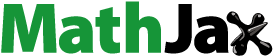
Abstract
The rate-independent stabilization of magnetic states with iterations in a Heusler alloy has been studied. The direct measurement of the adiabatic temperature change, ΔTad, of a Ni51Mn33.4In15.6 alloy near the magnetostructural phase transition is presented. The adiabatic temperature change at a given temperature within the temperature range of the magnetostructural transition is history dependent and varies considerably with the iteration count of the field cycle. The data show the transition from the low magnetization state to the high magnetization state during low to high (L–H) temperature change direction and from high magnetization to low magnetization state during high to low (H–L) temperature change direction require several field cycles to stabilize the ΔTad measurement, similar to the accommodation phenomenon in hysteretic materials. In the mixed magnetic state inside the first-order transition, both low and high magnetization portions of the alloy exist and it varies considerably with the induced fields. This original observation emphasizes that it is incorrect to assess the performance of a magnetic refrigeration system through a single measurement, and that achieving a stable, utilizable, adiabatic temperature change requires several field-induced transitions.
Public Interest Statement
In the advancement of alternative energy sources, magnetocaloric effect shows a promising technology for development of compact and energy efficient magnetic refrigerators. In the past 20 years, there has been a surge in research on the magnetocaloric response of materials, due mainly to the possibility of applying this effect for magnetic refrigeration close to room temperature. However, the magnetic materials available and studied by the scientific community do not yet have the necessary characteristics to be used in large scale, due to technological and/or economic restrictions. The purpose of this paper is to investigate the history-dependent behavior of the spin-reorientations and its effect on the directly measured adiabatic temperature change (ΔTad) in a Ni51Mn33.4In15.6 Heusler alloy.
1. Introduction
Heusler alloys have been studied in recent years due to their wide useful applications such as shape memory alloys (Krumme et al., Citation2015). Ni-Mn-based Heusler alloys have been the subjects of many studies because of their unusual and complex magnetic properties as well as their potential use as magnetocaloric devices (Du et al., Citation2007; Hütten et al., Citation2006; Krenke et al., Citation2007; Seyoum et al., Citation2013; Sharma, Chattopadhyay, & Roy, Citation2007). They are known to exhibit mixed magnetic states during the magnetostructural transformation resulting in a disorder and competing interactions between different magnetic sublattices (Aksoy, Acet, Deen, Mañosa, & Planes, Citation2009; Bennett et al., Citation2012; Ye et al., Citation2010). Spin reorientation mainly affects the orientation of the spins, while the magnetization magnitude remains unchanged. Such weak-order changes could be observed by few techniques and methods: neutron diffraction, X-ray diffraction, and AC resistometry (Gotoh, Ohashi, Kanomata, & Yamaguchi, Citation1995; Khovailo, Abe, & Takagi, Citation2003; Skanthakumar, Zhang, Clinton, Li, & Lynn, Citation1989).
Alternatively, additional peaks and anomalies in the direct measurements of the adiabatic temperature change reflect the existence of spin reorientations and the temperature range where they happen. Adiabatic temperature change is defined as the heating or cooling of magnetic material due to varying magnetic field under adiabatic conditions. Previous studies of spin-reorientations focused mainly on driving the change through controlling the alloy composition or the way it was prepared (Han et al., Citation2007; Krenke et al., Citation2006). The purpose of this paper is to investigate the history-dependent behavior of the spin reorientations and its effect on the directly measured adiabatic temperature change (ΔTad) in a Ni51Mn33.4In15.6 Heusler alloy.
2. Measurements and discussion
An ingot with a nominal composition of Ni51Mn33.4In15.6 was prepared by induction melting pure metals in an argon atmosphere. A rectangular prism specimen, with dimensions 25 mm × 11 mm × 10 mm, was cut from the ingot. The specimen was heat treated in an argon atmosphere at 900°C for 8 h, aged at 500°C for 48 h, and then quenched in water. A photography of the sample is shown in Figure .
Figure 1. Photography of the Huesler alloy Ni51Mn33.4In15.6 sample.
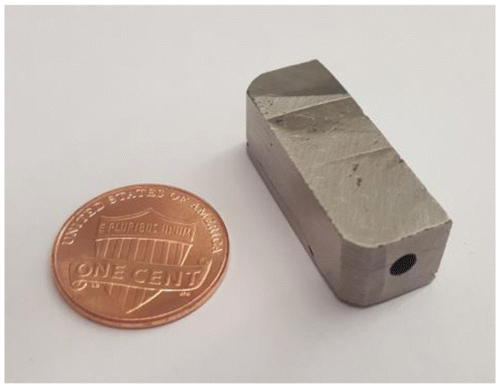
A photo of the experimental apparatus is shown in Figure . The experimental setup, its accuracy, and more details about the measurement procedure can be found in Ghahremani, Jin, et al. (Citation2012), Ghahremani, Seyoum, ElBidweihy, Della Torre, and Bennett (Citation2012). The magnetization, M, vs. the temperature, T, measurements of Ni51Mn33.4In15.6 under a field as low as 100 Oe showed that the temperature range for the magnetostructural transition is 280–310 K and 290–310 K for the L–H and H–L processes, respectively (Seyoum et al., Citation2013).
Ghahremani et al. (Citation2013) studied the latent heat of phase transmission in Heusler alloy. They measured an independent adiabatic temperature change (ΔTad) of Ni51Mn33.4In15.6 during the magnetization for L–H and H–L temperature change direction processes for a 2 T applied magnetic field as shown in Figure . The measurement procedure that was followed in Ghahremani et al. (Citation2013) work was done through the following process:
(1) | The sample temperature is set to an initial temperature that is 281 K for L–H process and 305 K for H–L process. | ||||
(2) | The magnetic field of 2 T is applied to the sample. | ||||
(3) | ΔTad is then measured and recorded. | ||||
(4) | The magnetic field is removed. The system is allowed to equilibrate. A waiting time of 20 min between two successive temperature measurements is used. A waiting time up to 130 min shows no additional differences and thus guarantees that complete equilibrium had been reached and displays the independency of each data point. | ||||
(5) | The sample’s initial temperature is increased or decreased by 0.5 K for L–H or H–L processes, respectively. | ||||
(6) | Repeat Steps (2–5) until reaching up to 305 K for the L–H process and 281 K for the H–L process. |
Figure 3. The adiabatic temperature change, ΔTad, vs. the temperature, T, of Ni51Mn33.4In15.6 measured during the magnetization process with L–H and H–L temperature change directions for a magnetic field change of 2 T. The dashed line is at 292 K, where the measurement was rate-independently repeated.
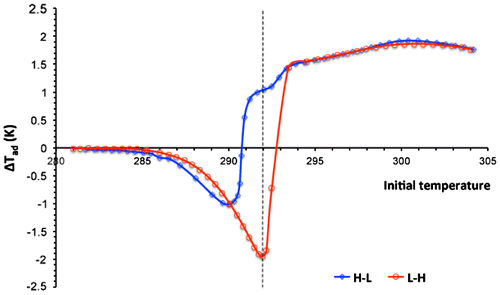
This experimental procedure guarantees that each data point depends only on whether the system is transforming from ferrimagnetic to ferromagnetic to paramagnetic or vice versa. Otherwise stated, each data point depends on whether the temperature change direction is H–L or L–H. Each ΔTad measurement is recorded only upon the application of the magnetic field within a magnetization process (Step 3). As all points were measured independently, the measurement procedure cancels any contribution of magnetic hysteresis. Therefore, the hysteresis shown in Figure is thermal hysteresis only.
The measurements in Figure display the conventional and inverse magnetocaloric effect peaks within the temperature range for the magnetostructural transition. For the same alloy and under different magnetic field changes, additional peaks and recurring kinks in the 290–295 K temperature range were observed (Seyoum et al., Citation2013). Thus, this paper describes an investigation of the behavior of spin reorientation at 292 K, a temperature lying within that temperature range.
As seen in Figure , at temperature 292 K (marked by dashed line), there exist two different ΔTad for L–H and H–L processes under magnetization of 2 T magnetic field. It is worth mentioning that the ΔTad at 292 K for L–H process is negative and for H–L process is positive. In order to study the history-dependent behavior of the sample in the magnetostructural transition region, the direct measurement of ΔTad for magnetization was reiterated a sufficient number of times at 292 K for both L–H and H–L processes to obtain repeatable results. The experimental procedure is as follows:
(1) | The sample temperature is set to an initial temperature of 292 K. | ||||
(2) | The sample is then magnetized by a magnetic field of 2 T. | ||||
(3) | ΔTad is then measured and recorded. | ||||
(4) | The magnetic field is removed. The system is allowed to equilibrate. | ||||
(5) | For L–H process, the sample is cooled down to 280 K, and then the sample’s temperature is increased to 292 K. For H–L process, the sample is heated up to 305 K, and then decreased again to 292 K. (This step guarantees independent measurements, and removes the existence of any magnetic hysteresis.) | ||||
(6) | Repeat Steps (2–5) until a stable measurement is recorded. |
The magnetization adiabatic temperature change (ΔTad) vs. the number of iterations for the L–H and H–L temperature change directions is shown in Figures and , respectively. For the L–H process, the measured magnetization ΔTad at 292 K for the first iteration is −2 K and it eventually stabilizes at −1.57 K. For the H–L process, the measured magnetization ΔTad at 292 K for the first iteration is 0.96 K and it eventually stabilizes at 0.86 K. It was found that, for this sample, nine iterations were sufficient to obtain a repeatable ΔTad measurement at 292 K.
Figure 4. The adiabatic temperature change, ΔTad, vs. the iteration count measured during the L–H magnetization process for a magnetic field change of 2 T.
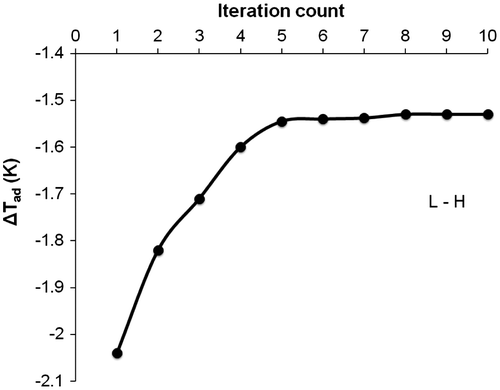
Figure 5. The adiabatic temperature change, ΔTad, vs. the iteration count measured during the H–L magnetization process for a magnetic field change of 2 T.
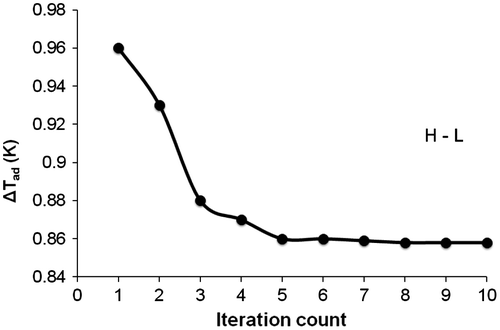
The effect shown in Figures and is similar to the accommodation phenomenon in hysteretic materials (Bennett, Vajda, Atzmony, & Swartzendruber, Citation1996; Della Torre, Citation1994; Della Torre & Jin, Citation2009). Accommodation (reptation) is the cyclical change of minor hysteresis loops towards an equilibrium limit cycle. The effect exhibited by the ΔTad measurements in this paper is rate independent, similar to accommodation. The stabilization occurs because the spin reorientation between the mixed magnetic states within the magnetostructural transition region is asymmetrical and requires several field-induced transitions to be repeatable. Unlike the magnitude of the magnetization, the adiabatic temperature change is sensitive to the orientations of the spins. Depending on the strength of the field change, spins will reorient to a new favorable magnetization axis. When the field is removed, not all the spins will revert back to the original orientation. After several field cycles, all the retained spins contribute to the reorientation process and the effect is now deterministic leading to a stable measurement. It is concluded that ΔTad will display a rate-independent transient-like behavior for the first few measurements before a consistent, stable measurement is obtained.
This stabilization effect, revealed for the first time in this paper, contributes to a significant difference in calculating the coefficient of performance (COP), a coefficient used to measure the performance of magnetic refrigeration systems. COP is equal to the ratio of heating or cooling provided (Q) to the energy dissipated (W). The amount of heat generated in each magnetization cycle is given by:(1)
(1)
where m is the mass of the sample and cp is the specific heat capacity at a given temperature. The relationship between the COP and the adiabatic temperature change is, therefore, given by:(2)
(2)
As seen in Figures and , the calculated COP at 292 K of the 1st iteration is 27% larger than that of the 9th iteration for the L–H process, and 12% larger for the H–L process. Considering that researchers usually record ΔTad after the first iteration, and that magnetic refrigeration is a cyclical process, our findings emphasize the error involved in measuring ΔTad or calculating COP after only one field iteration/cycle. This error leads to an overestimate of the performance of a magnetic refrigeration system.
3. Conclusion
Cyclical rate-independent measurements of the adiabatic temperature change, ΔTad, of Ni51Mn33.4In15.6 revealed that spin reorientations within the temperature range of the magnetostructural transition (292 K) are asymmetrical, vary with the iteration count, and change step by step into an equilibrium limit cycle. These weak-order changes require several field cycles to drive the measured ΔTad into a stable measurement, similar to the accommodated minor loops of hysteretic materials. The measurements presented emphasize the overestimate of the performance of a magnetic refrigeration system using a single cycle of ΔTad direct measurement.
Corrigendum
This article was originally published with errors. This version has been corrected. Please see Corrigendum (http://dx.doi.org/10.1080/23311940.2015.1130205).
Acknowledgments
We would like to thank Dr. Francis Johnson and Dr. Min Zou from G.E. Global Research for supplying the test sample and for useful discussions.
Additional information
Funding
Notes on contributors
Mohammadreza Ghahremani
Mohammadreza Ghahremani received his MSc degree in Computer Engineering from Sharif University of Technology in 1999, and PhD degree in Computer Engineering from the George Washington University in 2014. As a postdoctoral Scientist at the Institute for Magnetics Research (IMR) at the George Washington University, he conducted research in the area of magnetic refrigeration systems and magnetocaloric materials in collaboration with G.E. Global Research. His research objectives include design, build, and testing an advanced magnetocaloric temperature change test bed and magnetic refrigeration cooling system.
He is currently an assistant professor in the Department of Computer Science, Mathematics, and Engineering at the Shepherd University, where his research fields are magnetic refrigeration and energy efficient cooling systems.
References
- Aksoy, S., Acet, M., Deen, P. P., Mañosa, L., & Planes, A. (2009). Magnetic correlations in martensitic Ni-Mn-based Heusler shape-memory alloys: Neutron polarization analysis. Physical Review B, 79, 212401. doi:10.1103/PhysRevB.79.212401
- Bennett, L. H., Provenzano, V., Shull, R. D., Levin, I., Della Torre, E., & Jin, Y. (2012). Ferri-to ferro-magnetic transition in the martensitic phase of a Heusler alloy. Journal of Alloys and Compounds, 525, 34–38. doi:10.1016/j.jallcom.2012.02.062
- Bennett, L. H., Vajda, F., Atzmony, U., & Swartzendruber, L. J. (1996). Accommodation study of a nanograin iron powder. IEEE Transactions on Magnetics, 32, 4493–4495.10.1109/20.538908
- Della Torre, E. (1994). A Preisach model for accommodation. IEEE Transactions on Magnetics, 30, 2701–2707.10.1109/20.312509
- Della Torre, E., & Jin, Y. (2009). Comparison of the DEMAM and DEAM accommodation models. IEEE Transactions on Magnetics, 45, 1198–1201.10.1109/TMAG.2009.2012557
- Du, J., Zheng, Q., Ren, W. J., Feng, W. J., Liu, X. G., & Zhang, Z. D. (2007). Magnetocaloric effect and magnetic-field-induced shape recovery effect at room temperature in ferromagnetic Heusler alloy Ni-Mn-Sb. Journal of Physics D: Applied Physics, 40, 5523–5526.10.1088/0022-3727/40/18/001
- Ghahremani, M., ElBidweihy, H., Bennett, L. H., Della Torre, E., Zou, M., & Johnson, F. (2013). Implicit measurement of the latent heat in a magnetocaloric NiMnIn Heusler alloy. Journal of Applied Physics, 113, 17A943.
- Ghahremani, M., Jin, Y., Bennett, L. H., Della Torre, E., ElBidweihy, H., & Gu, S. (2012). Design and instrumentation of an advanced magnetocaloric direct temperature measurement system. IEEE Transactions on Magnetics, 48, 3999–4002. doi:10.1109/TMAG.2012.2203108
- Ghahremani, M., Seyoum, H. M., ElBidweihy, H., Della Torre, E., & Bennett, L. H. (2012). Adiabatic magnetocaloric temperature change in polycrystalline gadolinium—A new approach highlighting reversibility. AIP Advances, 2, 032149. doi:10.1063/1.4748131
- Gotoh, M., Ohashi, M., Kanomata, T., & Yamaguchi, Y. (1995). Spin reorientation in the new Heusler alloys Ru2MnSb and Ru2MnGe. Physica B: Condensed Matter, 213–214, 306–308.10.1016/0921-4526(95)00138-Y
- Han, Z. D., Wang, D. H., Zhang, C. L., Xuan, H. C., Gu, B. X., & Du, Y. W. (2007). Low-field inverse magnetocaloric effect in Ni50−xMn39+xSn11 Heusler alloys. Applied Physics Letters, 90, 042507.10.1063/1.2435593
- Hütten, A., Schmalhorst, J., Thomas, A., Kämmerer, S., Sacher, M., Ebke, D., … Reiss, G. (2006). Spin-electronic devices with half-metallic Heusler alloys. Journal of Alloys and Compounds, 423, 148–152.10.1016/j.jallcom.2005.12.106
- Khovailo, V. V., Abe, T., & Takagi, T. (2003). Detection of weak-order phase transitions in ferromagnets by ac resistometry. Journal of Applied Physics, 94, 2491–2493.10.1063/1.1594840
- Krenke, T., Acet, M., Wassermann, E. F., Moya, X., Mañosa, L., & Planes, A. (2006). Ferromagnetism in the austenitic and martensitic states of Ni-Mn-In alloys. Physical Review B, 73, 174413. doi:10.1103/PhysRevB.73.174413
- Krenke, T., Duman, E., Acet, M., Wassermann, E. F., Moya, X., Mañosa, L., … Ouladdiaf, B. (2007). Magnetic superelasticity and inverse magnetocaloric effect in Ni-Mn-In. Physical Review B, 75, 104414.10.1103/PhysRevB.75.104414
- Krumme, B., Auge, A., Herper, H., Opahle, I., Klar, D., Teichert, N., … Wende, H. (2015). Element-specific electronic structure and magnetic properties of an epitaxial Ni51.6Mn32.9Sn15.5 thin film at the austenite-martensite transition. Physical Review B, 91, 214417.10.1103/PhysRevB.91.214417
- Seyoum, H. M., Ghahremani, M., ElBidweihy, H., Bennett, L. H., Della Torre, E., Johnson, F., & Zuo, M. (2013). Metastability in the magnetic structure of Ni51Mn33.4In15.6 Heusler alloy. IEEE Magnetics Letters, 4, 6000204.
- Sharma, V. K., Chattopadhyay, M. K., & Roy, S. B. (2007). Large inverse magnetocaloric effect in Ni50Mn34In16. Journal of Physics D: Applied Physics, 40, 1869–1873.10.1088/0022-3727/40/7/005
- Skanthakumar, S., Zhang, H., Clinton, T. W., Li, W.-H., & Lynn, J. W. (1989). Magnetic phase transitions and structural distortion in Nd2CuO4. Physica C: Superconductivity, 160, 124–128.10.1016/0921-4534(89)90180-9
- Ye, M., Kimura, A., Miura, Y., Shirai, M., Cui, Y. T., Shimada, K., … Kanomata, T. (2010). Role of electronic structure in the martensitic phase transition of Ni2Mn1+xSn1−x studied by hard-X-ray photoelectron spectroscopy and ab initio calculation. Physical Review Letters, 104, 176401. doi:10.1103/PhysRevLett.104.176401