD level in the effluent of post-treatment was the same COD level in the control treatment. Additionally, both effluents before the phytoremediation process have small concentrations of COD, and therefore they would not need further remediation. Regarding this, it is crucial to emphasize that the remediation using lemna minor could diminish these concentrations even further, and this is a phytoremediation process advantage since these remediated effluents will reduce the detrimental impacts on the environment after discharging process. For the untreated forms, organic wastes are mineralized after discharging to the surface water and produce nutritive elements that induce plants growth, resulting in the eutrophication phenomena (Kanu & Achie, Citation2011).
This excess formation of organic content results in the formation of “sludge”, and the dissolved oxygen is consumed from the water due to the mineralization process, which is the main cause for fish death (Kanu & Achie, Citation2011). Therefore, lemna minor can be considered as a highly effective plant that could be applied in phytoremediation to enhance the quality of effluents of the tertiary treatment and to obtain further purification since a significant decrease of COD concentration was attended as shown in . Additionally, it is important to clear that the decrease of COD concentration by the remediation was high, where efficiencies of removability were about 75%. These variances can be attributed to the nature of the organic matters that are found in each effluent, which is a vital parameter for the degradation. The impacts of cephalexin toxicity on the aquatic environment are attributed to many factors; such as, it lasts in the environment for prolonged periods since it accumulates in biota and in sediments, pollutants decay into mutagenic compounds, and lack of aerobic biodegradation.
4. Conclusions
Treatment of cephalexin residue in wetlands using the typical approaches has a limited influence on the residue removal efficiency. In this study, a grau model was developed for simulating the pH impact of cephalexin wastes on the removal efficiency by using lemna minor. Lemna minor was manufactured in lab to be used for cephalexin removal. By simulating the model, the second-order kinetic constants of adsorption were linked to pH values of the wastes influent, highlighting the relationship between them. The model explained the complicated processes and the essential mechanisms of transformation, degradation, and mineralization of cephalexin residue by using lemna minor. Results showed that positive linear relationship exists between grau model kinetic constants and the pH level of the cephalexin initial concentration when the pH values are greater than 7, and the relationship becomes negative when the pH values are less than 7. Based on the model results, the cephalexin removal efficiency can be improved by varying the initial concentration of cephalexin in the wastes. In addition, lemna minor helps decrease COD level in the effluent significantly.
Additional information
Funding
Notes on contributors
Hussien A. M. Al-Zubaidi
Hussein A. M. Al-Zubaidi is Assist. Prof. Dr. and the Head of Environmental Engineering Department at the Faculty of Engineering, University of Babylon, Iraq. He received his B.Sc. degree in Civil Engineering from the College of Engineering, University of Babylon, Iraq and M.Sc. degree in Environmental Engineering from the same College in 2003 and 2007, respectively. The author finished his Ph.D. degree in Civil and Environmental Engineering at Portland State University, Oregon, USA in 2018 under the supervision of Prof. Dr. Scott A. Wells. He has worked as a research assistant at the Department of Environmental Engineering, University of Babylon since 2005 and has become the department head since 2020. His research interests are working on environmental modelling (analytical, statistical, and numerical methods), water and wastewater treatment, and environmental engineering. Also, Hussein has published more than 20 papers in reputed journals and has been serving as a reviewer for different reputable journals.
References
- Abd Ali, Z. T., Naji, L. A., Almuktar, S. A. A. A. N., Faisal, A. A. H., Abed, S. N., Scholz, M., Naushad, M., & Ahamad, T. (2020). Predominant mechanisms for the removal of nickel metal ion from aqueous solution using cement kiln dust. Journal of Water Process Engineering, 33, 101033. https://doi.org/10.1016/j.jwpe.2019.101033
- Ahmed, D. N., Faisal, A. A. H., Jassam, S. H., Naji, L. A., & Naushad, M. (2020a). Kinetic model for pH variation resulted from interaction of aqueous solution contaminated with nickel ions and cement kiln dust. Journal of Chemistry, 2020, 1–16. https://doi.org/10.1155/2020/8732308
- Ahmed, D. N., Naji, L. A., Faisal, A. A. H., Al-Ansari, N., & Naushad, M. (2020b). Waste foundry sand/MgFe-layered double hydroxides composite material for efficient removal of Congo red dye from aqueous solution. Scientific Reports, 10(1), 2042. https://doi.org/10.1038/s41598-020-58866-y
- Alkimin, G. D., Paisio, C., Agostini, E., & Nunes, B. (2020). Phytoremediation processes of domestic and textile effluents: Evaluation of the efficacy and toxicological effects in Lemna minor and Daphnia magna. Environmental Science and Pollution Research, 27(4), 4423–4441. https://doi.org/10.1007/s11356-019-07098-3
- Alkizwini, R. S. (2021). The use of an organo-kaolinite sorbent in a permeable reactive barrier for remediating groundwater contaminated with methylene blue dye: Experimental and theoretical investigation. Environmental Processes, 8(2), 889–910. https://doi.org/10.1007/s40710-021-00515-1
- Alquzweeni, S., Alkizwini, R., & Hassan, A. (2021). A novel application of building demolition waste for removal benzene from aqueous solutions. Przeglad Naukowy Inzynieria I Ksztaltowanie Srodowiska, 30(1), 86–97. https://doi.org/10.22630/PNIKS.2021.30.1.8
- Alquzweeni, S. S., & Alkizwini, R. S. (2020). Removal of cadmium from contaminated water using coated chicken bones with double-layer hydroxide (Mg/Fe-LDH). Water, 12(8), 2303. https://doi.org/10.3390/w12082303
- Alshammari, M., Al Juboury, M. F., Naji, L. A., Faisal, A. A. H., Zhu, H., Al-Ansari, N., & Naushad, M. (2020). Synthesis of a novel composite sorbent coated with siderite nanoparticles and its application for remediation of water contaminated with congo red dye. International Journal of Environmental Research, 14(2), 177–191. https://doi.org/10.1007/s41742-020-00245-6
- Al-Zubaidi, H. A., Naje, A. S., Abed Al-Ridah, Z., Chabuck, A., & Ali, M. A. (2021). A statistical technique for modelling dissolved oxygen in salt lakes. Cogent Engineering, 8(1). https://doi.org/10.1080/23311916.2021.1875533
- Andreo-Martínez, P., García-Martínez, N., & Almela, L. (2016). Domestic wastewater depuration using a horizontal subsurface flow constructed wetland and theoretical surface optimization: A case study under dry mediterranean climate. Water, 8(10), 434. https://doi.org/10.3390/w8100434
- Argekar, A. P., Raj, S. V., & Kapadia, S. U. (1997). Simultaneous determination of cephalexin and carbocisteine from capsules by reverse phase high performance liquid chromatography (RP - HPLC). Analytical Letters, 30(4), 821–831. https://doi.org/10.1080/00032719708006427
- Baker, N. R. (2008). Chlorophyll fluorescence: A probe of photosynthesis in vivo. Annual Review of Plant Biology, 59(1), 89–113. https://doi.org/10.1146/annurev.arplant.59.032607.092759
- Balarak, D., & Mostafapour, H. A. K. F. (2016). Study of the adsorption mechanisms of cephalexin on to azolla filiculoides. 8(10), 114-121. https://www.derpharmachemica.com/abstract/study-of-the-adsorption-mechanisms-of-cephalexin-on-to-azolla-filiculoides-10379.html
- Basibuyuk, M., & Kalat, D. G. (2004). The use of waterworks sludge for the treatment of vegetable oil refinery industry wastewater. Environmental Technology (United Kingdom), 25(3), 373–380. https://doi.org/10.1080/09593330409355471
- Białk-Bielińska, A., Matzke, M., Caban, M., Stolte, S., Kumirska, J., & Stepnowski, P. (2018). Effects of five sulphonamides on duckweed (Lemna minor) after prolonged exposure time and their dependency on photoradiation. Science of the Total Environment, 618, 952–960. https://doi.org/10.1016/j.scitotenv.2017.08.286
- Bradford, M. M. (1976). A rapid and sensitive method for the quantitation of microgram quantities of protein utilizing the principle of protein-dye binding. Analytical Biochemistry, 72(1–2), 248–254. https://doi.org/10.1016/0003-2697(76)90527-3
- Caverzan, A., Casassola, A., & Patussi Brammer, S., (2016) Reactive oxygen species and antioxidant enzymes involved in plant tolerance to stress. in Abiotic and Biotic Stress in Plants - Recent Advances and Future Perspectives, InTech.
- Diyanati, R., Cherati, J. Y., & Belarak, D. (2013). Effect of sorbitol on phenol removal rate by lemna minor. J-Mazand-Univ-Med-Sci, 22(2), 58-65. http://jmums.mazums.ac.ir/article-1-2030-en.html
- Dyanati-Tilaki, R.-A., Yousefi, Z., Yazdani-Cherati, J., & Balarak, D. (2013). The ability of azollaand lemna minor biomassfor adsorption of phenol fromaqueous solutions. Journal of Mazandaran University of Medical Sciences, 23(106), 141-6. https://www.researchgate.net/publication/284042707_The_Ability_of_Azollaand_Lemna_Minor_Biomassfor_Adsorption_of_Phenol_Fromaqueous_Solutions
- Faisal, A. A. H., Ali, I. M., Naji, L. A., Madhloom, H. M., & Al-Ansari, N. (2020b). Using different materials as permeable reactive barrier for remediation of groundwater contaminated with landfill’s leachate. Desalination and Water Treatment, 175, 152–163. https://doi.org/10.5004/dwt.2020.24890
- Faisal, A. A. H., Alquzweeni, S. S., Naji, L. A., & Naushad, M. (2020c). Predominant mechanisms in the treatment of wastewater due to interaction of benzaldehyde and iron slag byproduct. International Journal of Environmental Research and Public Health, 17(1), 226. https://doi.org/10.3390/ijerph17010226
- Faisal, A. A. H., Al-Wakel, S. F. A., Assi, H. A., Naji, L. A., & Naushad, M. (2020a). Waterworks sludge-filter sand permeable reactive barrier for removal of toxic lead ions from contaminated groundwater. Journal of Water Process Engineering, 33, 101112. https://doi.org/10.1016/j.jwpe.2019.101112
- Faisal, A. A. H., Ibreesam, M. M., Al-Ansari, N., Naji, L. A., Naushad, M., & Ahamad, T. (2020d). COMSOL multiphysics 3.5a package for simulating the cadmium transport in the sand bed-bentonite low permeable barrier. Journal of King Saud University - Science, 32(3), 1944–1952. https://doi.org/10.1016/j.jksus.2020.01.047
- Faisal, A. A. H., Jasim, H. K., Naji, L. A., Naushad, M., & Ahamad, T. (2020e). Cement kiln dust-sand permeable reactive barrier for remediation of groundwater contaminated with dissolved benzene. Separation Science and Technology, 1–14.
- Faisal, A. A. H., & Naji, L. A. (2019). Simulation of ammonia nitrogen removal from simulated wastewater by sorption onto waste foundry sand using artificial neural network. Association of Arab Universities Journal of Engineering Sciences, 26(1), 28–34. https://doi.org/10.33261/jaaru.2019.26.1.004
- Faisal, A. A. H., Nassir, Z. S., Naji, L. A., Naushad, M., & Ahamad, T. (2020f). A sustainable approach to utilize olive pips for the sorption of lead ions: Numerical modeling with aid of artificial neural network. Sustainable Chemistry and Pharmacy, 15, 100220. https://doi.org/10.1016/j.scp.2020.100220
- Gill, S. S., & Tuteja, N. (2010). Reactive oxygen species and antioxidant machinery in abiotic stress tolerance in crop plants. Plant Physiology and Biochemistry, 48(12), 909-30. https://doi.org/10.1016/j.plaphy.2010.08.016
- Giraldi, D., & Iannelli, R. (2009). Measurements of water content distribution in vertical subsurface flow constructed wetlands using a capacitance probe: Benefits and limitations. Desalination, 243(1–3), 182–194. https://doi.org/10.1016/j.desal.2008.05.012
- Girardi, C., Greve, J., Lamshöft, M., Fetzer, I., Miltner, A., Schäffer, A., & Kästner, M. (2011). Biodegradation of ciprofloxacin in water and soil and its effects on the microbial communities. Journal of Hazardous Materials, 198, 22–30. https://doi.org/10.1016/j.jhazmat.2011.10.004
- Gomes, M. P., Gonçalves, C. A., de Brito, J. C. M., Souza, A. M., Da Silva Cruz, F. V., Bicalho, E. M., Figueredo, C. C., & Garcia, Q. S. (2017). Ciprofloxacin induces oxidative stress in duckweed (Lemna minor L.): Implications for energy metabolism and antibiotic-uptake ability. Journal of Hazardous Materials, 328, 140–149. https://doi.org/10.1016/j.jhazmat.2017.01.005
- Halliwell, B. (2007). Biochemistry of oxidative stress. Biochemical Society Transactions, 35(5), 1147–1150. https://doi.org/10.1042/BST0351147
- Iris, U. S. E. P. A., (1993) Bromodichloromethane (CASRN 75-27-4). Integrated Risk Information System.
- Jahns, P., & Holzwarth, A. R. (2012). The role of the xanthophyll cycle and of lutein in photoprotection of photosystem II. Biochimica et Biophysica Acta (BBA) - Bioenergetics, 1817(1), 182–193. https://doi.org/10.1016/j.bbabio.2011.04.012
- Kanu, I., & Achie, O. K. (2011). Industrial effluents and their impact on water quality of receiving rivers in Nigeria. Journal of Applieal Technology in Environmental Sanitation, 1(1), 75-86.
- Karthikeyan, K. G., & Meyer, M. T. (2006). Occurrence of antibiotics in wastewater treatment facilities in Wisconsin, USA. Science of the Total Environment, 361(1–3), 196–207. https://doi.org/10.1016/j.scitotenv.2005.06.030
- Kouki, S., M’hiri, F., Saidi, N., Belaïd, S., & Hassen, A. (2009). Performances of a constructed wetland treating domestic wastewaters during a macrophytes life cycle. Desalination, 246(1–3), 452–467. https://doi.org/10.1016/j.desal.2008.03.067
- Kröger, R., Holland, M. M., Moore, M. T., & Cooper, C. M. (2007). Plant senescence: A mechanism for nutrient release in temperate agricultural wetlands. Environmental Pollution, 146(1), 114–119. https://doi.org/10.1016/j.envpol.2006.06.005
- Legnoverde, M., Jiménez-Morales, I., Jimenez-Morales, A., Rodriguez- Castellon, E., & Basaldella, E. (2013). Modified silica matrices for controlled release of cephalexin. Medicinal Chemistry, 9(5), 672–680. https://doi.org/10.2174/1573406411309050006
- Mahvi, A. H., Mostafapour, F. K., & Balarak, D. (2018). Biosorption of tetracycline from aqueous solution by Azolla Filiculoides: Equilibrium kinetic and thermodynamics studies. Fresenius Environmental Bulletin, 27(8), 5759-5767. https://www.cabdirect.org/cabdirect/abstract/20193150282
- Moreira, V. R., Lebron, Y. A. R., Da Silva, M. M., de Souza Santos, L. V., Jacob, R. S., de Vasconcelos, C. K. B., & Viana, M. M. (2020). Graphene oxide in the remediation of norfloxacin from aqueous matrix: Simultaneous adsorption and degradation process. Environmental Science and Pollution Research, 27(27), 34513–34528. https://doi.org/10.1007/s11356-020-09656-6
- Murchie, E. H., & Niyogi, K. K. (2011). Manipulation of Photoprotection to Improve Plant Photosynthesis. Plant Physiology, 155(1), 86–92. https://doi.org/10.1104/pp.110.168831
- Naji, L. A., Faisal, A. A. H., Rashid, H. M., Naushad, M., & Ahamad, T. (2020a). Environmental remediation of synthetic leachate produced from sanitary landfills using low-cost composite sorbent. Environmental Technology and Innovation, 18(1), 100680. https://doi.org/10.1016/j.eti.2020.100680
- Naji, L. A., Jassam, S. H., Yaseen, M. J., Faisal, A. A. H., & Al-Ansari, N. (2020c). Modification of Langmuir model for simulating initial pH and temperature effects on sorption process. Separation Science and Technology (Philadelphia), 55(15), 2729–2736. https://doi.org/10.1080/01496395.2019.1655055
- Nunes, B., Veiga, V., Frankenbach, S., Serôdio, J., & Pinto, G. (2019). Evaluation of physiological changes induced by the fluoroquinolone antibiotic ciprofloxacin in the freshwater macrophyte species Lemna minor and Lemna gibba. Environmental Toxicology and Pharmacology, 72, 103242. https://doi.org/10.1016/j.etap.2019.103242
- Nunes, B., Verde, M. F., & Soares, A. M. V. M. (2015). Biochemical effects of the pharmaceutical drug paracetamol on Anguilla anguilla. Environmental Science and Pollution Research, 22(15), 11574–11584. https://doi.org/10.1007/s11356-015-4329-6
- Olguín, E. J., & Sánchez-Galván, G. (2012). Heavy metal removal in phytofiltration and phycoremediation: The need to differentiate between bioadsorption and bioaccumulation. New Biotechnology, 30(1), 3–8. https://doi.org/10.1016/j.nbt.2012.05.020
- Pawęska, K., Bawiec, A., & Pulikowski, K. (2017). Wastewater treatment in submerged aerated biofilter under condition of high ammonium concentration. Ecological Chemistry and Engineering S, 24(3), 431–442. https://doi.org/10.1515/eces-2017-0029
- Petrović, M., Hernando, M. D., Díaz-Cruz, M. S., & Barceló, D. (2005). Liquid chromatography–tandem mass spectrometry for the analysis of pharmaceutical residues in environmental samples: A review. Journal of Chromatography. A, 1067(1–2), 1–14. https://doi.org/10.1016/j.chroma.2004.10.110
- Phillips, I. (2003). Does the use of antibiotics in food animals pose a risk to human health? A critical review of published data. Journal of Antimicrobial Chemotherapy, 53(1), 28–52. https://doi.org/10.1093/jac/dkg483
- Racchi, M. (2013). Antioxidant Defenses in Plants with Attention to Prunus and Citrus spp. Antioxidants, 2(4), 340–369. https://doi.org/10.3390/antiox2040340
- Radić, S., Stipaničev, D., Cvjetko, P., Mikelić, I. L., Rajčić, M. M., Širac, S., Pevalek-Kozlina, B., & Pavlica, M. (2010). Ecotoxicological assessment of industrial effluent using duckweed (Lemna minor L.) as a test organism. Ecotoxicology, 19(1), 216–222. https://doi.org/10.1007/s10646-009-0408-0
- Rahi, M. A., Faisal, A. A. H., Naji, L. A., Almuktar, S. A., Abed, S. N., & Scholz, M. (2020). Biochemical performance modelling of non-vegetated and vegetated vertical subsurface-flow constructed wetlands treating municipal wastewater in hot and dry climate. Journal of Water Process Engineering, 33, 101003. https://doi.org/10.1016/j.jwpe.2019.101003
- Ramel, F., Birtic, S., Ginies, C., Soubigou-Taconnat, L., Triantaphylides, C., & Havaux, M. (2012). Carotenoid oxidation products are stress signals that mediate gene responses to singlet oxygen in plants. Proceedings of the National Academy of Sciences, 109(14), 5535–5540. https://doi.org/10.1073/pnas.1115982109
- Saad, N., Abd Ali, Z. T., Naji, L. A., Faisal, A. A. A. H., & Al-Ansari, N. (2019). Development of Bi-Langmuir model on the sorption of cadmium onto waste foundry sand: Effects of initial pH and temperature. Environmental Engineering Research, 25(5), 677–684. https://doi.org/10.4491/eer.2019.277
- Singh, K., Misra, A., & Pandey, S. N. (2008). Responses of Lemna minor L. (duckweed) plants to the pollutants in industrial waste water. Research in Environment and Life Sciences, 1(1), 5-8. https://www.researchgate.net/publication/221658359_Responses_of_Lemna_minor_L_duckweed_plants_to_the_pollutants_in_industrial_waste_water
- Turk Sekulic, M., Boskovic, N., Slavkovic, A., Garunovic, J., Kolakovic, S., & Pap, S. (2019). Surface functionalised adsorbent for emerging pharmaceutical removal: Adsorption performance and mechanisms. Process Safety and Environmental Protection, 125, 50–63. https://doi.org/10.1016/j.psep.2019.03.007
- Türker, O. C., & Yakar, A. (2017). A hybrid constructed wetland combined with microbial fuel cell for boron (B) removal and bioelectric production. Ecological Engineering, 102, 411–421. https://doi.org/10.1016/j.ecoleng.2017.02.034
- Valko, M., Rhodes, C. J., Moncol, J., Izakovic, M., & Mazur, M. (2006). Free radicals, metals and antioxidants in oxidative stress-induced cancer. Chemico-Biological Interactions, 160(1), 1–40. https://doi.org/10.1016/j.cbi.2005.12.009
- Verma, R., & Suthar, S. (2015). Lead and cadmium removal from water using duckweed – Lemna gibba L.: Impact of pH and initial metal load. Alexandria Engineering Journal, 54(4), 1297–1304. https://doi.org/10.1016/j.aej.2015.09.014
- Zeng, D. H., Hu, Y. L., Chang, S. X., & Fan, Z. P. (2009). Land cover change effects on soil chemical and biological properties after planting Mongolian pine (Pinus sylvestris var. mongolica) in sandy lands in Keerqin, northeastern China. Plant and Soil, 317(1–2), 121–133. https://doi.org/10.1007/s11104-008-9793-z
- Zhao, J.-L., Ying, -G.-G., Liu, Y.-S., Chen, F., Yang, J.-F., Wang, L., Yang, X.-B., Stauber, J. L., & Warne, M. S. J. (2010a). Occurrence and a screening-level risk assessment of human pharmaceuticals in the Pearl River system, South China. Environmental Toxicology and Chemistry, n/a–n/a. https://doi.org/10.1002/etc.161
- Zhao, L., Dong, Y. H., & Wang, H. (2010b). Residues of veterinary antibiotics in manures from feedlot livestock in eight provinces of China. Science of the Total Environment, 408(5), 1069–1075. https://doi.org/10.1016/j.scitotenv.2009.11.014