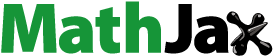
Abstract
Austempered ductile iron is an innovative material obtained by subjecting it to an austempering heat treatment. The wide application of this material is because its properties are comparable to those of steel, which has a much lower weight. However, the machinability of this material is always a difficult task owing to its high hardness. This problem becomes more complex when a considerable amount of Mn is added. In this study, a novel two-step austempering heat treatment was performed on spheroidal graphite iron containing different amounts of manganese. Although the novel heat treatment method helps to obtain a superior combination of hardness and impact, its effect on machinability must be determined. This study provides the results of machinability tests carried out on austempered ductile iron with various manganese contents produced using the novel method. Scanning electron microscope images of the microstructures revealed a typical ausferrite structure with no segregation of manganese at the grain boundary. This is evident from the good tool life obtained in the machinability tests. Regression equations are fitted to determine the tool life and surface roughness for machining parameters with a range of values considered in this study. The results obtained have shown that till 1 wt% of manganese alloyed austempered ductile iron can be successfully produced using the novel heat treatment method. This helps to obtain the optimum combination of strength and impact properties.
1. Introduction
Spheroidal graphite (SG) iron is a type of cast iron, which is also known as ductile iron, and has better ductility than other types of cast iron. The better ductility is due to the presence of graphite in the spheroid shape, unlike white and grey cast iron, in which graphite exists as a flake. Hence, the ductile iron name has been established because of the good ductility of the cast iron family (Artola et al., Citation2017; Nan et al., Citation2020; Zhang et al., Citation2014; Zimba et al., Citation2003). However, the strength and hardness were not as high as those of ductile iron. Conventional austempering heat treatment involves heating the material to the austenetization temperature and then cooling it in a salt bath furnace to obtain an ausferritic microstructure. Owing to this typical structure, the material gains hardness with a slight reduction in ductility. Although this conventional austempering treatment is widely used to impart good mechanical properties to ductile iron, the beneficial properties are obtained at the expense of ductility (Dakre et al., Citation2017; Francucci et al., Citation2008; Hsu & Chuang, Citation2001; Putatunda, Citation2001). To overcome this, a novel two-step austempering treatment was designed. This heat treatment involved rapid quenching to a lower temperature for a short duration before quenching in a salt bath furnace, which was maintained at a higher austempering temperature. This supercooling of the specimen for a short duration is a novel way of improving properties compared to the conventional austempering method which does not involve any supercooling. This allows finer ferrite to be obtained along with a higher amount of retained austenite with a high carbon content. This novel heat treatment resulted in an increase in the hardness without any reduction in ductility. This is a great advantage compared to conventional austempering treatment. It is well-known that the addition of alloying elements to Austempered Ductile Iron (ADI) greatly affects its mechanical properties. The addition of Mn is particularly difficult considering the possible difficulties in machining the material. The wide range of applications of the ADI is well-known in various sectors, from agriculture to automobiles. With these concepts, it is important to develop an ADI with a good combination of mechanical properties and machinability.
Few reports are available regarding the machinability of ADI produced using conventional heat treatment methods. Bayati and Elliot (Bayati & Elliot, Citation1995) reported the difficulty in machining the 0.6 wt% alloyed ADI produced using the conventional method. This study reported the high strength and low ductility obtained in the ADI. In another study, in which machining tests were conducted at different austempering temperatures, machinability was obtained at a higher austempering temperature of 400 °C (Cemal Cakir & Isik, Citation2008). Few other reports are available regarding the possible effects of other alloying elements on the mechanical properties of ADI. Very few studies have reported a two-step austempering method. Elsayed et al. (Citation2009) reported the mechanical properties of Ni and Mo alloyed ADI produced using both conventional and two-step austempering processes. However, the addition of these alloying elements considerably reduces the strength and hardness.
In one of the previous studies by the author, conventional austempering heat treatment was carried out on manganese alloyed ductile iron and the machinability tests were conducted on the same (Hegde & Sharma, Citation2018). This study highlights possible improvements in the mechanical properties of manganese alloyed ADI produced by a different heat treatment method.
Hence, it is clear that limited reports are available regarding the ability of ADI to be machined, especially when it is alloyed with manganese, which is produced using a novel two-step method.
This study aims to determine how the heat treatment and machining parameters affect the machinability of manganese-alloyed ADI produced using a two-step austempering method. Statistical analysis was performed using ‘Minitab’ software to develop a regression equation for determining the machining results in terms of the tool life and surface roughness. These equations can be used to predict the machinability of this material for any value of the heat treatment and machining parameters within the range of values considered in this study.
2. Materials and methods
2.1. Material composition
To produce austempered ductile iron, the material initially required was spheroidal graphite iron. The raw materials were melt in the medium frequency induction furnace and the required alloying elements were added to the melt followed by nodulization and inoculation. The melt was poured into the standard 1-inch Y block mold and the pouring temperature was in the range of 1490-1520 °C. This was obtained from the casting of ductile iron, as per the ASTM standard A897/A897M-15 (ASTM A897/A897M-15, Citation2015). Three ductile iron alloys with different Mn contents were obtained. The compositions of the ductile iron alloys are given in .
Table 1. Chemical composition of ductile iron.
2.2. Heat treatment and characterization
A muffle furnace was used to heat the specimen to the austenitization temperature. The samples were maintained at an austenetization temperature of 950 °C for a fixed holding time. The samples were then quickly quenched in a salt bath maintained at a much lower temperature of 260 °C for a short duration of 5 min, after which they were transferred back to another salt bath furnace maintained at the austempering temperature for a fixed duration before cooling the samples in air to room temperature. The heat treatment cycle is shown in .
The various processes involved in the novel two-step austempering heat treatment process are shown in . The process is as follows.
- 2: Austenitization process of heating the samples from room temperature to the austenitization temperature of 950 °C.
- 3: Holding the samples at the austenitization temperature for 2 h.
- 4: Rapid quenching of the samples in the first salt bath maintained at 260 °C.
- 5: Holding the samples at 260 °C for short duration of 5 min for super cooling.
- 6: Transferring the samples to the second salt bath maintained at the austempering temperature (320, 370 and 420 °C).
- 7: Holding the samples at the austempering temperature for 2 h.
- 8: Air cooling of the samples to room temperature
The hardness of all specimens was determined using the Brinell hardness method according to the procedure given in ASTM E10 (ASTM E10-18, Citation2018). A scanning electron microscopy (SEM) study was carried out to obtain the microstructure of the heat-treated samples.
2.3. Machinability test
Machinability is the ease with which a material can be machined. Various factors affect machinability and are often expressed in terms of these factors. However, the tool life and surface finish obtained after machining are two important parameters used to quantify the machinability of any material is the tool life and surface finish obtained after machining (Çelik et al., Citation2017). In this study, a turning operation was performed in a CNC machine using a carbide insert as the cutting tool. The test specimens were prepared by machining the round bars of ductile iron castings. The dimension of the machinability test specimen is as per the ISO 3685 standard with 300 mm length and 30 mm diameter. The machining parameters such as speed, feed, and depth of cut were varied at three different levels, the details of which are explained in the next section of the design of the experiments. No cutting fluid was used during the machining. The tool life was obtained using the flank wear measurement concept according to the procedure given by ISO 3685 (ISO 3685: 1993 \(E\), 1993). The surface roughness value was measured according to ISO 4287 (ISO 4287, 4287, Citation1997) using a profilometer (Mitutoyo Surftest SJ 210).
2.4. Design of experiments
In this study, the austenitization temperature was kept constant at 950 °C for all the treatment processes. The other heat treatment parameters, such as the austempering temperature, manganese content, and machining parameters, vary at different levels, as shown in . The machining parameters are selected based on the practical usage values of different machining applications. Taguchi method is used to get the reduced number of trails for carrying out the machinability tests involving various factors at different levels. L27 orthogonal array is used to carry out machinability tests for different sets of parameters.
Table 2. Details of various factors used.
3. Results and discussions
3.1. Mechanical characterization
The Brinell hardness values of the heat-treated samples were measured, and the results are listed in .
Table 3. Hardness results.
3.2. Microstructure analyses
The SEM images of the ADI samples obtained using the novel two-step heat treatment are shown in and . The two images listed in show the microstructures of the ADI samples austenetized at 950 °C and austempered at two different temperatures of 320 and 420 °C for the samples with 0.28 wt% of manganese content. It can be seen that a fine acicular ferrite structure is obtained at the lower austempering temperature of 320 °C, and a coarse feathery ferrite is obtained at the higher austempering temperature of 420 °C. This is the reason for the higher hardness obtained at lower austempering temperatures. shows SEM images of the ADI samples with 1.01 wt% manganese, which were austempered at 320 and 420 °C. This result is in accordance with few of the reported studies regarding the production ADI (Dakre et al., Citation2017; Dasgupta et al., Citation2013; Francucci et al., Citation2008; Górny et al., Citation2023; Hegde & Sharma, Citation2018; Hsu & Chuang, Citation2001; Liu et al., Citation2023; Putatunda & Gadicherla, Citation2000). However, in many of the studies reported about the high amount of manganese alloyed ADI, there is a formation of carbides at the grain boundary (Bendikiene et al., Citation2021; Górny et al., Citation2021; Ingxu et al., Citation2020; Olawale & Oluwasegun, Citation2016; Pereira et al., Citation2020; Zhiwang et al., Citation2022). The novel heat treatment method which is adopted in the current study did not result in carbide formation at the grain boundary because of the presence of manganese. This ensures that machinability will not be affected negatively by the addition of 1 wt% manganese when the ADI is produced using this novel heat treatment method.
Figure 2. SEM images of ADI with 0.28 wt% Mn austenitized at 950 °C for 2 h and austempered at (a) 320 °C and (b) 420 °C.
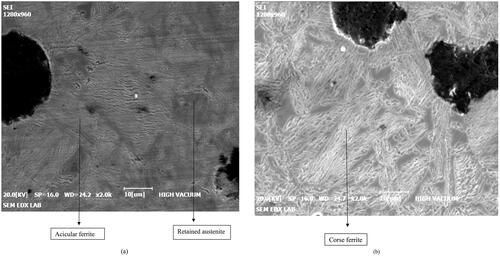
Figure 3. SEM images of ADI with 1.01 wt% Mn austenitized at 950 °C for 2 h and austempered at (a) 320 °C and (b) 420 °C.
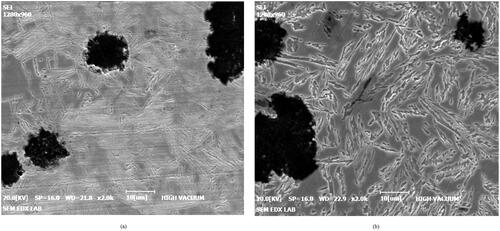
From the results in and the SEM images in and , it is evident that a slight increase in hardness is observed for the higher manganese content. However, there is no evidence of carbide formation at the grain boundaries, which is seen as a beneficial effect on machinability. This is in accordance with the reported articles regarding alloyed ADI (Gecu, Citation2022; Nalcaci et al., Citation2023; Sylvester et al., Citation2020).
3.3. Machinability test results
provides the results for the tool life and surface roughness obtained in various trials conducted with varying values for the machining and heat treatment parameters. Average of 3 test readings are taken and tabulated.
Table 4. Tool life and surface roughness results.
Statistical analysis was performed to determine the relative contribution of each factor to the tool life and surface roughness. Analysis of Variance (ANOVA) was used for this purpose. Also. Regression equations were fit to determine the tool life and surface roughness for the factors involved in the range of values considered in this study.
3.2.1. Analysis of variance (ANOVA) for tool life
ANOVA was carried out at 5% significance level for all the factors. The results of the ANOVA of the tool life are shown in .
Table 5. ANOVA results for tool life.
provides the ANOVA results for the tool life. It may be seen that P value for almost all factors are less than 0.05 indicating all factors are statistically significant. From the results provided in , it is evident that the austempering temperature is the major contributing factor to tool life, followed by the manganese content. These two factors together contributed to the majority of the changes in tool life within the range of values under study. However, the relative contribution of machining parameters is not significant for the range of values considered in this study.
The main effect plot for the tool life is shown in . This helps to understand tool life with the variation in all independent variables. As the austempering temperature increased from 320 to 420 °C, the tool improved drastically. This could be due to the lower hardness observed at higher austempering temperatures. This is the result of the coarser ferrite obtained at higher austempering temperatures. The tool life was found to decrease with an increase in Mn content. Although no carbide was formed along the grain boundary, a slight increase in the hardness resulted in a slight deterioration of the tool life. However, considering the hardenability benefit with higher manganese content, this marginal reduction in tool life at higher manganese content is not of much concern for machinability. The machining parameters of speed, feed, and depth of cut did not significantly contribute to the variation in tool life, as seen in the main effect plot shown in .
3.2.2. Analysis of variance (ANOVA) for surface roughness
The details of ANOVA results for the surface roughness are provided in .
Table 6. ANOVA results for surface roughness.
From , it can be seen that the major contributing factor to the variation in surface roughness is the austempering temperature, followed by the feed rate and manganese content. However, based on the p value, which is greater than 0.05, we may speculate that apart from austempering temperature, the other parameters may not be statistically significant with respect to the variation in surface roughness for the range of values considered in the current research work.
The nature of the variation in surface roughness with different parameters is shown as the main effect plot in . It is a well-known fact that finer grains produces the good surface finish with lower surface roughness value. The microstructure study clearly shows the change in grain structure as the austempering temperature increases from 320 to 420 °C. This is the reason for obtaining a lower surface roughness value at the lower austempering temperature because of the presence of finer ferrite grains. Even though a slight deterioration of the surface finish was observed with an increase in the manganese content from 0.28 to 0.64 wt%, the same surface finish was observed for a further increase in the manganese content. This could be possible because of the absence of carbide formation in the 1.01 wt% manganese alloy. Furthermore, a speed of 120 m/min resulted in an optimum surface finish. Better contact between the tool and workpiece may have contributed to the improvement in the surface finish with increasing cutting speed.
Regression equations were generated to predict tool life and surface roughness (EquationEquations (1)(1)
(1) and Equation(2)
(2)
(2) , respectively). These equations have a good fit with higher R squared value which assures that they may be used to predict the tool life and surface roughness for the factors involved with the range of values considered in this study.
(1)
(1)
EquationEquation (1)(1)
(1) gives the regression equation for tool life. The R squared value obtained for this equation was 97%, which indicates a good fit.regression equation for surface roughness is provided in EquationEquation (2)
(2)
(2) with an R-squared value of 85%.
(2)
(2)
Minitab software is used to carry out all the statistical analysis along with optimization. In order to obtain the high tool life with minimum surface roughness value, response surface optimzation is carried out with goal selected as “maximum” for tool life and “minimum” for surface roughness values. The following are the values for the various factors used in the current research to obtain the optimum combination of the tool life and surface roughness.
Austempering Temperature = 420 °C
Manganese content = 0.28 wt%
Speed = 92.72 m/min
Feed = 0.17 mm/rev
Depth of cut = 0.9 mm
4. Conclusions
In this study, a novel two-step austempering heat treatment was performed on Mn-alloyed ductile iron. It is shown that up to 1 wt% manganese-alloyed ADI can be successfully produced with good machinability. Further, the following points provide details about the specific outcomes of the study.
Microstructure analysis of the sample produced using the novel method did not show any carbide formation for the higher manganese content ADI, which is helpful in producing ADI with good machinability.
Tool life and surface roughness were mainly affected by the austempering temperature, whereas a higher speed of 120 m/min resulted in an optimum surface finish.
Regression equations were successfully generated to predict the tool life and surface finish of the manganese-alloyed ADI produced using the novel heat-treatment method. The high R squared value of 97% shows the excellent fit for the regression equation developed.
Response surface optimization provided an optimum cutting speed of 92.7 m/min in order to obtain an optimum combination of tool life and surface finish.
Author’s contributions
Ananda Hegde: Writing original draft preparation, visualization. Gajanan Anne: Conceptualization, methodology, investigation. Gowrishankar M C & Karthik B M: Resources, validation, review. Sathyashankara Sharma & Melwyn Rajesh Castelino: Validation, review & editing.
Consent for publication
The authors confirm that the work described has not been published.
Disclosure statement
No potential conflict of interest was reported by the authors.
Data availability statement
Authors agree to share the data upon reasonable request.
Additional information
Notes on contributors
Ananda Hegde
Dr. Ananda Hegde is working as Associate Professor in the Department of Mechanical & Industrial Engineering, MIT, MAHE, Manipal. He holds M.Tech (Materials Engineering) and Ph.D. (Heat treatment) degrees. He has more than 10 years of teaching experience. His area of interest includes materials engineering, heat treatment of metals. He has published more than 50 papers in journals and conferences.
Gajanan Anne
Dr. Gajana Anne is working as Assistant Professor in the Department of Mechanical & Industrial Engineering, MIT, MAHE, Manipal. He holds PhD degree in Mechanical Engineering. He has more than 10 years of teaching experience. His area of interest includes surface treatment, coating of metals and alloys.
Gowrishankar M. C.
Dr. Gowri Shankar, holds a Ph.D. from the Manipal Institute of Technology, and currently is a Professor at the Department of Mechanical and Industrial Engineering of the same institute. His main areas of research include Machining of Materials; Heat Treatment of Ferrous and Non-Ferrous Materials.
Sathyashankara Sharma
Dr. Sathyashankara Sharma is working as Senior Professor in the Department of Mechanical & Industrial Engineering, MIT, MAHE, Manipal. He holds M.Tech. (Materials Engineering) and Ph.D. (Materials Engineering) degrees. He has more than 35 years of teaching experience. His area of interest includes materials engineering, heat treatment of metals and composites and deformation behaviour of metals and composites. He has published more than 150 papers in journals and conferences.
Karthik B. M.
Dr. Karthik B. M. holds PhD degree from Manipal Institute of Technogy, Manipal. He is working a assisting professor at MIT Manipal. His research includes age hardening of alloys and characterization.
Melwyn Rajesh Castelino
Dr. Melwyn Rajesh Castelino is working as assistant professor in the mechanical engineering department of NMAMIT, Nitte. He has more than 15 years of teaching experience.
References
- Artola, G., Gallastegi, I., Izaga, J., Barreña, M., & Rimmer, A. (2017). Austempered ductile iron (ADI) alternative material for high-performance applications. International Journal of Metalcasting, 11(1), 131–135. https://doi.org/10.1007/s40962-016-0085-8
- ASTM A897/A897M-15. (2015). Standard specification for austempered ductile iron castings, Annual Book of ASTM Standards, Vol. 01.02. ASTM International.
- ASTM E10-18. (2018). Standard Test Method for Brinell Hardness of Metallic Materials, Vol. 01.02. ASTM International.
- Bayati, H., & Elliot, R. (1995). Influence of austenitising temperature on mechanical properties of high manganese alloyed ductile iron. Materials Science and Technology, 11(9), 908–913. https://doi.org/10.1179/mst.1995.11.9.908
- Bendikiene, R., Ciuplys, A., Cesnavicius, R., Jutas, A., Bahdanovich, A., Marmysh, D., Nasan, A., Shemet, L., & Sherbakov, S. (2021). Influence of austempering temperatures on the microstructure and mechanical properties of austempered ductile cast iron. Metals, 11(6), 967. https://doi.org/10.3390/met11060967
- Çelik, Y. H., Kilickap, E., & Güney, M. (2017). Investigation of cutting parameters affecting on tool wear and surface roughness in dry turning of Ti-6Al-4V using CVD and PVD coated tools. Journal of the Brazilian Society of Mechanical Sciences and Engineering, 39(6), 2085–2093. https://doi.org/10.1007/s40430-016-0607-6
- Cemal Cakir, M., & Isik, Y. (2008). Investigating the machinability of austempered ductile irons having different austempering temperatures and times. Materials & Design, 29(5), 937–942. https://doi.org/10.1016/j.matdes.2007.04.002
- Dakre, V., Peshwe, D. R., Pathak, S. U., & Likhite, A. (2017). Mechanical characterization of austempered ductile iron obtained by two step austempering process. Transactions of the Indian Institute of Metals, 70(9), 2381–2387. https://doi.org/10.1007/s12666-017-1099-5
- Dasgupta, R. K., Mondal, D. K., & Chakrabarti, A. K. (2013). Evolution of microstructures during austempering of ductile irons alloyed with manganese and copper. Metallurgical and Materials Transactions A, 44(3), 1376–1387. https://doi.org/10.1007/s11661-012-1502-0
- Elsayed, A. H., Megahed, M. M., Sadek, A. A., & Abouelela, K. M. (2009). Fracture toughness characterization of austempered ductile iron produced using both conventional and two-step austempering processes. Materials & Design, 30(6), 1866–1877. https://doi.org/10.1016/j.matdes.2008.09.013
- Francucci, G., Sikora, J., & Dommarco, R. (2008). Abrasion resistance of ductile iron austempered by the two step process. Materials Science and Engineering: A, 485(1-2), 46–54. https://doi.org/10.1016/j.msea.2007.07.081
- Gecu, R. (2022). Microstructure, mechanical, and wear properties of Al-alloyed austempered ductile irons. Tribology Transactions, 65(5), 952–962. https://doi.org/10.1080/10402004.2022.2117112
- Górny, M., Gondek, Ł., Angella, G., Tyrała, E., Kawalec, M., & Bitka, A. (2023). Structural stability of thin-walled austempered ductile iron castings. Archives of Civil and Mechanical Engineering, 23(2), 79. https://doi.org/10.1007/s43452-022-00597-0
- Górny, M., Gondek, Ł., Tyrała, E., Angella, G., & Kawalec, M. (2021). Structure homogeneity and thermal stability of austempered ductile iron. Metallurgical and Materials Transactions A, 52(6), 2227–2237. https://doi.org/10.1007/s11661-021-06214-8
- Hegde, A., & Sharma, S. (2018). Machinability study of manganese alloyed austempered ductile iron. Journal of the Brazilian Society of Mechanical Sciences and Engineering, 40(7), 338. https://doi.org/10.1007/s40430-018-1258-6
- Hsu, C., & Chuang, T. (2001). Influence of stepped austempering process on the fracture toughness of austempered ductile iron. Metallurgical and Materials Transactions A, 32(10), 2509–2514. https://doi.org/10.1007/s11661-001-0040-y
- Ingxu, W., Barber, G. C., Qiu, F., Zou, Q., & Yang, H. (2020). A review: phase transformation and wear mechanisms of single-step and dual-step austempered ductile irons. Journal of Materials Research and Technology, 9(1), 1054–1069. https://doi.org/10.1016/j.jmrt.2019.10.074
- ISO 3685: 1993 (E). (1993). Tool-life testing with single point tools.
- ISO 4287. (1997). Geometrical product specifications (GPS) – surface texture: Profile method – terms, definitions and surface texture parameters.
- Liu, C., Du, Y., Wang, X., Zheng, Q., Zhu, X., Zhang, D., Liu, D., Yang, C., & Jiang, B. (2023). Comparison of the tribological behavior of quench-tempered ductile iron and austempered ductile iron with similar hardness. Wear, 520-521pp, 204668. https://doi.org/10.1016/j.wear.2023.204668
- Nalcaci, B., Davut, K., Neite, M., Münstermann, S., & Erdogan, M. (2023). Influence of partitioning treatment on microstructure and mechanical properties of an alloyed ductile iron austempered at different temperatures. Materials Testing, 65(6), 896–910. https://doi.org/10.1515/mt-2022-0421
- Nan, R., Fu, H., Yang, P., Lin, J., & Guo, X. (2020). Microstructure evolution and wear resistance of Cu-bearing carbidic austempered ductile iron after austempering. Journal of Materials Engineering and Performance, 29(4), 2440–2459. https://doi.org/10.1007/s11665-020-04788-9
- Olawale, J. O., & Oluwasegun, K. M. (December 29, 2016). Austempered ductile iron (ADI): A Review. ASTM International. Materials Performance and Characterization, 5(1), 289–311. 2016. https://doi.org/10.1520/MPC20160053
- Pereira, L., Amaral, R. F. d., Wolfart, M., & Barcellos, V. K. d (2020). Microstructural and mechanical properties of Cu-Ni-Mn-Mo austempered ductile iron obtained from two-step hot air austempering. Journal of Materials Research and Technology, 9(3), 3055–3063. https://doi.org/10.1016/j.jmrt.2020.01.036
- Putatunda, S. K. (2001). Development of austempered ductile cast iron (ADI) with simultaneous high yield strength and fracture toughness by a novel two-step austempering process. Materials Science and Engineering: A, 315(1-2), 70–80. https://doi.org/10.1016/S0921-5093(01)01210-2
- Putatunda, S. K., & Gadicherla, P. K. (2000). Effect of austempering time on mechanical properties of a low manganese austempered ductile iron. Journal of Materials Engineering and Performance, 9(2), 193–203. https://doi.org/10.1361/105994900770346150
- Sylvester, O. O., Oyetunji, A., Alaneme, K. K., & Olubambi, P. A. (2020). Structural characterization and mechanical properties of pearlite – Enhanced micro-alloyed ductile irons. Journal of King Saud University - Engineering Sciences, 32(3), 205–210. https://doi.org/10.1016/j.jksues.2018.11.008
- Zhang, J., Zhang, N., Zhang, M., Lu, L., Zeng, D., & Song, Q. (2014). Microstructure and mechanical properties of austempered dutcile iron with different strength grades. Materials Letters, 119, 47–50. https://doi.org/10.1016/j.matlet.2013.12.086
- Zhiwang, S., Xiaohui, Z., Yufan, S., Hanguang, F., Xingye, G., & Jian, L. (2022). Microstructure and properties of high manganese carbidic austempered ductile iron. Transactions of the Indian Institute of Metals, 75(3), 833–842. https://doi.org/10.1007/s12666-021-02476-3
- Zimba, J., Simbi, D. J., & Navara, E. (2003). Austempered ductile iron: An alternative material for earth moving components. Cement and Concrete Composites, 25(6), 643–649. https://doi.org/10.1016/S0958-9465(02)00078-1