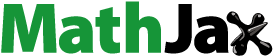
Abstract
This paper presents the results of several searches for dark matter with the ATLAS experiment at Large Hadron Collider using proton–proton collisions at TeV. These include searches for events with large missing transverse momentum and a photon, a single jet or
boson. Both hadronic and leptonic
decays are considered. In a number of models, the dark matter particles can be produced in association with heavy flavour (top or b-quarks). Results of these searches are summarised.
Public Interest Statement
Astrophysical and cosmological measurements show that our universe must contain a large quantity of matter that does not interact with light and is therefore “invisible” or “dark”. This matter is called dark matter. Today’s best explanation for this dark matter is that it is made of particles that interact weakly like neutrinos but that are relatively heavy, perhaps hundred times heavier than a proton. Such a particle is called weakly interacting massive particle (WIMP). One problem is that there is no known elementary particle that has the properties of a WIMP, therefore it must be a new, and yet an unknown particle.
The large hadron collider (LHC) at CERN collides protons at sufficiently high energy that it could produce dark matter WIMPs if they exist. The difficulty lies in finding the WIMPs among billions of recorded particle collisions. The ATLAS experiment has been used to examine the collisions and look for the WIMP particles in several possible experimental signatures.
1. Dark matter with standard model vector bosons
The origin of dark matter is one of the outstanding questions in contemporary physics. Collider experiments such as ATLAS are sensitive to the pair production of the so-called WIMPs in association with an initial state radiation leading to a final state jet, photon or ; we denote this reaction
, where
is the WIMP and
is either a jet, photon or
. The
pair escapes the detector undetected, leading to a signature of missing transverse energy (
). The remaining signal characteristics are determined by the nature of
. In this paper, quantities such as missing transverse energy and transverse momentum are given in vector notation when their direction matters (
and
) are in roman font when their magnitudes are used (
and
).
ATLAS examined the case where is a photon (ATLAS Collaboration, Citation2015a) by selecting events with a photon in the central region of the detector defined by
1.37, with high transverse momentum
GeV and high missing transverse energy
150 GeV. The photon is required to be away from the missing energy direction in the transverse plane
. At most one hadronic jet is permitted in these events, while events with electrons above 7 GeV or muons above 6 GeV of transverse momentum are vetoed. The absence of excess in the signal region is used to derive upper limits on the production of dark matter particles together with a final state photon. The experimental result is interpreted in the framework of an effective field theory (EFT); the complete list of allowed effective operators is given in Goodman et al. (Citation2010). Here, the case where the WIMP is a Dirac particle is investigated. The EFT operators that can be constrained by this analysis correspond to a spin-1 mediator with a vector coupling (D5), an axial-vector coupling (D8) and tensor interaction (D9). The other parameters of the EFT are the so-called suppression scale
and the mass of the WIMP
. The effective theory is only valid if the momentum transfer in the elementary process
is smaller than the mass of the particle mediating the interaction
. In the EFT,
is integrated away and is not an explicit parameter of the theory. If the coupling of the mediator particle to the standard model fermions is written
while
is its coupling to the WIMP, then the EFT validity condition is written
. In order to evaluate the effect of this condition on the ATLAS limits on dark matter, one can re-evaluate the limits on dark matter using the conservative assumption that ATLAS simply has no sensitivity to dark matter events in which the EFT validity condition is broken, the
distribution is truncated to remove dark matter signal events above the validity limit. The fraction of dark matter signal events that are truncated depends on the value of
, thus two cases are considered:
and
, also referred to as max coupling. Figure shows the ATLAS limits based on the single-photon signal region, without truncation and with the two truncation scenarios for the EFT operators D5, D8 and D9. Limits on
up to 760 GeV (D5, D8) and up to 1 TeV (D9) are obtained. The effect of the truncation is small in the case of D9 but can be strong in the case of max coupling for operators D5 and D8. Upper limits on the WIMP–nucleon spin-dependent and spin-independent cross-sections are inferred and also shown in Figure .
Figure 1. ATLAS limits on dark matter production with a high transverse momentum photon in the final state, in the framework of the EFT for operators D5 (top left), D8 (top right) and D9 (bottom left). Bottom right: Upper limits on the WIMP–nucleon cross-section in the spin-dependent and spin-independent cases inferred from the high transverse momentum photon results.
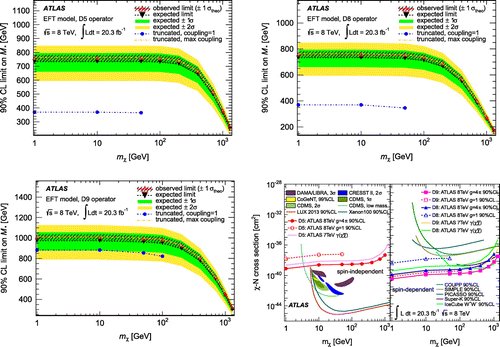
The absence of excess in ATLAS data in the high transverse momentum photon signal region is also interpreted in the framework of a simplified model where the WIMP production at LHC is mediated by a heavy spin-1 vector boson. The model parameters are the WIMP mass , the mediator mass
, the mediator width
and the coupling
. Figure shows the upper limit on
in the case
. The shaded area in the upper left corner corresponds to models that would provide relic dark matter densities larger than those allowed by cosmological measurements and is thus excluded. The ATLAS’s high transverse momentum photon signal region is finally used to set limits on a model where dark matter is produced at LHC via an effective theory
vertex (Nelson, Carpenter, Cotta, Johnstone, & Whiteson, Citation2014). The model parameters are the coupling strength of the WIMP to the
and
gauge bosons,
and
, and the suppression mass scale
. Figure shows the lower limits on the suppression scale
in this scenario. The shaded area corresponds to models where the relic dark matter density provided by the model would be inconsistent with other experimental observations considered in Nelson et al. (Citation2014).
Figure 2. Left: ATLAS limits on the coupling in a simplified model where dark matter production at LHC is mediated by a vector boson of mass
. Right: Upper limits on
when the high transverse momentum photon signal region is used to set limits on an effective theory with a
vertex.
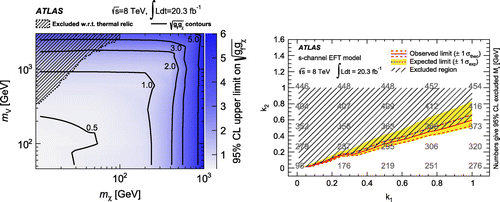
In the case of boosted and hadronically decaying (ATLAS Collaboration, Citation2014a), the two daughter quarks are boosted and yield a large cone jet. The large jet is reconstructed using the Cambridge–Aachen algorithm (Dokshitzer, Leder, Moretti, & Webber, Citation1997) of size
, with transverse momentum
GeV and
1.2. It is required that two anti-
jets of size
are also found inside the large cone jet and that the transverse momentum is distributed between them as expected from
decays, this is ensured by
, where
is the invariant mass of the two small jets, and
and
are their momenta and
is the inter-jet distance. Finally, for consistency with a
decay,
GeV is required. A veto is applied against leptons, photons and light jets. Two signal regions are defined with
350 and 500 GeV.
In the case of leptonically decaying (ATLAS Collaboration, Citation2014b), a single electron (muon) with
GeV (45 GeV) is required. The same lepton-dependent selection cut value is applied on the
. The final discriminating variable is the transverse mass
, where
is the distance in the azimuthal angle
between the charged lepton and the direction of
. Several signal regions are used with different thresholds on
, but start to be sensitive to new physics at
GeV.
If the associated boson is a decaying into two charged leptons (ATLAS Collaboration, Citation2014c), the identification of the final state relies on the presence of two same flavour leptons denoted
(electrons or muons), with
GeV and an invariant mass within 10 GeV of the
-boson mass, and
of the dilepton system less than 2.5. The dilepton system is required to balance
in both direction and magnitude, with
, where
is the transverse momentum of the dilepton system and
. The final discriminating variable defining the signal regions is the
which is required to be above a lower threshold of 150, 250, 350 and 450 GeV, thus defining four regions.
The results from ATLAS dark matter searches are translated into upper limits on the WIMP–nucleon cross-section as function of the WIMP mass () in Figure using an EFT approach (Goodman et al., Citation2010), in the case of spin-independent (left panel) and spin-dependent interactions (right panel).
2. Dark matter with heavy flavour
In a number of models, a single top quark can be produced in association with missing energy arising from the presence of a single stable or metastable particle that could be interpreted as a dark matter candidate or “top+dark matter”. ATLAS considered two scenarios (ATLAS Collaboration, Citation2015b) one in which the production is resonant via a coloured scalar resonance which decays into a top quark and a spin-1/2 colour singlet fermion
. In the second scenario, the production considered is non-resonant and leads to the production of a colour singlet spin-1 boson labelled
. Two signal regions are designed for the two production modes. Both signal regions rely on the semi-leptonic decay of the top quark, thus requiring one isolated lepton
(electron or muon) with
GeV; the presence of exactly one b-tagged jet
with
GeV is also required together with
35 GeV. To reduce the background from
+jets and multijet processes, it is also required that
60 GeV. Signal Region I is targeted at resonant production and requires
210 GeV and
1.2, while Signal Region II requires
250 GeV and
1.4. The dominant background in these signal regions is single top and top pair production. The ATLAS data are observed to agree well with the background-only hypothesis, and are thus used to set limits on the considered models. Figure shows the upper limits on the visible production cross-sections in the resonant and non-resonant scenarios as function of the stable invisible particle masses
and
. In the resonant case, effective coupling strengths
0.15 are excluded for
between 0 and 100 GeV. In the non-resonant scenario, effective couplings above
0.1 (0.2 and 0.3) are excluded for
up to 432 GeV (657 and 796 GeV, respectively).
Figure 4. Left: ATLAS upper limits on the visible production cross-section in the resonant production mode for top+dark matter for different values of the resonant effective coupling strength . Right: ATLAS upper limits on the visible production cross-section in the non-resonant production mode for top+dark matter, for different values of the non-resonant effective coupling strength
.
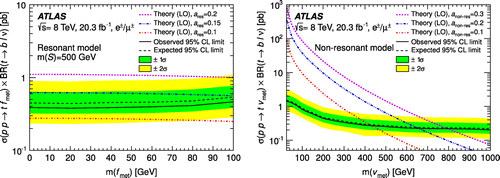
Associated production of a pair of WIMPs together with a single b-quark or a pair of top or b-quarks is possible via EFT operators D1, C1 and D9 (Lin, Kolb, & Wang, Citation2013). The coupling strength in the case of the D1 and C1 operators is proportional to the mass of the quark at the vertex and is thus heavily suppressed for light quarks. Final states with heavy quarks are therefore an important probe of the D1 and C1 operators. ATLAS developed four signal regions (ATLAS Collaboration, Citation2015c) to address this scenario. The four signal regions labelled SR1 to SR4 are designed to cover the various scenarios with either b-quarks or top-quarks, final states with up to 2 jets or more than 3 jets, and cases where both top quarks decay hadronically versus the case where one of the top quarks decays semileptonically. The ATLAS data agree well with the background-only hypothesis in all four signal regions. For the final dark matter limits, the signal region providing the best expected sensitivity is selected and depends on the EFT operator considered. As in the case of the single high transverse momentum photon signal region, the limit of validity of the EFT is considered; here, the maximum coupling scenario is adopted. Figure shows the upper limit on the spin-independent and spin-dependent WIMP–nucleon cross-sections as function of the WIMP mass .
Figure 5. ATLAS upper limits on the WIMP–nucleon cross-sections in the spin-independent case via the D1 EFT operator (left) and in the spin-dependent case via the D9 EFT operator (right), as function of the WIMP mass .
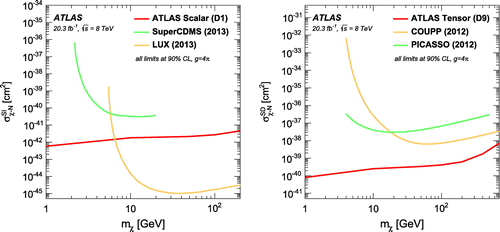
ATLAS also considered the model proposed to explain excess of -rays from the galactic centre, recently observed by Fermi satellite, and interpreted as a signal for DM annihilation in Agrawal, Batell, Hooper and Lin (Citation2014). In this model dark matter has bottom flavour and could be produced at LHC in association with b-quarks via a new scalar field
. For
= 35 GeV as suggested by the FERMI signal at the galatic center, mediator masses between 300 and 500 GeV are excluded at 95% CL.
Acknowledgements
The author would like to acknowledge support from the Swedish Research Council and Stockholm University Natural Science Faculty.
Additional information
Funding
Notes on contributors
Christophe Marcel Victor Clément
The author is involved in the analysis of data from the ATLAS experiment since 2010, and in particular in the search for Supersymmetry, weakly produced supersymmetric particles and search for dark matter using the so-called monojet final states. The work presented here is a summary of recent searches performed by the ATLAS Collaboration, relevant to the search for dark matter using the so-called monojet, mono-photon, mono-W/Z and mono-top final states.
Notes
This article is associated with the From Higgs to Dark Matter 2014 meeting in Geilo, Norway.
On behalf of the ATLAS Collaboration.
References
- Agrawal, P., Batell, B., Hooper, D., & Lin, T. (2014). Flavored dark matter and the galactic center gamma-ray excess. Physical Review D, 90, 063512.
- ATLAS Collaboration. (2014a). Search for dark matter in events with a hadronically decaying W or Z boson and missing transverse momentum in pp collisions at √s = 8 TeV with the ATLAS detector. Physical Review Letters, 112, 041802.
- ATLAS Collaboration. (2014b). Search for new particles in events with one lepton and missing transverse momentum in pp collisions at √s = 8 TeV with the ATLAS detector. Journal of High Energy Physics, 09, 037.
- ATLAS Collaboration. (2014c). Search for dark matter in events with a Z boson and missing transverse momentum in pp collisions at √s = 8 TeV with the ATLAS detector. Physical Review D, 90, 012004.
- ATLAS Collaboration. (2015a). Search for new phenomena in events with a photon and missing transverse momentum in pp collisions at √s = 8 TeV with the ATLAS detector. Physical Review D, 91, 012008.
- ATLAS Collaboration. (2015b). Search for invisible particles produced in association with single-top-quarks in proton--proton collisions at √s = 8 TeV with the ATLAS detector. European Physical Journal C, 75, 79. Retrieved from http://link.springer.com/article/10.1140/epjc/s10052-014-3233-4
- ATLAS Collaboration. (2015c). Search for dark matter in events with heavy quarks and missing transverse momentum in pp collisions with the ATLAS detector. European Physical Journal C, 75, 92, Retrieved from http://link.springer.com/article/10.1140/epjc/s10052-015-3306-z
- Dokshitzer, Y. L., Leder, G. D., Moretti, S., & Webber, B. R. (1997). Better jet clustering algorithms. Journal of High Energy Physics, 08, 001.
- Goodman, J., Ibe, M., Rajaraman, A., Shepherd, W., Tait, T. M. P., & Yu, H.-B. (2010). Constraints on dark matter from Colliders. Physical Review D, 82, 116010.
- Lin, T., Kolb, E. W., & Wang, L. T. (2013). Probing dark matter couplings to top and bottom at the LHC. Physical Review D, 88(6), 063510.
- Nelson, A., Carpenter, L. M., Cotta, R., Johnstone, J. A., & Whiteson, D. (2014). Confronting the Fermi line with LHC data: An effective theory of dark matter interaction with photons. Physical Review D, 89, 056011.