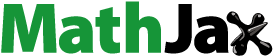
Abstract
This investigation delves into the dynamics of stress distribution during particle impacts on Incisor teeth, revealing the significant roles of particle size, impact angle, and standoff distance. An explicit dynamics study highlights a crucial revelation: optimal outcomes are achieved with a particle size of 250 µm, a 5 mm standoff distance, and an 80-degree impact angle, resulting in maximal stress manifestation. The analysis highlights a direct relationship between particle size and stress distribution, where larger particles amplify stress levels, emphasizing its pivotal role in determining stress distribution patterns. Additionally, an investigation of the Signal-to-Noise (SN) ratio reaffirms this trend, validating the direct correlation between stress distribution magnitude and abrasive particle size. Interestingly, variations in standoff distance and impact angle exhibit relatively minor effects. The ANOVA further strengthens these findings, with lower p-values for particle size and impact angle, highlighting their substantial contributions to stress distribution. Exploring interactions uncovers significant relationships between abrasive particle size and impact angle, as well as impact angle and standoff distance, with implications for stress distribution optimization. This study offers a broad investigation of the effects of abrasive particle size, standoff distance, and handle angulation on stress distribution during air abrasive procedures in dentistry. Through advanced computational modelling techniques, we interpret the complex interactions between these parameters and their impact on tooth surface integrity. The research findings not only provide valuable insights into optimizing procedure parameters for enhanced safety and efficacy but also contribute to the advancement of knowledge in the field of dental materials and techniques.
REVIEWING EDITOR:
1. Introduction
Air polishers have gained significant popularity in the field of dentistry due to their efficiency and effectiveness in cleaning and preparing tooth surfaces. These dental instruments utilize a combination of compressed air, water, and fine abrasive powders to remove dental plaque, stains, and debris from teeth (Matson et al., Citation2012). It involves the use of a specialized handpiece that delivers a high-speed stream of fine abrasive particles, such as aluminum oxide or silica, propelled by compressed air. Air polishers provide superior cleaning compared to traditional methods like scaling and polishing. Air polishers excel at removing dental plaque, the sticky film of bacteria that accumulates on teeth surfaces over time. This plaque can lead to the development of cavities and gum disease if not adequately addressed. Depending upon the patient’s medical conditions the assessment to opt for use of the air abrasion is considered (Gutmann, Citation1998; Wilkins, Citation2009).
Air abrasion has gained popularity in recent years due to its several advantages over traditional polishing methods, such as using rubber cups and polishing paste. Some of the advantages of air polishing include; efficiency, minimal tooth removal, access to hard-to-reach zones and less discomfort (Barnes, Citation2010). Gerbo et al. (Citation1993), studied the air polishing procedures of the dental surface and also initiated the optimal position of the nozzle for plaque and stain removal from the orthodontic bracket. The authors suggested the held angle depending on the tooth area to be accessed. Leon et al. (Citation2016) carried out the practical evaluation of the Bio-Art micro blaster, an air abrasion device. For the study, they considered the retention of various restoration materials applied to dental teeth preparation during air abrasion procedures. Additionally, they also examined the level of patient approval and gathered insights from dentists regarding this technique. The authors concluded that the preparation time is lengthier compared to that of burs, the air abrasion technique is more favorably embraced by patients, primarily because it is less painful. Paolinelis et al. (Citation2009) explored the factors such as propellant air pressure, abrasive powder flow rate, abrasive size, nozzle-to-substrate angle, and distance that could impact the cutting rate and profiles generated during both static and dynamic cutting of an enamel substitute. The authors suggested maintaining the nozzle angle of 60 degrees and a standoff distance of 5 mm away from the enamel surface to achieve the highest cutting rate with a maximum propellant and flow rate of particles of 2.5 g/min. Coskun et al. (Citation2018) compared the effect of grit size, standoff distance and air pressure on the metal substances and evaluated their bond strength. For the study authors adopted 50, 110, and 250 µm Al2O3 at 25, and 50, 75 psi for 10, 20, and 30 s at a distance of 10, 20, and 30 mm. The authors concluded that the surface roughness shows that selected parameters are effective when pressure and gain size vary. Tastepe et al. (Citation2017) optimized the air polishing method on the titanium surface by considering the size and shape of the cleaning surface. They adopted the different optimized device settings, nozzle movement and cutting depth. They concluded for the effective cleaning of teeth, high pressure, nozzle movement and nozzle insertion with water flow is necessary.
Design of Experiments (DOE) offers a statistical approach that facilitates the systematic investigation of multiple parameters and their interactions with a system’s response (Islam & Pramanik, Citation2016; Krishnaiah & Shahabudeen, Citation2012). By applying DOE, dental researchers, and practitioners can discern critical factors influencing air abrasion and establish optimized process parameters to minimize risks while ensuring effective material removal. One prominent technique within the realm of DOE is the Taguchi Method, developed by Genichi Taguchi (Stewardson, Citation2001; Taguchi et al., Citation2004). This method provides a systematic framework for optimizing processes and achieving robust product designs (Tsui, Citation1992). Taguchi Method’s central focus is on minimizing variation and improving quality by identifying key factors affecting a process and determining optimal parameter settings (Lin & Chang, Citation2021; Peng et al., Citation2022). Peruchi et al. (Citation2013) evaluated the abrasion tips, operation parameters and process times for the dental enamel surface. Also, ANOVA (Analysis of variance) was employed and compared the effect of the operation mode. Their study concludes that the sonic tips remove more dental tissues when compared to the conventional air abrasion tips. Gupta et al. (Citation2021) optimized dental implants using the design of experiments. They used finite element analysis to assess the biomechanical performance. By analyzing the stresses, they concluded that the maximum stress found in the cortical and cancellous bone depends on the implant.
Finite Element Analysis (FEA) has emerged as a potent numerical tool for analyzing complex stress distributions in various engineering and biomechanical applications (Eram et al., Citation2020; Keni et al., Citation2019). In the domain of dental materials science, FEA has proven successful in investigating stress patterns and mechanical behavior in dental structures (Murakami & Wakabayashi, Citation2014; Valera-Jiménez et al., Citation2020). Through the construction of a virtual model of the tooth and simulation of the air abrasion process, FEA enables the visualization and quantification of stress distribution on dental surfaces under varying operating conditions.
This study aims to conduct a Finite Element Analysis of stress distribution on dental teeth subjected to air abrasion, exploring the impact of key process parameters such as abrasive particle size, nozzle distance, and angle of application on stress levels. Understanding the parameters that generate maximum stress on the tooth surface during air abrasive procedures is essential for ensuring safety, optimizing procedure parameters, preventing tooth damage, enhancing patient comfort, and promoting long-term tooth health. Furthermore, the results of this analysis provide valuable insights for dental practitioners and researchers to ensure safe and efficient air abrasion procedures. Through the application of DOE, dental professionals can establish robust and reliable protocols to optimize the process parameters, leading to improved patient outcomes and reduced risk of structural damage. This study contributes broad investigation of the effects of abrasive particle size, standoff distance, and handle angulation on stress distribution during air abrasive procedures in dentistry. Through advanced computational modelling techniques, we interpret the complex interactions between these parameters and their impact on tooth surface integrity. The research findings not only provide valuable insights into optimizing procedure parameters for enhanced safety and efficacy but also contribute to the advancement of knowledge in the field of dental materials and techniques.
2. Material and methods
2.1. Taguchi’s DOE method
Taguchi’s Design of Experiment (DOE) methodologies have found extensive application in the realm of engineering design (Su et al., Citation1999; Yang & Tarng, Citation1998). The central essence of Taguchi’s DOE methodologies revolves around parameter design, a robust engineering approach employed in the design of products or processes. This approach specifically aims to identify the optimal settings of parameters (factors) that yield superior levels of a quality characteristic (performance measure), while concurrently minimizing variability (Durakovic, Citation2017; Meena et al., Citation2017; Tzeng et al., Citation2009). FEA of stress distribution on teeth conducted based on Taguchi Experimental Design. Simulations were carried out as per L27 Taguchi full factorial design by varying process parameters such as abrasive particle size, standoff distance, and angle of application. The process parameters in FEA simulation varied in three levels and the corresponding values are shown in .
Table 1. Levels of process variables of the simulation (Barnes, Citation2010; Graumann et al., Citation2013; Peng et al., Citation2022; Sawai et al., Citation2015).
2.2. Finite element modelling
The explicit method was adopted for the analysis. For the image processing, the tooth was subjected to Cone Beam Tomography (CBCT). A series of cross-sections of the tooth were acquired at uniform intervals of 1 mm in DICOM format. The DICOM images are fed into a 3D slicer a computer-based imaging software to extract the 3D dental model. The model is generated with the pulp, dentin, and enamel as shown in . Dental air abrasion is particularly useful for treating small cavities, removing superficial stains, and preparing teeth for dental bonding or sealants. The particle which impacts the teeth is assumed to be spherical. The analysis is carried out to investigate the stress distribution on the teeth by considering the parameters as per the L27 Taguchi method and the average stress distribution of the analysis is shown in . These parameters include abrasive particle size, standoff distance (SoD), and angle of impact (AoI). shows the parameters considered for the simulation. Two sets of simulations were performed by varying particle size, standoff distance and angle of impact and the average stress distribution of the analysis was considered for the statistical analysis.
Table 2. Orthogonal array for L27 simulation design and stress distribution.
2.3. Boundary condition and meshing
Using the ANSYS 2023 R2 (ANSYS INC) software the modeling and meshing are completed as shown in . A mesh size is considered 0.5 mm. A total of 108,077 elements and 20,669 nodes are generated for the analysis with element type SOLID 187. Which is 10-node higher order 3D element in the elemental library fits for irregular mesh applicable to the patient-specific dental model (). shows the material properties that have been applied to the dental models. For the boundary condition, the lower surfaces are fixed. These surfaces are considered fixed because they are stable and not subjected to movement or deformation during the procedure. Dentists typically use a dental dam or other protective measures to isolate the tooth being treated, ensuring that the neighboring teeth and tissues are not affected by the procedure. The particles are assumed to be rigid and the teeth are treated as elastic for the explicit dynamics analysis. Abrasive particles used in the air abrasion system are propelled by compressed air and directed onto the front face of the tooth with a specific velocity as shown in . The compressed air consists the pressure. The pressure is converted to the velocity using EquationEq. (1)(1)
(1) . The impact velocity of 28.764 m/s is approved and made to impact the teeth surface.
(1)
(1)
Table 3. Dental model material properties (Brandão & Brandão, Citation2013; Eram et al., Citation2020; Karimi et al., Citation2020).
where is the velocity (m/s),
represents the fluid mass density (kg/m3), and
signifies the dynamics pressure (Pa).
3. Results and discussion
Explicit dynamics analysis is being conducted within the ANSYS Workbench-R2 environment. The simulation involves setting up a virtual model of the Incisor teeth, including geometry and material properties, within the ANSYS Workbench-R2 software. The primary objective of this analysis is to understand the behavior of a single particle as it impacts a modelled set of Incisor teeth. The analysis can provide valuable information about potential damage or stresses that may occur as a result of such impacts.
3.1. Stress analysis
A total of 27 simulations were carried out as per the Taguchis DOE matrix (). The stress results are analyzed as per the parameters shown in . From , it was found that the maximum stress is obtained when the particle size is 250 µm, the Standoff distance of 5 mm and the angle of impact is 80 degrees. Under these specific conditions, the analysis of the explicit dynamics yielded a maximum stress value of 1.41709 MPa within the material of the modelled Incisor teeth (). Based on the explicit dynamics analysis different combinations of particle size, angle of impact, and standoff distance. For the particle size 250 µm, the maximum stress occurred at an angle of impact of 80 degrees and a standoff distance of 5 mm. Minimum stress occurred at an angle of impact of 90 degrees and a standoff distance of 3 mm. Similarly, for the particle size of 150 µm, maximum stress occurred at an angle of impact of 80 degrees and a standoff distance of 5 mm. Minimum stress occurred at an angle of impact of 60 degrees and a standoff distance of 3 mm. These findings suggest that, for both particle sizes (250 µm and 150 µm), the maximum stress is achieved when the angle of impact is 80 degrees, and the standoff distance is 5 mm. On the other hand, the minimum stress is observed under different conditions: when the angle of impact is 90 degrees and the standoff distance is 3 mm for the 250 µm particle size, and when the angle of impact is 60 degrees and the standoff distance is 3 mm for the 150 µm particle size. These results indicate that the angle of impact and standoff distance have a significant influence on the stress distribution within the modelled Incisor teeth during particle impact. The optimal parameters can inform practitioners in optimizing their technique to minimize stress concentration and potential damage to tooth structures during air abrasive procedures. From , it is clear that there is a direct relationship between the size of the impacting particle and the resulting stress within the modelled system. As the particle size increases, the stress levels also increase. This finding indicates that larger particles generate higher stress on the materials they impact. This finding suggests that larger particles generate higher stress on the materials they impact, which has implications for treatment planning and optimization of air abrasive procedures.
Figure 4. Stress distribution due to particle impact (a) 250 µm, 5 mm, 80° (b) 250 µm, 3 mm, 90° (c) 150 µm, 5 mm, 80° (d) 150 µm, 3 mm, 60°.
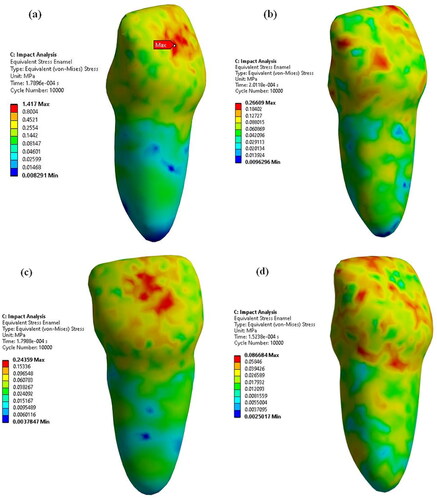
Figure 5. Particle impact on the incisor (a) SoD- 3 mm, 4 mm and 5 mm and AoI- 60°, (b) SoD- 3 mm, 4 mm and 5 mm and AoI- 80°, (c) SoD- 3 mm, 4 mm and 5 mm and AoI- 90°.
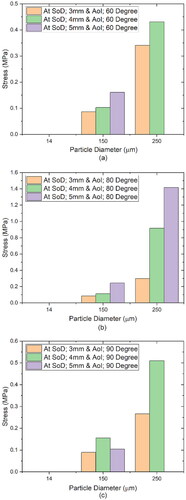
indicates that for higher-sized particles (250 µm), specifically when the impact angle is set to either 60 degrees or 90 degrees, and the maximum standoff distance of 5 mm is used, indicates no significant stress is generated within the teeth material. When the standoff distance is 5 mm and the angle of impact is 80 degrees the maximum stress is obtained in the teeth. Similarly, when the particle size is 150 µm, there is a direct relationship between the standoff distance and the resulting stress. It was found that for the standoff distance (3 mm, 4 mm and 5 mm) and angle of impact (60 and 80 degrees), there is an increase in stress taking place as the standoff distance increases (). Also for the 90 degrees, the stress variation shows and the maximum was found 0.2435 MPa when the standoff distance is 4 mm (). The findings of the study have direct implications for clinical practice, guiding practitioners in selecting appropriate parameters to achieve desired treatment outcomes while minimizing risks. For example, adjusting the angle of impact and standoff distance according to the identified optimal values can help reduce stress concentration and enhance the safety and efficacy of air abrasive procedures.
Gerbo et al. (Citation1993), in their research, stated for the optimal result the parameters such as the angle of impact should be 60 degrees to 80 degrees for the posterior and 90 degrees for the occlusal surface. The frontal incisor used in this research is the anterior smooth surface. From , it is clear that the impact angle of 80 degrees affects the teeth by producing the maximum stress. This suggests that maintaining the impact angle within the recommended range can help achieve effective results while minimizing potential stress-related risks to tooth structures. Also in the same research article (Gerbo et al., Citation1993), the author indicates that the instrument should be maintained at a distance of roughly 4 to 5 mm from the surface of the tooth. In our result, the maximum stress is obtained when the angle of impact is 5 mm. This underscores the importance of controlling the standoff distance to optimize stress distribution and minimize potential damage during air abrasive procedures. A study conducted by White and Peyton (Citation1954), investigated the effects of an Air dent blasting device on tooth enamel. The study demonstrated that when this particular blasting device was utilized, an enamel loss of approximately 25 μm was observed. This enamel loss occurred under specific application conditions: the device was directed at the tooth surface at an angle ranging between 60 to 90 degrees (Mishkin et al., Citation1986). This reinforces the importance of optimizing parameters to achieve desired outcomes while minimizing adverse effects on tooth structures.
3.2. Effect of process parameters on stress distribution
In Taguchi’s Design of Experiments (DOE) method, the designation of ‘signal’ represents the desired value, while ‘noise’ corresponds to the undesired value. The objective of utilizing the S–N ratio to measure quantifying performance facilitates the creation of products and processes that exhibit resilience in the face of noise factors.
The S–N ratio serves as a quantifiable metric in place of the standard deviation due to the inverse relationship between mean and standard deviation. When the mean decreases, the standard deviation tends to decrease as well, and conversely. Two pertinent scenarios wherein the S–N ratio concept proves beneficial encompass enhancing quality through the reduction of variability and refining measurement techniques. For continuous attributes, the S–N ratio can be categorized into three distinct groups, as elucidated by EquationEqs. (1)(1)
(1) to Equation(3)
(3)
(3) (Bagci, Citation2016; Biswas & Satapathy, Citation2010; Mahapatra et al., Citation2008).
(1)
(1)
(2)
(2)
(3)
(3)
Here, y is the observed data, signifies the average of observed data,
denotes the variation of y and n represents the total number of observations. Given the aim of minimizing stress distribution, the ‘smaller the better’ characteristic inherent in Taguchi analysis is embraced within the context of this study. To ascertain the determinants exerting an impact on stress distribution, a comprehensive analysis of variance (ANOVA) is executed. This analytical procedure, performed at a confidence level of 95%, involves the utilization of specialized statistical software. The process parameters at different levels are represented in . It was found that the particle size is the dominant parameter in stress variation followed by standoff distance and angle of impact.
Table 4. Response table for signal to noise ratios smaller is better.
shows the influence of individual process parameters on stress distribution over the teeth surface. This finding suggests that larger abrasive particles result in higher stress levels on the tooth surface, which can have implications for treatment planning and optimization of air abrasive procedures. The conclusion that a particle size of 250 µm has a major impact on the abrasion process is supported by , which shows high-stress distribution across the incisor when using particles of this size. indicates that variations in standoff distance and angle of impact have the least influence on stress distribution over the teeth surface compared to abrasive particle size. While these parameters still play a role in the overall process, their impact on stress levels appears to be less significant compared to particle size. This highlights the importance of focusing on optimizing particle size to achieve desired outcomes and minimize potential risks to tooth structures during air abrasive procedures. The findings regarding the influence of abrasive particle size on stress distribution align with the observations reported by Coskun et al. (Citation2018). Their investigation into air abrasion protocols concluded that increasing the pressure and grit size of particles results in higher surface removal rates. This observation corroborates the present study’s findings and underscores the importance of understanding the effects of particle size on the quality and outcome of air abrasive procedures in dental treatments.
3.3. Analysis of variance (ANOVA) for SN ratios
The analysis of variance (ANOVA) presented in provides valuable insights into the effects of process parameters and their interdependencies on stress distribution over the teeth surface during air abrasion procedures. The assessment of the significant factors impacting stress distribution on teeth can be effectively conducted using p-values. These statistical values serve as crucial indicators of the strength of the association between factors and stress patterns. In the context of a 95% confidence level, a p-value below 0.05 holds considerable importance and suggests a high level of confidence that the factor substantial impact on the overall process. The analysis shows particle size and angle of impact exhibit reasonably lower p-values. The analysis of variance reveals that particle size and angle of impact exhibit reasonably lower p-values, indicating their strong association with stress distribution during the air abrasion process. This implies that these two factors are the primary contributors to variations in stress levels over the teeth surface. These factors emerge as key influencers when designing strategies to optimize parameters in dental applications. As a result, these insights from the analysis can guide practitioners and researchers in making informed decisions to enhance the efficacy and safety of dental procedures.
Table 5. Analysis of variance for stress distribution.
provides a visual representation of the interaction effects between various factors on stress distribution across the surface of teeth. It becomes evident that parallel lines do not intersect, signifying an absence of complete independence among these factors. However, within this context, it is important to note the emergence of a subtle interaction effect, potentially influencing stress distribution. This interaction effect could manifest between two specific pairs of factors: particle size and the angle of impact, as well as the angle of impact and the standoff distance. In practical terms, the potential interaction effect involving abrasive particle size and angle of impact suggests that modifying either the size of the abrasive particles or the angle at which they impact the surface might lead to a combined influence on stress distribution outcomes. Similarly, the interaction between the angle of impact and the standoff distance implies that adjusting the angle at which the tool impacts the surface or the distance between the tool and the surface could jointly shape the resultant stress distribution pattern.
Applying the findings of the present study to practical applications within a clinical setting can lead to improved treatment outcomes, enhanced patient care, and advancements in the field of dental practice. By optimizing procedure parameters, tailoring treatment plans, ensuring safety and efficacy, supporting education and training, developing guidelines, and fostering innovation, practitioners can leverage the knowledge gained from the study to enhance the quality and effectiveness of air abrasive procedures in clinical practice.
4. Conclusions
This comprehensive study underscores the pivotal roles played by particle size, impact angle, and standoff distance in influencing stress distribution during particle impacts on the modelled Incisor teeth. The culmination of these analyses provided invaluable insights into the mechanical behavior of the modelled Incisor teeth when subjected to varying impact scenarios.
Explicit dynamics study reveals that the optimal combination of parameters - particle size of 250 µm, standoff distance of 5 mm, and impact angle of 80 degrees – results in the highest stress manifestation. Minimal stress occurs at an impact angle of 90 degrees and a standoff distance of 3 mm. The study underscores the pivotal roles played by particle size, impact angle, and standoff distance in influencing stress distribution during particle impacts on the teeth. These parameters significantly affect the mechanical behavior of the teeth when subjected to varying impact scenarios.
A direct correlation is observed between particle size and stress, with larger particles leading to higher stress levels. This finding highlights the importance of particle size selection in managing stress distribution during air abrasive procedures.
The SN ratio analysis enhances our understanding of the relationship between process parameters and outcomes, facilitating informed decision-making and optimization of air abrasive procedures in dental practice. Overall, this finding underscores the significance of particle size as a key factor influencing stress distribution and emphasizes its importance in managing stress levels effectively during dental treatments.
Variations in standoff distance and impact angle have minor effects on stress distribution, suggesting that particle size plays a dominant role in determining stress intensity. This emphasizes the need to focus on optimizing particle size to achieve desired stress distribution outcomes.
The Analysis of Variance (ANOVA) with p-values demonstrates that particle size and angle of impact have lower p-values, signifying their significant contributions to stress distribution. This statistical analysis further supports the importance of these factors in influencing stress levels on the tooth surface.
Overall, the study offers a broad investigation of the effects of abrasive particle size, standoff distance, and handle angulation on stress distribution during air abrasive procedures in dentistry. Through advanced computational modelling techniques, we interpret the complex interactions between these parameters and their impact on tooth surface integrity. The research findings not only provide valuable insights into optimizing procedure parameters for enhanced safety and efficacy but also contribute to the advancement of knowledge in the field of dental materials and techniques.
Author contributions statement
Laxmikant G K: Conceptualization, Investigation, Methodology, Formal analysis, Writing – original draft, Writing – review & editing, Visualization. Thanachai Namlimhemnatee: Methodology, Formal analysis, Writing – original draft, Writing – review. Padmaraj N H: Methodology, Formal analysis, Writing – original draft, Writing – review & editing, Visualization. Chethan K N: ¨ Formal analysis, Visualization, Writing – review & editing, Afiya Eram: Visualization, Writing – review. Nisha Shetty: Formal analysis, Visualization. Saurabh Kumar: Methodology, Formal analysis, Visualization. Joompon Bamrungwong: Methodology, Formal analysis, Visualization.
Acknowledgements
The authors thank the Department of Aeronautical and Automobile Engineering, Manipal Institute of Technology, Manipal Academy of Higher Education, Manipal for providing the high computational facility to carry out this research.
Disclosure statement
No potential conflict of interest was reported by the author(s).
Data availability statement
The data available on request from the authors.
Additional information
Notes on contributors
Chethan K. N.
Chethan K. N. working as Associate Professor in the Department of Aeronautical and Automobile Engineering, Manipal Institute of Technology, Manipal Academy of Higher Education, Manipal, Karnataka, INDIA. He holds B. E. (Mechanical Engineering), M.Tech (Manufacturing Engineering & Technology) and Ph.D. (Computational Biomechanics) degrees. Author has worked as post-doctoral research associate in New Jersey institute of Technology, USA. He has a more than twelve years of teaching and research experience. His area of interest includes Biomechanics of the hip joint, Finite element analysis of biomedical implants, Composite materials, and Manufacturing of medical implants.
Thanachai Namlimhemnatee
Thanachai Namlimhemnatee worked as IASTE intern at the Department of Aeronautical and Automobile Engineering, Manipal Institute of Technology in India, supporting research in Optimizing Stress Distribution in Dental Air Abrasion through Desigh of Experiments: A Finite Element Analysis. Previously, he interned as engineer at Rajavithi Hospital, Bangkok, Thailand a government public hospital widely known for its emergency care. Thanachai holds a bachelor's degree in Biomedical Engineering with First-Class Honours from King Mongkut's University of Technology North Bangkok, Thailand.
Padmaraj N. H.
Padamraj N. H. working as Associate Professor in the Department of Aeronautical and Automobile Engineering, Manipal Institute of Technology, Manipal Academy of Higher Education, Manipal, Karnataka, INDIA. He holds B. E. (Automobile Engineering), M.Tech (Manufacturing Engineering & Technology) and Ph.D. (Composites) degrees. He has a more than 13 years of teaching and research experience. His area of interest includes Composite materials, and Manufacturing of Composites, fatigue analysis of composites.
Saurabh Kumar
Saurabh Kumar working as Associate Professor in the Department of Pediatric & Preventive Dentistry, Manipal College of Dental Sciences, Manipal, Manipal Academy of Higher Education, Manipal, Karnataka, INDIA. His arear of interests are Dental Caries, Pediatric Endodontics.
Afiya Eram
Afiya Eram working as Assistant Professor in the Department Of Conservative Dentistry & Endodontics, Manipal College of Dental Sciences, Manipal, Manipal Academy of Higher Education, Manipal, Karnataka, INDIA. Her area of interests are Regenerative Endodontics, Dental Materials, Endodontics, FEM.
Nisha Shetty
Nisha Shetty working as reader in the Department of Oral & Maxillofacial Pathology and Oral Microbiology, Manipal College of Dental Sciences, Manipal, Manipal Academy of Higher Education, Manipal, Karnataka, INDIA. Her area of interests are Benign and Potentially Malignant Oral lesions & conditions, Oral cancer.
Joompon Bamrungwong
Joompon Bamrungwong is a faculty of the Department of Industrial Physics and Medical Instrumentation at King Mongkut's University of Technology North Bangkok, Bangkok, Thailand. His area of research includes Finite element analysis, bone fracture and material science.
Laxmikant G. Keni
Laxmikant G. Keni graduated in Mechanical Engineering in 2002 from Visvesvaraya Technological University, Karnataka. He completed his Master's degree in computer-Aided Mechanical Design and Analysis in 2008 and his Ph.D. in ureter flow dynamics from Manipal Academy of Higher Education in 2023. He joined Manipal Institute of Technology, Manipal, as a Lecturer in the Department of Mechanical and Manufacturing Engineering in 2008. In 2010, he moved to the Department of Aeronautical and Automobile Engineering. His areas of interest include Fluid-Structure Interaction, Computational Bio-fluid Mechanics, CFD, Structural Analysis, and Design. He has completed several projects on FEM analysis of dental implants and machining operations using the Finite Element Method. He has published papers in Scopus and Web of Science journals. Currently, he is working as an Associate Professor in the Department of Aeronautical and Automobile Engineering.
References
- Bagci, M. (2016). Determination of solid particle erosion with Taguchi optimization approach of hybrid composite systems. Tribology International, 94, 336–345. https://doi.org/10.1016/j.triboint.2015.09.032
- Barnes, C. M. (2010). An in-depth look at air polishing. University of Nebraska - Lincoln University of Nebra. 2010. chrome-extension://efaidnbmnnnibpcajpcglclefindmkaj/https://digitalcommons.unl.edu/cgi/viewcontent.cgi?article=1013&context=dentistryfacpub.
- Biswas, S., & Satapathy, A. (2010). A study on tribological behavior of alumina-filled glass-epoxy composites using Taguchi experimental design. Tribology Transactions, 53(4), 520–532. https://doi.org/10.1080/10402000903491309
- Brandão, R. C. B., & Brandão, L. B. C. (2013). Finishing procedures in orthodontics: Dental dimensions and proportions (Microesthetics). Dental Press Journal of Orthodontics, 18(5), 147–174. https://doi.org/10.1590/S2176-94512013000500006
- Coskun, M. E., Akar, T., & Tugut, F. (2018). Airborne-particle abrasion; searching the right parameter. Journal of Dental Sciences, 13(4), 293–300. https://doi.org/10.1016/j.jds.2018.02.002
- Durakovic, B. (2017). Design of experiments application, concepts, examples: State of the art. Periodicals of Engineering and Natural Sciences (PEN), 5(3), 421–439. https://doi.org/10.21533/pen.v5i3.145
- Eram, A., Zuber, M., Keni, L. G., Kalburgi, S., Naik, R., Bhandary, S., Amin, S., & Badruddin, I. A. (2020). Finite element analysis of immature teeth filled with MTA, biodentine and bioaggregate. Computer Methods and Programs in Biomedicine, 190, 105356. https://doi.org/10.1016/j.cmpb.2020.105356
- Gerbo, L. R., Barnes, C. M., & Leinfelder, K. F. (1993). Applications of the air-powder polisher in clinical orthodontics. American Journal of Orthodontics and Dentofacial Orthopedics: Official Publication of the American Association of Orthodontists, Its Constituent Societies, and the American Board of Orthodontics, 103(1), 71–73. https://doi.org/10.1016/0889-5406(93)70109-2
- Graumann, S. J., Sensat, M. L., & Stoltenberg, J. L. (2013). Air polishing: A review of current literature. Journal of Dental Hygiene: JDH/American Dental Hygienists’ Association, 87(4), 173–180.
- Gupta, Y., Iyer, R., Dommeti, V. K., Nutu, E., Rana, M., Merdji, A., Biswas, J. K., & Roy, S. (2021). Design of dental implant using design of experiment and topology optimization: A finite element analysis study. Proceedings of the Institution of Mechanical Engineers. Part H, Journal of Engineering in Medicine, 235(2), 157–166. https://doi.org/10.1177/0954411920967146
- Gutmann, M. E. (1998). Air polishing: A comprehensive review of the literature. Journal of Dental Hygiene: JDH, 72(3), 47–56.
- Islam, M. N., & Pramanik, A. (2016). Comparison of design of experiments via traditional and Taguchi method. Journal of Advanced Manufacturing Systems, 15(03), 151–160. https://doi.org/10.1142/S0219686716500116
- Karimi, A., Razaghi, R., Biglari, H., Rahmati, S. M., Sandbothe, A., & Hasani, M. (2020). Finite element modeling of the periodontal ligament under a realistic kinetic loading of the Jaw system. The Saudi Dental Journal, 32(7), 349–356. https://doi.org/10.1016/j.sdentj.2019.10.005
- Keni, L. G., Kalburgi, S., Hameed, B. M. Z., Zuber, M., Tamagawa, M., & Shenoy, B. S. (2019). Finite element analysis of urinary bladder wall thickness at different pressure condition. Journal of Mechanics in Medicine and Biology, 19(05), 1950029. https://doi.org/10.1142/S0219519419500295
- Krishnaiah, K., & Shahabudeen, P. (2012). Applied design of experiments and Taguchi methods. PHI Learning.
- Leon, A., Ungureanu, L., & Puscasu, C. (2016). Air abrasion: Interdisciplinary modern technologies—Approach to minimally invasive treatment of dental caries. In Proceedings of the International Conference on Interdisciplinary Studies (ICIS 2016) - Interdisciplinarity and Creativity in the Knowledge Society. InTech. https://doi.org/10.5772/65419
- Lin, K.-W., & Chang, Y.-C. (2021). Use of the Taguchi method to optimize an immunodetection system for quantitative analysis of a rapid test. Diagnostics (Basel, Switzerland), 11(7), 1179. https://doi.org/10.3390/diagnostics11071179
- Mahapatra, S. S., Patnaik, A., & Satapathy, A. (2008). Taguchi method applied to parametric appraisal of erosion behavior of GF-reinforced polyester composites. Wear, 265(1-2), 214–222. https://doi.org/10.1016/j.wear.2007.10.001
- Matson, M. R., Lewgoy, H. R., Barros Filho, D. A., Amore, R., Anido-Anido, A., Alonso, R. C. B., Carrilho, M. R. O., & Anauate-Netto, C. (2012). Finite element analysis of stress distribution in intact and porcelain Veneer restored teeth. Computer Methods in Biomechanics and Biomedical Engineering, 15(8), 795–800. https://doi.org/10.1080/10255842.2011.561013
- Meena, A., Singh Mali, H., Patnaik, A., & Kumar, S. R. (2017). Comparative investigation of physical, mechanical and thermomechanical characterization of dental composite filled with nanohydroxyapatite and mineral trioxide aggregate. e-Polymers, 17(4), 311–319. https://doi.org/10.1515/epoly-2016-0319
- Mishkin, D. J., Engler, W. O., Javed, T., Darby, T. D., Cobb, R. L., & Coffman, M. A. (1986). A clinical comparison of the effect on the Gingiva of the prophy-jet and the rubber cup and paste techniques. Journal of Periodontology, 57(3), 151–154. https://doi.org/10.1902/jop.1986.57.3.151
- Murakami, N., & Wakabayashi, N. (2014). Finite element contact analysis as a critical technique in dental biomechanics: A review. Journal of Prosthodontic Research, 58(2), 92–101. https://doi.org/10.1016/j.jpor.2014.03.001
- Paolinelis, G., Banerjee, A., & Watson, T. F. (2009). An in-vitro investigation of the effects of variable operating parameters on alumina air-abrasion cutting characteristics. Operative Dentistry, 34(1), 87–92. https://doi.org/10.2341/08-52
- Peng, B.-R., Pan, L.-F., Huang, S.-H., Chen, C.-Y., Lin, C.-S., Chiang, F.-T., & Pan, L.-K. (2022). Practical application of Taguchi optimization methodology to medical facilities: An integrated study. Journal of Mechanics in Medicine and Biology, 22(08), 1–15. https://doi.org/10.1142/S0219519422400255
- Peruchi, C., Santos-Pinto, A., Dias, T. C., Oliveira, A. C. M., & Santos-Pinto, L. (2013). Influence of air abrasion tips and operation modes on enamel-cutting characteristics. European Journal of Dentistry, 7(1), 1–5.
- Sawai, M., Bhardwaj, A., Jafri, Z., Sultan, N., & Daing, A. (2015). Tooth polishing: The current status. Journal of Indian Society of Periodontology, 19(4), 375. https://doi.org/10.4103/0972-124X.154170
- Stewardson, D. (2001). Robust engineering, Taguchi G, Chowdhury S, Taguchi S, McGraw-Hill, New York, 2000. Hard-Bound, Number of Pages: 241. ISBN 0 07 134782 8. Quality and Reliability Engineering International, 17(2), 141–142. https://doi.org/10.1002/qre.382
- Su, Y. L., Yao, S. H., Wei, C. S., Kao, W. H., & Wu, C. T. (1999). Design and performance analysis of TiCN-coated cemented Carbide milling cutters. Journal of Materials Processing Technology, 87(1-3), 82–89. https://doi.org/10.1016/S0924-0136(98)00335-5
- Taguchi, G., Chowdhury, S., & Wu, Y. (2004). Taguchi’s quality engineering handbook. John Wiley & Sons, Inc. https://doi.org/10.1002/9780470258354
- Tastepe, C. S., Lin, X., Donnet, M., Wismeijer, D., & Liu, Y. (2017). Parameters that improve cleaning efficiency of subgingival air polishing on Titanium implant surfaces: An in vitro study. Journal of Periodontology, 88(4), 407–414. https://doi.org/10.1902/jop.2016.160270
- Tsui, K.-L. (1992). An overview of Taguchi method and newly developed statistical methods for robust design. IIE Transactions, 24(5), 44–57. https://doi.org/10.1080/07408179208964244
- Tzeng, C.-J., Lin, Y.-H., Yang, Y.-K., & Jeng, M.-C. (2009). Optimization of turning operations with multiple performance characteristics using the Taguchi method and Grey relational analysis. Journal of Materials Processing Technology, 209(6), 2753–2759. https://doi.org/10.1016/j.jmatprotec.2008.06.046
- Valera-Jiménez, J. F., Burgueño-Barris, G., Gómez-González, S., López-López, J., Valmaseda-Castellón, E., & Fernández-Aguado, E. (2020). Finite element analysis of narrow dental implants. Dental Materials: Official Publication of the Academy of Dental Materials, 36(7), 927–935. https://doi.org/10.1016/j.dental.2020.04.013
- White, H. D., & Peyton, F. A. (1954). Effects of air abrasive in prophylaxis. Journal of the American Dental Association (1939), 49(2), 155–163. https://doi.org/10.14219/jada.archive.1954.0139
- Wilkins, E. M. (2009). Clinical practice of the dental hygienist. Wolters Kluwer Health/Lippincott Williams & Wilkins.
- Yang, W. H., & Tarng, Y. S. (1998). Design optimization of cutting parameters for turning operations based on the Taguchi method. Journal of Materials Processing Technology, 84(1-3), 122–129. https://doi.org/10.1016/S0924-0136(98)00079-X